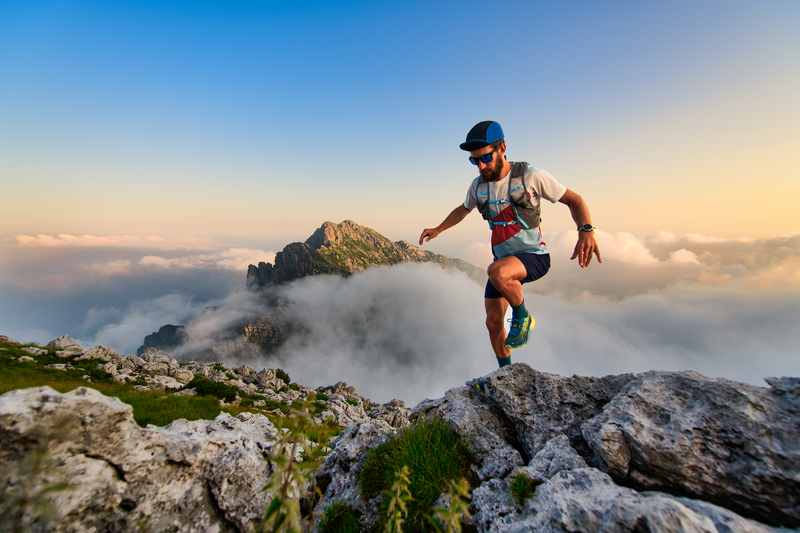
95% of researchers rate our articles as excellent or good
Learn more about the work of our research integrity team to safeguard the quality of each article we publish.
Find out more
REVIEW article
Front. Energy Res. , 30 March 2022
Sec. Fuel Cells, Electrolyzers and Membrane Reactors
Volume 10 - 2022 | https://doi.org/10.3389/fenrg.2022.844729
This article is part of the Research Topic Research in (PEM) Fuel Cell and Fuel Cell Catalysis View all 6 articles
In the commercialization of the hydrogen fuel cell for the transportation sector, one of the main factors affecting the lifespan of the fuel cell is voltage reversal, especially when the anode of the fuel cell is subject to fuel starvation momentarily during the operation. In this article, mitigation methods for voltage reversal are summarized in three parts, namely, the catalyst approaches, the MEA design approaches, the stack and system strategies approaches, which include the application of a highly active oxygen evolution reaction (OER) catalyst or durable catalyst support in the anode, employing a protective layer for the catalyst layer or optimizing the formula of the catalyst layer or employing a durable GDL, or optimization of stack design or system operation strategies.
In an effort to decarbonize the transportation sector, there has been a solid desire to electrify the drivetrain of both commercial and passenger vehicles. The approaches include hybrid vehicles, lithium battery vehicles, and hydrogen fuel cell vehicles. Specifically, for commercial vehicles such as buses and trucks, the fast refueling and long-distance driving range can be achieved by hydrogen fuel cells. Additionally, hydrogen fuel cells exhibit high energy density, high energy conversion efficiency, and zero on-site pollution (Peng et al., 2015; Xiong et al., 2015; Jia et al., 2017; Yanzhou, 2017; Bizon et al., 2019; Huang et al., 2020). Hyundai, Toyota, Honda, and other car companies have launched various fuel cell vehicles, and in China, this technology is being used to replace diesel engines. However, fuel cells are yet to achieve significant cost reduction to expand their commercial footprint. To lower the cost of fuel cell stacks, approaches such as utilizing non-Pt catalysts to replace the Pt/C (Peng et al., 2013; Liu et al., 2014a; You et al., 2014; Nakashima, 2019; Sibul et al., 2020) and self-humidifying membrane electrode assembly (MEA) to reduce the size/cost of the humidifier (Fan et al., 2017; Anu and Cindrella, 2019; Hou, 2019; Shin, 2020) have been investigated. To improve the performance of fuel cells, significant research has been focusing on the synthesis of catalysts (particularly the cathode) (Paul et al., 2015; Dang et al., 2017; Dustin et al., 2019; Chalgin, 2020) and extensive efforts have been carried out to understand the mechanism of degradation (Liu et al., 2014b; Jia et al., 2016; Shen, 2020; Vichard et al., 2020; Chen et al., 2021a; Hu, 2021; Rui, 2021).
During normal fuel cell operations, electrode potential drives the desired electrochemical reactions, namely, the oxygen reduction reaction (ORR) at the cathode and the hydrogen oxidation reaction (HOR) at the anode. Under normal circumstances, both the anode and the cathode have excess hydrogen and air supply, respectively. However, there are circumstances that cause drastic potential excursions at the electrode, such as low catalyst performance, insufficient supply of reactants, uneven gas distribution, rapid changes in load, and startup or shutdown operations. These failure modes are known to degrade the performance of MEA rapidly, and hence degrade the performance of the fuel cell stack as a whole (Chen et al., 2019; Wh, 2021; Jia et al., 2015; Cheng, 2015; Zhao, 2020).
For a commercial application, one of the most damaging factors is the global fuel starvation. This occurs when complete blockage of H2 occurs at the anode of one or more MEAs, while the other MEA in the stack continues to operate normally. As shown in Figure 1A, local fuel starvation leads to current reversal, while overall fuel starvation leads to voltage reversal as shown in Figure 1B. A slight fuel shortage occurs during low stoichiometric ratio or a surge in load demand, and these may resulting in local fuel starvation. Low pressure caused by local fuel starvation would result in the permeation of air; accordingly, carbon corrosion takes place in regions facing air/H2 boundary and in the cathode. When the stoichiometric ratio of fuel is less than 1, overall fuel starvation occurs. Once the fuel cell has been forced to sustain the current, carbon oxidation reaction (COR) and OER would take place to provide protons and electrons. When this happens, as is shown in Figure 2, the anode potential of the fuel-starved MEA is driven to excessively high potentials (>2 V), leading to the rapid oxidation of the anodic carbon structure. Meanwhile, the cell voltage drops rapidly until below 0 V, and the fuel cell turns into an electrolyzer. This failure must be differentiated from local fuel starvation, which leads to a very different failure mode in which the cathodic carbon structure would be damaged. At the same time, the anode does not experience any high potential.
FIGURE 2. Potential profile of the single cell during the simulated cell reversal event. Reproduced with permission (Taniguchi et al., 2004). Copyright 2004, Elsevier.
When hydrogen is not supplied to the anode, the voltage of the MEA decreases rapidly in a few seconds due to the raising of the anode potential. The reversal occurs when the anode potential exceeds the cathode potential. When the anode potential rises to 1.5 V, both carbon corrosion and water electrolysis occur to provide electrons and protons, rather than the normal hydrogen oxidation reaction to provide protons and electrons (Zhang et al., 2012; Yi, 2012; Dong, 2009). The result of these reversal events is carbon corrosion and sintering of Pt particles leading to significant damage to the anode catalyst layer and eventually complete failure of the MEA. Carbon corrosion of the anode near the outlet of the stack is often more severe than that of the inlet due to H2 concentration gradients through the flow fields (Yi, 2012). As shown in Figure 3, the voltage of the two ends of singles cells have been compared under different fuel stoichiometry. Compared with single cells in Figure 3A, and the single cells in Figure 3B that is partially fuel starved is found to quickly suffer from voltage drop (Hu et al., 2018). Water electrolysis and carbon corrosion are concurrent. However, the anode potential determines the relative rate of the two reactions. Water electrolysis is favored at cell voltages between −0.9 V and −1.1 V (Velikokhatnyi et al., 2013; Liu et al., 2014b; Wang et al., 2020a; Wang et al., 2020b), while carbon corrosion is favored between −1.7 and 2.0 V (Hasché et al., 2010; Dhanushkodi et al., 2013; Pandy et al., 2013). Prolonging the reaction of water electrolysis is helpful to alleviate the occurrence of carbon corrosion reaction (Lee et al., 2021a).
FIGURE 3. Voltage difference between the two ends of the cells. Reproduced with permission (Hu et al., 2018). Copyright 2018, Elsevier.
Overcoming reversal has been a key focus within the fuel cell industry. However, it has received relatively less attention within academia. However, with the rapid growth of the PEMFC industry in recent years, and the large-scale deployment of the technology, the importance of solving this critical issue has gained momentum in the academic research community. Lots of work on reversal tolerance anode (RTA) strategy were explored; to reduce the influence of fuel starvation, RTA uses OER catalyst, which promotes the electrolysis of water and reduces the rate of COR. In this review article, approaches to solving this technical challenge are categorized and discussed according to the 1) materials level (catalyst solutions), 2) MEA level (anode/MEA design solutions), and 3) stack and system level. Following a review of recent literature, concluding remarks on future directions are made.
Previous studies suggested that COR and MEA deterioration can be suppressed by adding OER catalyst into the anode. Using oxidation-resistant carbon supports is also helpful in alleviating carbon corrosion (Petch et al., 2015). Thus, from the level of the material, approaches to overcoming reversal have focused on developing a highly active OER catalyst and a highly durable HOR catalyst.
Tita Labi (2021) prepared graphitized Vulcan carbon black-supported IrOx catalyst (IrOx/GV) by the microwave-assisted polyol deposition technology. The mass fraction of Ir in IrOx/GV is 56wt%, and the Ir nanoparticles are 4–8 nm. IrOx/GV was added to the anode CL as an OER catalyst, and the loading of Ir in the CL was 0.1 mg/cm2. After 5 min, 10 min, and 30 min of the intermittent reversal test, the reversal voltage is basically maintained at −0.8 V; after the reversal test, the performance is kept at 95% of the initial performance of the fuel cell, without causing serious performance damage. Through SEM and EIS analysis, the attenuation of the performance was found to be mainly due to the loss and shrinkage of the proton exchange membrane (PEM) and the structural deformation of the ionomer affecting the proton transport pathway. The anode CL inevitably becomes thinner, which leads to the decline in performance and increase in contact resistance. In addition, it was found that the Pt/C is stable in the short-term intermittent starvation process, while it becomes charged and soluble in the long-term instigation reversal process.
However, due to the difference in the surface characteristics of Pt/C and IrO2, it is still a challenge for IrO2 to be uniformly dispersed in the catalyst slurry. At the same time, the loading of Pt/C and IrO2 catalysts will also be reduced. Compared with directly mixing IrOx prepared using the Adams me1lting method with commercial Pt/C as the reversal tolerance anode, Roh et al. (2019) deposited IrOx on the surface of the commercial Pt/C catalyst. As is shown in Figure 4, compared with the mixture of IrOx and commercial Pt/C, IrOx-Pt/C can protect the carbon support from carbon corrosion, as Figure 4A shows, the durability is increased by four times. The OER ability of different catalyst has been verified by on-line monitoring the release rate of CO2 and O2 as shown in Figures 4B,C. At the same time, the anti-reversal effect of IrOx and Ir were compared, and it was found that the anti-reversal effect of Ir was very poor, mainly because Ir could also participate in the competition of HOR reaction and Ir was easily poisoned by CO.
FIGURE 4. Reversal tolerance ability of various RTAs. Release of (B) CO2 (m/z 44) and (C) O2 (m/z 32) during the voltage reversal test. Reproduced with permission (Roh et al., 2019). Copyright 2019, Elsevier.
Lee et al. (2021b) synthesized graphitized carbon-supported Ir-Ru alloy as an anti-reversal OER catalyst as IrRu2 alloy particles have good particle size distribution. The activity of IrRu2 with grain diameter less than 5.33 nm is 1.1 times higher than the activity of Pt/C. Through the simulated reversal test, using IrRu2/C as an anode catalyst, the durability was actually up to 7 h, while using only a Pt/C catalyst, the durability was only approximately 10 min. The modified IrRu alloy catalyst is expected to be used as an anode catalyst and replace Pt in PEMFC. The surface segregation of Ir in the IrRu alloy would downshift the d-band which lead to the decrease of hydrogen adsorption energy, resulting in high catalytic activity (Lile, 2021). Xiangyang Zhou et al. (Zhou et al., 2020) prepared the IrO2/RuO2 catalyst using the improved Adams melting method. In the half-cell, the durability of the MEA with the IrO2/RuO2 catalyst was improved, the activity decreased slightly, and the voltage of the single cell at 1 A/cm2 and the maximum power density decreased by 2.8 and 8.7%, respectively. The durability was prolonged from 0.67 to 66 min, the H2 starvation test was repeated, and the cumulative durability was as long as 78 h. You et al. (2020) reported the effects of the ratio of Ru to Ir in alloy catalysts and the graphitization extent of the carbon support on the reversal tolerance ability. The impregnation reduction method was used to prepare RuxIry alloy catalysts, and the carbon support was treated at different high temperatures. The results show that the crystalline structure of RuxIry is hexagonal with the increase in Ru content. Under the condition of single-cell test, the performance of RuxIry alloy is similar to that of the Pt/C catalyst. Moreover, the RuxIry alloy catalyst showed a coordinated lifting effect, and the reversal tolerance ability was better than that of the mixed Ir and Ru catalysts in the same proportion. With the same proportion of RuxIry alloy, the higher the graphitization degree of the carbon support, the better the antireversal ability of MEA. Kim et al. (2020) prepared Ir-Ru/C alloy catalysts using the impregnation reduction method. The effects of different reduction temperatures in a hydrogen atmosphere and different heat treatment temperatures in a nitrogen atmosphere on the OER and HOR properties of the Ir-Ru/C alloy catalysts were investigated. The results showed that the precursors of Ir and Ru can also be reduced in a nitrogen atmosphere. The IrRu4/C reduced at 200°C in the hydrogen atmosphere shows better electrochemical activity and stability than IrRu4/C prepared at 300°C, which is related to the low crystallization on the surface of catalyst particles under mild heat treatment. The properties of IrRu4/C at different heat treatment temperatures in the nitrogen atmosphere are the same as those of IrRu4/C prepared in the hydrogen atmosphere. However, the performance of IrRu4/C prepared at 900°C in the nitrogen atmosphere is similar to that of IrRu4/C prepared in the hydrogen atmosphere.
Lee et al. (2019) synthesized graphitized carbon-supported IrRu4Y0.5 ternary alloy catalyst, which showed 21% higher performance and better antireversal durability than Pt/C. It is considered that the IrRuY-based catalyst is expected to displace the Pt-based catalyst as the bifunctional catalyst of HOR and OER. Cullen et al. (2014) reported the OER catalyst would limit the migration of dissolved Pt. This is because Ir tends to exist in the form of highly dispersed nanoparticles, while Pt tends to exist in the form of large particle agglomeration. Ir migrated into the PEM provides nuclei for Pt, which limits further migration of Pt to the PEM. Ru is easier to dissolve than Pt and Ir, but migrates to MPL rather than PEM. Moore et al. (2019) investigated the OER activity and durability of IrO2 with different crystallization and IrOx/TiO2 with TiO2 as support using the non-in situ accelerated pressure test. The results showed that the higher the crystallization of IrO2, the longer the reversal tolerance durability, the higher the specific surface area of IrO2, and the better the mass activity. Compared with IrO2 without support, IrOx/TiO2 has the best mass activity, but the dissolution concentration of Ir is the highest, which may be due to Ti3+ acting as a reducing agent, leading to the dissolution of IrO2, and the formation of oxygen vacancy in TiO2 support is helpful in improving the OER activity of IrOx/TiO2. Tovini et al. (2020) confirmed the surface of IrO2 particles will be reduced to Ir by H2, and the Ir will dissolve, diffuse through the membrane, and be deposited on the cathode Pt/C during repeated start-up and shutdown, resulting in the decrease in the cathode ORR activity. The Ir in the cathode CL can be observed with the help of XPS. At the same time, repeated start and stop will also result in the loss of decreased OER activity because the anode Pt will be dissolved and deposited on the surface of IrO2 to block the OER active site. The chemical stability of IrO2 should be considered when IrO2 is blended with Pt/C as an anode catalyst.
The occurrence of carbon corrosion will not only cause an increase in electrical resistance, but also increase the hydrophilicity of carbon support which leads to flooding (Taniguchi et al., 2004). Some support shows a positive effect on the catalyst, for instance, enhanced active surface area, improved activity, long service life, etc (Puthiyapura et al., 2014; Liu et al., 2020; Bhanja et al., 2019; Min et al., 2017). Kumar et al. (2017) synthesized an anode catalyst with 40wt.% Pt on RuO2-TiO2. Compared with TKK TEC10EA30E, the voltage of Pt/RuO2-TiO2 remained stable after 400 cycles of reversal test, which was related to the corrosion resistance of the support and the excellent OER activity of RuO2. Using Ti4O7 as catalyst support also has a good reversal tolerance ability. TinO2n-1 (4 < n < 10) of Magneli phase has been considered for various electrochemical applications because of its chemical stability and electrical properties. The conductivity of TinO2n-1 increases with the decrease in n, and the conductivity of Ti4O7 is similar to that of graphite. Tsutomu Ioroi et al. (Ioroi and Yasuda, 2020) prepared Ti4O7 by pulsed ultraviolet laser irradiation on TiO2, then impregnated and reduced Pt/Ti4O7 catalyst, and then supported a small amount of Ir on Pt/Ti4O7 by impregnation reduction. The reversal tolerance performance of Pt/C, Pt/GKB, and Pt/Ti4O7 catalysts was compared, and it was found that the reversal tolerance performance of the Pt/Ti4O7 catalyst was the best. At the same time, the reversal tolerance performance of Pt/Ti4O7 with different loading of Ir was compared. The results showed that the reversal tolerance ability improved with the increase of Ir content. For the catalyst supported by trace Ir on Pt/Ti4O7, although the loading of Ir is only 0.014 mg/cm2, the durability is still as long as 7200 s. As is shown in Figure 5, the voltage of Pt-Ir/Ti4O7 at 1 A/cm2 only decreased 19 mV, while using Pt/C as the anode catalyst with the loading of Ir is as high as 0.1 mg/cm2, the voltage decrease is still higher than that of 50 mV. In addition, it is also found that Ir/Ti4O7 with low Pt can replace Pt/Ti4O7 as an anode catalyst, and Ir/Ti4O7 with low Pt has excellent HOR activity and high OER activity. Lee et al. (2020) prepared IrRu4/Ti4O7 with 20% mass fraction of IrRu from commercial Ti4O7 as an anode catalyst. Compared with 20wt.% commercial Pt/C catalyst, the HOR activity of IrRu4/Ti4O7 is similar to that of 20wt.% commercial Pt/C, but the reversal tolerance ability of using IrRu4/Ti4O7 is much higher than that of Pt/C. Zhu et al. (2017) synthesized Pt/C by modifying Pt by hydrophobic ionic liquids. The modified Pt/C catalysts showed excellent oxygen reduction and oxygen evolution performance due to its high ionic conductivity and excellent oxygen solubility of protons ionic liquids. The OER performance of the Pt/C catalyst modified by protons ionic liquids is helpful to protect the carbon group from oxidation and obtain a certain antireversal ability. Jung (2011) used carbon nanotube-supported Pt as a cathode catalyst to study the durability of Pt/CNT under anode reversal. Compared with the application of carbon black, CNT has a smaller edge area and less carbon corrosion when the reversal occurs, which is helpful to alleviate the sintering of Pt particles and boost the durability of MEA. The way to boost the durability of MEA is to increase the loading of anode catalyst, cover the surface of anode catalyst support, or use oxidation-resistant catalyst support. Knights et al. (Knights et al., 2003) believed that increasing the catalyst coverage to greater than 9% of support surface area or decreasing the relative interface perimeter of the catalyst and support to less than 4 × 1010 m/g can help improve the voltage reversal tolerance, and the higher the graphitization extent of support is, the better the voltage reversal tolerance will be.
FIGURE 5. Voltage loss of MEA with various RTAs at 1 A/cm2 after the reversal test for approximately 2 h (H2 and O2 were humidified and the flow is 100 ml/min each, ambient pressure, 80°C). Reproduced with permission (Ioroi and Yasuda, 2020). Copyright 2020, Elsevier.
Wang et al. (2020c) synthesized octahedral PtNi/C which has better electrochemical activity and durability than JM Pt/C. The electrochemical activity of PtNi/C decreased slightly when IrO2, RuO2, and PtNi/C were mixed as a composite anode and the PtNi/C catalyst was used as a cathode. Compared with only PtNi/C as a cathode and anode catalyst, the electrochemical activity of composite anode remained higher after accelerated durability test. Especially under the condition of continuous reversal test, the durability of MEA prepared by the composite anode was extended by 1 h. Hee-sun Kim et al. (Kim et al., 2021) deposited nano-Ir on the Pt/C catalyst and oxidized it at a high temperature to obtain Pt-IrO2/C catalyst. The carbon support is multi-defect carbon support, which is traditionally considered to be more prone to carbon corrosion. IrO2 anchored on the support surface can better protect the support, so that the durability of the fuel cell can be as long as 44 h. Because of the higher specific surface area of multi-defect carbon base, IrO2 has higher OER activity and resistance to CO poisoning. Through the on-line mass spectrometer monitoring, the continuous release of O2 under the condition of reactant starvation could be observed. When the fuel cell begins to fail, the emission of CO2 increases continuously. The treatment temperature of the Pt-IrO2/C catalyst was also studied, and the treatment effects of 250°C, 300°C, 350°C, and 400°C on Pt-IrO2/C were compared. It is not conducive to the formation of IrO2 at a low temperature; however, excessive temperature will cause IrO2 particles to agglomerate and reduce the specific surface area, and the performance of Pt-IrO2/C synthesized at the treatment temperature of 300°C is the best.
The addition of OER catalyst into the anode has two constraints that need to be considered, one challenge is the stability of OER catalyst in acid media, another challenge is the cost. Controlling the hydrophilicity or hydrophobicity within the catalyst layer, changing the morphology in the catalyst layer, or improving the corrosion resistance of parts had been proved valid to alleviate deterioration from MEA level.
(Mandal et al., 2015) analyzed the change in the Pt/C catalyst after reversal through nano-CT imaging technology and electrochemical diagnosis. The result shows that the reversal results in the collapse of the structure of the anode CL rather than the inter-facial exfoliation between the CL and the PEM. It is generally considered that the performance degradation caused by the reversal is ascribed to the COR of the anode catalyst support. Many previous studies on fuel deficiency have shown that IrO2, as a RTA catalyst, can inhibit the deterioration of COR of MEA. Hu et al. (2019a) found that when the fuel cell operated below the freezing point, the addition of OER catalyst to the anode had little effect on the reversal tolerance ability. Repeated hydrogen starvation tests were carried out on a single cell at a freezing point temperature of −5°C. It was found that there was no significant change in the polarization curve and EIS curve of the fuel cell. By observing the performance at different temperatures, comparing the performance of fuel cells without IrO2 catalyst from 15 to 20°C and fuel cells with 5wt% IrO2 catalyst from 15 to 45°C, they found that carbon corrosion was well suppressed below 0°C. At 10°C and above, the COR of anodes without IrO2 becomes obvious. Katie Heeyum Lim et al. (Lim et al., 2017) found that the application of graphitized carbon support and OER catalyst in the anode can effectively alleviate the effect of voltage reversal even if 1wt% IrO2 was added by monitoring the voltage and the concentration of O2 and CO2 emissions in the anode side by on-line mass spectrometer. The reversal tolerance time increased significantly and carbon corrosion was inhibited at higher temperature (>40°C) with the addition of IrO2. The significantly shortened duration of the water electrolysis platform below the freezing point temperature may be attributed to the limited availability of liquid water due to the limitation of the unfrozen proportion of water and its fluidity.
The addition of OER catalyst is an effective way to alleviate the voltage reversal, but the addition of OER catalyst cannot avoid failure. Leining Hu et al. (Hu et al., 2021) found that the performance degradation still occurs when applying Pt black as an anode catalyst, which is speculated to be caused by COR in the microporous layer of the gas diffusion layer (GDL). Based on this conjecture, they utilized Pt black as an anode catalyst, and the coated anode CL with Ti layer with a thickness of 13 um. The results showed that the decay rate of voltage decreased from 15.2%/min to 0.049%/min, and the durability was increased by approximately 310 times. Wang et al. (2020d) studied the influence of gas humidity on reversal tolerance ability, and found that humidity had the opposite effect on reversal tolerance ability when the inlet humidity was greater than 55%. The reversal tolerance failure time of the anode CL area near the inlet is shorter than that near the outlet, and the reversal tolerance ability is better in the MEA region on the side of the air inlet with a higher loading of IrO2. Joo et al. (2020) Joo suggested that the performance of RTA drops prominently at high humidity because of accelerated poisoning by carbon corrosion species rather than electrical isolation caused by corrosion of carbon support. They found the OER activity of IrO2 increases linearly with relative humidity, while the rate of COR increases exponentially with relative humidity. Chi-Yeong Ahn et al. (Ahn et al., 2021) investigated the influence of different IrO2 content in the anode CL on the performance of the MEA and found that the influence was negligible when the IrO2 content in the anode CL was less than 5%. With further increase in the IrO2 content in the anode CL, the performance decreased with the increase in ohmic resistance, but the durability increased with the increase in the IrO2 content. Xiaoyang Zhou et al. (Zhou et al., 2021) designed an RTA, and after the fuel starvation test, the voltage of the MEA attenuates only 0.12% per hour at 1.2 A/cm2. It is considered that the increase in mass transfer resistance is the primary reason for the increase in anode potential. Moreover, the location of IrO2RuO2 has a huge influence on the reversal tolerance ability. Compared with the three ways of blending, IrO2RuO2 and Pt/C doping, coating the IrO2RuO2 layer first and then coating the Pt/C layer, and coating the Pt/C layer first and then coating the IrO2RuO2 layer, the effect of coating 20wt% IrO2RuO2 catalyst on the layer of Pt/C is the best, and the durability is as long as 853 min, and with the increase in IrO2RuO2 catalyst load, the durability can be as long as 5020 min.
Bentele et al. (2021) reported the normal failure reason is the voltage loss and failure caused by the raising of HOR dynamics and mass transfer resistance, and the increase in mass transport overpotential indicates the collapse of the anode CL caused by COR. In addition, two different HOR catalysts were compared, and it was found that the durability of the RTA was also significantly dependent on the HOR catalyst. Moreover, the OER catalyst is still active after the collapse of the anode CL, and the activity of the surface OER catalyst is not necessarily related to the structural integrity of the anode. Mandal et al. (2018) analyzed the mechanism of antireversal failure by means of electrochemical means and X-ray tomography. The RTA performance improved with the increased loading of IrO2. However, although IrO2 was added, RTA failure would still occur. Through the postimaging images, it was found that there was no significant distribution and morphological change of IrO2 particles in the anode CL. The characteristics and mechanism of failure are similar to those of the MEA without the OER catalyst, which indicates that COR occurs at the same time of water electrolysis, and the collapse of anode CL resulted in the increase in ohmic resistance and the degradation of performance.
(Hong et al., 2016) by means of electrochemical methods and nano-X-ray tomography technology found that the performance of RTA not only attenuates rapidly under the condition of dehydration but also attenuates quantitatively when the humidity increases from 68 to 82%. However, most of the reversal phenomena occur in cold start-up or flooding conditions, which will lead to high humidity conditions. The performance decline of MEA caused by reversal will be accompanied by carbon corrosion, so it can be judged that the final disappearance of OER electrolytic activity is not only the disappearance of IrO2 itself. This puts forward some opinions on how to solve the potential risk of fuel cells based on materials, and emphasizes the necessity of MEA design, material optimization, and operation strategies to improve RTA performance.
Chen et al. (2021b) compared the influence of the thickness of anode CL on the antireversal time and found that the reversal tolerance ability of the traditional anode CL with only Pt/C is poor and the durability was basically the same regardless of the thickness. As shown in Figure 6, for instance, the TEC30-01/20 means the anode CL consists of 0.1 mg/cm2 of TEC10E30TPM Pt/C and 20 mg/cm2 of IrO2. The TEC60-01/50 means the anode CL consists of 0.1 mg/cm2 of TEC10E50TPM Pt/C and 50 mg/cm2 of IrO2. The thinner the anode CL with same loading of IrO2 is, the better the reversal tolerance ability is. The results show that the durability is as long as 310 min when the thickness of the anode CL is approximately 2 um. It is considered that the effect of thickness on the reversal tolerance ability is mainly due to the distribution of ionomer on the surface of the OER catalyst. In addition, the results showed that the durability prolonged with the increase in loading of IrO2 and the mass ratio of ionomer to carbon support.
FIGURE 6. Influence of anode CL thickness and IrO2 loading on the durability of the RTA. Reproduced with permission (Chen et al., 2021b). Copyright 2021, Elsevier.
Cai et al. (2020) monitored the change in the electrical resistance of the MEA during the reversal process and found that the electrical resistance of the MEA increased slowly at the water electrolysis voltage platform, but increased rapidly after the end of the water electrolysis voltage platform. The correction voltage of electrical resistance shows that the end of the water electrolysis platform is not the deactivation or lack of water of OER catalyst, but the increase in internal resistance caused by carbon corrosion. Yu Zhang et al. (Zhang et al., 2018) investigated the degradation of GDL after the durability test; they found the attenuation of GDL accounted for 13.36% of the whole attenuation of MEA. Yajun Wang et al. (Yajun et al., 2019) designed a test pool in which current density sensors are added to the cathode plate, temperature and humidity sensors are added to the anode plate. It is found that the failure of the RTA is primarily ascribed to the destruction of the electronic path in the GDL, CL, and bipolar plate caused by carbon corrosion instead of the inactivation of the OER catalyst. In addition, In addition, adding OER catalyst to the GDL can significantly improve the reversal tolerance ability of fuel cells. Shengyu Fang et al. (Fang et al., 2014) coated anode GDL with TiVCr hydrogen storage alloy by direct current sputtering, the MEA with TiVCr-coated GDL shows greater durability than MEA with uncoated GDL under no hydrogen condition. As shown in Figure 7, the TiVCr layer absorbs hydrogen to saturation and releases hydrogen during starvation, the released hydrogen can mitigate the voltage reversal. Hao et al. (2015) replaced XC-72 with antimony-doped tin oxide as a microporous layer; the GDL with antimony-doped tin oxide MPL shows better durability after oxidation for 55 h.
The high potential of the anode will accelerate the COR and lead to the degradation of the anode CL in a short period, resulting in the irreversible performance attenuation of MEA (Liu and Hou, 2013; Matsuura et al., 2013; Zhang et al., 2017; Malinowski et al., 2019). Zhong et al. (2018) optimized the flow field design by simulation to improve the distribution of hydrogen in the flow field to obtain uniform and distinct current density. Scott et al. (2015) changed the series structure of the traditional stack by changing the structural design of the stack. The bipolar plate, which is used to distribute reactants, was changed to supply only one kind of reactant, and the MEA was also adjusted from the original cathode face to anode to cathode face to cathode. The stack can still work normally after some cells failed. Cell voltage monitor (CVM) has been used to monitor the voltage of individual cells in stack for the control unit to avoid the occurrence of reversal (Debenjak et al., 2015; Vivas et al., 2019; Shin et al., 2020); the additional accessories involved will not only increase the complexity of the system and reduce the cost efficiency, but also cannot solve the reversal problem. The second solution is the improvement of system hardware materials. But it is expensive and complicated for the fuel cell system. Optimizing the control strategy would be effective to prevent voltage reversal. Control strategies generally monitor operation parameters and feedback by adjusting pressure, flow, and load change, and controlling the stack temperature and gas humidity.
Voltage reversal damage can be minimized by adjusting the stoichiometric ratio of fuel and air, temperature, current load, water management, and other operating parameters. Taniguchi et al. (2008) investigated the effect of reversal caused by air deficiency on the performance of MEA. After the reversal test of 120 min, the size of Pt particles in the cathode CL increased and the electrochemical specific surface area (ECSA) of the cathode CL decreased, which accelerated the performance decay and permanent damage of the MEA while there was no degradation in the anode CL. Taniguchi et al. (2004) studied the influence of hydrogen starvation on the performance of the MEA. The EDX of the anode CL clearly showed that Ru was dissolved from the anode CL and serious Ru loss was found in the hydrogen outlet area. Through CO stripping voltammetry and the test of degraded MEA, it was found that the tolerance of MEA to CO decreased. The specific surface area loss of Pt in the cathode CL was found by TEM and CV.
Enhancing the stiochiometric ratio of fuel and air is necessary to avert starvation. Zhou et al. (2015) found that when the stoichiometric ratio of H2 is less than 1, the voltage of the MEA decreases coupled with the local current density that begins to differ, the current density near the hydrogen inlet shows an upward trend, and the current density near the hydrogen outlet shows a downward trend. When the reversal occurs, CO2 and O2 can be detected at the hydrogen outlet. When the load further increases and the stoichiometric ratio decreases, the problem of uneven current density is more serious. Dou et al. (2011) investigated the influence of air starvation by in situ monitoring of the local interface potential, current, and temperature distribution of MEA. The results showed that the local potential of the air inlet area remains positive. In contrast, the local potential of the middle and outlet areas becomes negative during the redox reaction, indicating that the reduction reactions can occur in different regions of the cathode at the same time. Before the occurrence of the reversal, with the decrease in the air stoichiometric ratio, the current distribution will be more uneven. When the voltage reversal occurs, the current will be redistributed and will tend to be uniform. At the critical point of voltage reversal, the nonuniformity of current distribution is the most significant. The temperature distribution in the MEA was also monitored online. When the reversal occurred, lots of hot spots were found in the MEA. The study of air stoichiometric ratio at the critical point of voltage reversal under different loads shows that the critical point of air stoichiometric ratio increases with the increase in load. Bing Li et al. (Li and Drew, 2015) found that the rapid change in loading frequency leads to agglomeration of catalyst particles in the anode, which leads to the decline in the performance of MEA, as the rate of mass transfer cannot meet the load demand. Ye Peng et al. (Peng et al., 2021) compared constant current and pulse-accelerated pressure tests to study the RTA ability of MEA. It was found that the pulse test caused more serious MEA damage than the galvanostatic experiment at the same total reversal time. It is possible that each oxidation/reduction cycle accelerates the dissolution of Pt and carbon corrosion. On the contrary, in the galvanostatic test, the COR is relatively slow due to the accumulation of passivation layer or products. The mainway to solve this problem is to optimize system control strategy and stack structure, in which the exhaust composition of anode and cathode outlet, voltage, and local density are monitored by specially designed software (YehLai and Rapaport, 2013; Hu et al., 2019b; Pan et al., 2019; Chen et al., 2020).
Eskin et al. (Eskin and Yesilyurt, 2019) investigated the effect of three different anode exhaust modes on stability and durability. The differential pressure between the anode and the cathode was 1 bar to reduce the penetration of nitrogen into the anode. The change in ECSA of the CL was compared by cyclic voltammetry (CV). As shown in Figure 8, compared with the dead-end anode mode and flow through mode, the anode bleeding can effectively alleviate the voltage fluctuation and performance degradation through the syringe pump. With the increase in the pumping speed of the injection pump, the fluctuation of voltage is reduced. Although the operation strategies can effectively prolong the lifetime of the fuel cell, it needs peripheral sensors to monitor and back feed information to adjust the parameters to operate stably. Chen et al. (2016) optimized the opening size of the cathode outlet and found the carbon corrosion of support in both sides becomes less serious with the increase in opening size under the dead-ended anode mode. Lizhong Luo et al. (Luo and Jian, 2019) found that the system strategy can help alleviate the fuel cell reversal phenomenon and boost the performance of the stack. By replacing the single-way solenoid valve used in the closed anode with a three-position three-way solenoid valve, the results show that the maximum power of the air-cooler stack increased by 10.96%, which significantly improves the distribution of hydrogen in the anode channel and reduces the voltage fluctuation. In fact, fuel cell reversal does not take long to occur. Lin et al. (2018) found that the step load strategy is more efficient in mitigating the COR caused by reversal during startup and shutdown operations. (KimHan and Jeong, 2018) Juhan Kim et al. provided the stack with anode reversal tolerance during cold start by measuring the cell voltage, by judging whether the voltage is reversal voltage and comparing the voltage with reference value to judge whether in water electrolysis state, if not, limit the current of stack or shut down, if yes, execute cold start.
FIGURE 8. Voltage transient at 0.67 A/cm2 with 3 modes of operation: flow through (A), anode bleeding, (B) dead-end anode (C). In (B), the inset demonstrates the 5-h portion of the specified area. In (C), the 2-h insets show the frequency of the dead-end anode cycles. Reproduced with permission (Eskin and Yesilyurt, 2019). Copyright 2021, Elsevier.
The pros and cons of the aforementioned approaches are summed up in Table 1.
Abnormal operating conditions that cannot provide sufficient H2 to generate the required electron and proton lead to irreversible damage to the MEA and hence the PEMFC stack as a whole. The degradation factors include carbon corrosion, catalyst sintering and agglomeration, and membrane degradation.
Mitigating reversal can be achieved at three different levels of design: 1) materials, 2) MEA, and 3) system. By optimizing the carbon support and adding OER catalyst into the CL, damage due to reversal can be significantly mitigated. Furthermore, changing the morphology and location of the OER catalyst in the CL and replacing the carbon support with conductive oxides can help increase the anode lifespan. At the MEA level, optimizing the design, such as using more voltage reversal tolerant GDL, or protecting the CL by an inactive metal, is a possible solution to alleviate performance degradation. Finally, the anode can be protected by an appropriate control strategy at the system level. Due to limited supply and rapidly increasing prices of Ir, future efforts will have to focus on developing either non-Ir reversal catalysts or a more advanced system level to eliminate the use of Ir in the MEA altogether.
ZX: conceptualization, investigation, writing—original draft; BW: resources; DB: review and editing; SC: review and editing; ZX: supervision; YL: resources; SL: supervision and review.
The authors declare that the research was conducted in the absence of any commercial or financial relationships that could be construed as a potential conflict of interest.
All claims expressed in this article are solely those of the authors and do not necessarily represent those of their affiliated organizations, or those of the publisher, the editors, and the reviewers. Any product that may be evaluated in this article, or claim that may be made by its manufacturer, is not guaranteed or endorsed by the publisher.
The authors acknowledge financial support from the National Key Research and Development Program of China (No. 2018YFB1502502) and the Science and Technology Innovation Program of Hunan Province (2021RC1001).
Ahn, C.-Y., Kang, S. Y., Choi, H. J., Kim, O.-H., Sung, Y.-E., and Cho, Y.-H. (2021). Effect of Anode Iridium Oxide Content on the Electrochemical Performance and Resistance to Cell Reversal Potential of Polymer Electrolyte Membrane Fuel Cells. Int. J. Hydrogen Energ. 4627, 14713–14723. doi:10.1016/j.ijhydene.2021.01.199
Anu, Ksai., and Cindrella, L. (2019). Self-humidifying Novel Chitosan-Geopolymer Hybrid Membrane for Fuel Cell Applications. Carbohydr. Polym. 223, 115073. doi:10.1016/j.carbpol.2019.115073
Bentele, D., Aylar, K., Olsen, K., Klemm, E., and Eberhardt, S. H. (2021). PEMFC Anode Durability: Innovative Characterization Methods and Further Insights on OER Based Reversal Tolerance. J. Electrochem. Soc. 1682, 024515. doi:10.1149/1945-7111/abe50b
Bhanja, P., Mohanty, B., Patra, A. K., Ghosh, S., Jena, B. K., and Bhaumik, A. (2019). IrO2 and Pt Doped Mesoporous SnO2 Nanospheres as Efficient Electrocatalysts for the Facile OER and HER. ChemCatChem 111, 583–592. doi:10.1002/cctc.201801312
Bizon, N., Lopez-Guede, J. M., Kurt, E., Thounthong, P., Mazare, A. G., Ionescu, L. M., et al. (2019). Hydrogen Economy of the Fuel Cell Hybrid Power System Optimized by Air Flow Control to Mitigate the Effect of the Uncertainty about Available Renewable Power and Load Dynamics. Energ. Convers. Manage. 179, 152–165. doi:10.1016/j.enconman.2018.10.058
Cai, C., Rao, Y., Zhou, J., Zhang, L., Chen, W., Wan, Z., et al. (2020). Carbon Corrosion: A Novel Termination Mechanism of the Water Electrolysis Plateau during Voltage Reversal. J. Power Sourc. 473, 228542. doi:10.1016/j.jpowsour.2020.228542
Chalgin, A. (2020). Effect of Supporting Materials on the Electrocatalytic Activity, Stability and Selectivity of noble Metal-Based Catalysts for Oxygen Reduction and Hydrogen Evolution Reactions. Prog. Nat. Sci. 303, 15–23. doi:10.1016/j.pnsc.2020.01.003
Chen, B., Wang, J., Yang, T., Cai, Y., Pan, M., Tu, Z., et al. (2016). Mitigation Studies of Carbon Corrosion by Optimizing the Opening Size of the Cathode Outlet in a Proton Exchange Membrane Fuel Cell with Dead-Ended Anode. Energ. Convers. Manage. 119, 60–66. doi:10.1016/j.enconman.2016.04.043
Chen, K., Laghrouche, S., and Djerdir, A. (2019). Degradation Prediction of Proton Exchange Membrane Fuel Cell Based on Grey Neural Network Model and Particle Swarm Optimization. Energ. Convers. Manage. 195, 810–818. doi:10.1016/j.enconman.2019.05.045
Chen, K., Laghrouche, S., and Djerdir, A. (2021). Performance Analysis of PEM Fuel Cell in mobile Application under Real Traffic and Environmental Conditions - ScienceDirect. Energ. Convers. Manage. 227, 1136002. doi:10.1016/j.enconman.2020.113602
Chen, W., Cai, C., Li, S., Tan, J., and Pan, M. (2021). Thickness Effects of Anode Catalyst Layer on Reversal Tolerant Performance in Proton Exchange Membrane Fuel Cell. Int. J. Hydrogen Energ. 4612, 8749–8757. doi:10.1016/j.ijhydene.2020.12.041
Chen, X., Xu, J., Liu, Q., Chen, Y., Wang, X., Li, W., et al. (2020). Active Disturbance Rejection Control Strategy Applied to Cathode Humidity Control in PEMFC System. Energ. Convers. Manage. 224, 113389. doi:10.1016/j.enconman.2020.113389
Cheng, W. (2015). The Durability Research on the Proton Exchange Membrane Fuel Cell for Automobile Application. Prog. Chem. 274, 424–435. doi:10.7536/PC140807
Cullen, D. A., More, K. L., Atanasoska, L. L., and Atanasoski, R. T. (2014). Impact of IrRu Oxygen Evolution Reaction Catalysts on Pt Nanostructured Thin Films under Start-Up/shutdown Cycling. J. Power Sourc. 269, 671–681. doi:10.1016/j.jpowsour.2014.06.153
Jung, D.-W. (2011). Durability of Polymer Electrolyte Membrane Fuel Cell with Pt/CNTs Catalysts in Cell Reversal Conditions by Hydrogen Starvation. Fuel Cells 116, 866–874. doi:10.1002/fuce.201100034
Dang, D., Zhang, L., Zeng, X., Tian, X., Qu, C., Nan, H., et al. (2017). In Situ construction of Ir@Pt/C Nanoparticles in the Cathode Layer of Membrane Electrode Assemblies with Ultra-low Pt Loading and High Pt Exposure. J. Power Sourc. 355, 83–89. doi:10.1016/j.jpowsour.2017.04.050
Debenjak, A., Petrovcic, J., Boskoski, P., Musizza, B., and Juricic, D. (2015). Fuel Cell Condition Monitoring System Based on Interconnected DC-DC Converter and Voltage Monitor. IEEE Trans. Ind. Electron. 628, 5293–5305. doi:10.1109/tie.2015.2434792
Dhanushkodi, S. R., Tam, M., Kundu, S., Fowler, M. W., and Pritzker, M. D. (2013). Carbon Corrosion Fingerprint Development and De-convolution of Performance Loss According to Degradation Mechanism in PEM Fuel Cells. J. Power Sourc. 24015 (oct), 114–121. doi:10.1016/j.jpowsour.2013.03.033
Dong, L. (2009). Study of the Cell Reversal Process of Large Area Proton Exchange Membrane Fuel Cells under Fuel Starvation. J. Power Sourc. 1942, 847–853. doi:10.1016/j.jpowsour.2009.06.059
Dou, M., Ming, H., and Dong, L. (2011). Behaviors of Protons Exchange Membrane Fuel Cells under Oxidant Starvation. J. Power Sourc. 5, 2759–2762. doi:10.1016/j.jpowsour.2010.11.005
Dustin, B., Ja-Yeon, C., Takeaki, K., and Siyu, Y. (2019). Integrating PGM-free Catalysts into Catalyst Layers and Proton Exchange Membrane Fuel Cell Devices. Adv. Mater. 3131, 1804846. doi:10.1002/adma.201804846
Eskin, M. G., and Yesilyurt, S. (2019). Anode Bleeding Experiments to Improve the Performance and Durability of Protons Exchange Membrane Fuel Cells. Int. J. Hydrogen Energ. 44, 2111047–2111056. doi:10.1016/j.ijhydene.2019.02.152
Fan, L., Zhang, G., and Jiao, K. (2017). Characteristics of PEMFC Operating at High Current Density with Low External Humidification. Energ. Convers. Manage. 150, 763–774. doi:10.1016/j.enconman.2017.08.034
Fang, S.-Y., Huang, R.-H., Teoh, L. G., Hsueh, K.-L., Chao, W.-K., Tsai, D.-C., et al. (2014). Coating TiVCr Hydrogen Storage alloy on the Anode Gas Diffusion Layer of Proton Exchange Membrane Fuel Cells to Improve Performance. J. Power Sourc. 268, 443–450. doi:10.1016/j.jpowsour.2014.06.072
Hao, J., Yu, S., Jiang, Y., Li, X., Shao, Z., and Yi, B. (2015). Antimony Doped Tin Oxide Applied in the Gas Diffusion Layer for Proton Exchange Membrane Fuel Cells. J. electroanalytical Chem. 7561, 201–206. doi:10.1016/j.jelechem.2015.08.035
Hasché, F., Oezaslan, M., and Strasser, P. (2010). Activity, Stability and Degradation of Multi Walled Carbon Nanotube (MWCNT) Supported Pt Fuel Cell Electrocatalysts. Phys. Chem. Chem. Phys. 1246, 15251–15258. doi:10.1039/c0cp00609b
Hong, B. K., Mandal, P., Oh, J.-G., and Litster, S. (2016). On the Impact of Water Activity on Reversal Tolerant Fuel Cell Anode Performance and Durability. J. Power Sourc. 328, 280–288. doi:10.1016/j.jpowsour.2016.07.002
Hou, S. (2019). Enhanced Low-Humidity Performance in a Proton Exchange Membrane Fuel Cell by Developing a Novel Hydrophilic Gas Diffusion Layer. Int. J. Hydrogen Energ. 451, 937–944. doi:10.1016/j.ijhydene.2019.10.160
Hu, L., Hong, B. K., Oh, J.-G., and Litster, S. (2021). Reversal Tolerant Anodes Using Protective Layers for Highly Robust Automotive Fuel Cells. ACS Appl. Energ. Mater. 41, 119–127. doi:10.1021/acsaem.0c02011
Hu, L., Hong, B. K., Oh, J.-G., and Litster, S. (2019). Robust Operation of Fuel Cell Systems in Subfreezing Conditions: A Material-Based Solution to Achieve Better Anode Durability. ACS Appl. Energ. Mater. 210, 7152–7161. doi:10.1021/acsaem.9b01108
Hu, Z. (2021). Mechanistic Insight into the Accelerated Decay of Fuel Cells from Catalyst-Layer Structural Failure. Energ. Convers. Manage. 227, 113568. doi:10.1016/j.enconman.2020.113568
Hu, Z., Xu, L., Li, J., Gan, Q., Xu, X., Song, Z., et al. (2019). A Novel Diagnostic Methodology for Fuel Cell Stack Health: Performance, Consistency and Uniformity. Energ. Convers. Manage. 185, 611–621. doi:10.1016/j.enconman.2019.02.031
Hu, Z., Xu, L., Li, J., Hu, J., Xu, X., Du, X., et al. (2018). A Cell Interaction Phenomenon in a Multi-Cell Stack under One Cell Suffering Fuel Starvation. Energ. Convers. Manage. 174, 465–474. doi:10.1016/j.enconman.2018.08.062
Huang, Z., Shen, J., Chan, S. H., and Tu, Z. (2020). Transient Response of Performance in a Proton Exchange Membrane Fuel Cell under Dynamic Loading. Energ. Convers. Manage. 226, 113492. doi:10.1016/j.enconman.2020.113492
Ioroi, T., and Yasuda, K. (2020). Highly Reversal-Tolerant Anodes Using Ti4O7-Supported Platinum with a Very Small Amount of Water-Splitting Catalyst. J. Power Sourc. 450, 227656. doi:10.1016/j.jpowsour.2019.227656
Jia, F., Guo, L., and Liu, H. (2017). Mitigation Strategies for Hydrogen Starvation under Dynamic Loading in Proton Exchange Membrane Fuel Cells. Energ. Convers. Manage. 139, 175–181. doi:10.1016/j.enconman.2017.02.051
Jia, F., Liu, F., Guo, L., and Liu, H. (2016). Mechanisms of Reverse Current and Mitigation Strategies in Proton Exchange Membrane Fuel Cells during Startups. Int. J. Hydrogen Energ. 4115, 6469–6475. doi:10.1016/j.ijhydene.2016.03.037
Jia, Q., Caldwell, K., Strickland, K., Ziegelbauer, J. M., Liu, Z., Yu, Z., et al. (2015). Improved Oxygen Reduction Activity and Durability of Dealloyed PtCox Catalysts for Proton Exchange Membrane Fuel Cells: Strain, Ligand, and Particle Size Effects. ACS Catal. 51, 176–186. doi:10.1021/cs501537n
Joo, T., Hu, L., Hong, B. K., Oh, J.-G., and Litster, S. (2020). On the Origin of Deactivation of Reversal-Tolerant Fuel Cell Anodes under Voltage Reversal Conditions. J. Power Sourc. 472, 228439. doi:10.1016/j.jpowsour.2020.228439
Kim, H.-E., Shin, S., and Lee, H. (2021). Pt-IrOx Catalysts Immobilized on Defective Carbon for Efficient Reversal Tolerant Anode in Proton Exchange Membrane Fuel Cells. J. Catal. 395, 404–411. doi:10.1016/j.jcat.2021.01.028
Kim, T.-Y., Lee, S. W., and Pak, C. (2020). Optimization of Carbon-Supported Ir-Ru Alloys for Polymer Electrolyte Fuel Cell Anodes under Cell Reversal. J. Ind. Eng. Chem. 85, 87–93. doi:10.1016/j.jiec.2020.01.024
KimHan, J. W. S. B., and Jeong, K. S. (2018). Method of Controlling Operation of Fuel Cell. US Patent. 20180351185A1. (Accessed May 30, 2017).
Knights, S. D., Taylor, J. L., and Wilkinson, D. P. (2003). Supported Catalysts for the Anode of a Voltage Reversal Tolerant Fuel Cell. US20040157110A1: WO.
Kumar, A., Atienza, D., and Ramani, V. (2017). “Pt/RuO2-TiO2 (RTO) as Cell Reversal Tolerant Anode Catalyst for PEFCs,” in 231st ECS Meeting (New Orleans, LA.
Labi, T. (2021). Increasing Fuel Cell Durability during Prolonged and Intermittent Fuel Starvation Using Supported IrOx. J. Power Sourc. 490, 229568. doi:10.1016/j.jpowsour.2021.229568
Lee, S. W., Lee, B., Baik, C., Kim, T.-Y., and Pak, C. (2021). Multifunctional Ir-Ru alloy Catalysts for Reversal-Tolerant Anodes of Polymer Electrolyte Membrane Fuel Cells. J. Mater. Sci. Techn. 60, 105–112. doi:10.1016/j.jmst.2020.05.020
Lee, S. W., Lee, B. H., Baik, C., You, E., You, D. J., and Pak, C. (2020). MagnéLi-Phase Ti4O7-Supported Irru-Based Catalysts for Reversal Tolerant Anode of Automotive Polymer Electrolyte Membrane Fuel Cell. Meet. Abstr. 0138, 1656. doi:10.1149/ma2020-01381656mtgabs
Lee, S. W., Lee, B. H., Kim, T.-Y., Baik, C., Kim, M. S., Chai, G. S., et al. (2019). Multifunctional Non-pt Ternary Catalyst for the Hydrogen Oxidation and Oxygen Evolution Reactions in Reversal-Tolerant Anode. Catal. Commun. 130, 105758. doi:10.1016/j.catcom.2019.105758
Lee, W. H., Ko, Y. J., Kim, J. H., Choi, C. H., Chae, K. H., Kim, H., et al. (2021). High Crystallinity Design of Ir-Based Catalysts Drives Catalytic Reversibility for Water Electrolysis and Fuel Cells. Nat. Commun. 12 (2021), 4271. doi:10.1038/s41467-021-24578-8
Li, Bing., and Drew, C. (2015). The Durability of Carbon Supported Pt Nanowire as Novel Cathode Catalyst for a 1.5 kW PEMFC Stack. Appl. Catal. B: Environ. 162, 133–140. doi:10.1016/j.apcatb.2014.06.040
Lile, Jrd. (2021). First Principles Study of Ir3Ru, IrRu and IrRu3catalysts for Hydrogen Oxidation Reaction: Effect of Surface Modification and Ruthenium Content. Appl. Surf. Sci. 545, 149002. doi:10.1016/j.apsusc.2021.149002
Lim, K. H., Lee, W. H., Jeong, Y., and Kim, H. (2017). Analysis of Carbon Corrosion in Anode under Fuel Starvation Using On-Line Mass Spectrometry in Polymer Electrolyte Membrane Fuel Cells. J. Electrochem. Soc. 16414, F1580–F1586. doi:10.1149/2.0731714jes
Lin, R., Xia, S., Zhang, Q., and Dutruel, B. (2018). Comparison between the Constant Dummy Load and Step Load Shut-Down Strategy for PEMFCs. Fuel Cells 183, 306–314. doi:10.1002/fuce.201700095
Liu, B., Wang, S., Wang, C.-y., Ma, B.-z., and Chen, Y.-q. (2020). Electrochemical Behavior and Corrosion Resistance of IrO2-ZrO2 Binary Oxide Coatings for Promoting Oxygen Evolution in Sulfuric Acid Solution. Int. J. Miner Metall. Mater. 272, 264–273. doi:10.1007/s12613-019-1847-0
Liu, F., Peng, H., Qiao, X., Fu, Z., Huang, P., and Liao, S. (2014). High-performance Doped Carbon Electrocatalyst Derived from Soybean Biomass and Promoted by Zinc Chloride. Int. J. Hydrogen Energ. 3919, 10128–10134. doi:10.1016/j.ijhydene.2014.04.176
Liu, G., Xu, J., Wang, Y., Jiang, J., and Wang, X. (2014). A Novel Catalyst Coated Membrane Embedded with Cs-Substituted Phosphotungstates for Proton Exchange Membrane Water Electrolysis. Int. J. Hydrogen Energ. 3927, 14531–14539. doi:10.1016/j.ijhydene.2014.07.106
Liu, J., and Hou, Z. (2013). Carbon Corrosion in Polymer Electrolyte Membrane Fuel Cell Catalysts and its Mitigation Strategies. Springer Berlin Heidelberg. doi:10.1007/978-3-642-33497-9_3
Luo, L., and Jian, Q. (2019). Experimental Study and Mitigation of Abnormal Behavior of Cell Voltage in a Protons Exchange Membrane Fuel Cell Stack. Int. J. Energ. Res. 43 (5), 1912–1923. doi:10.1002/er.4413
Malinowski, M., Iwan, A., Hreniak, A., and Tazbir, I. (2019). An Anode Catalyst Support for Polymer Electrolyte Membrane Fuel Cells: Application of Organically Modified Titanium and Silicon Dioxide. RSC Adv. 942, 24428–24439. doi:10.1039/c9ra04862f
Mandal, P., Hong, B. K., Oh, J.-G., and Litster, S. (2015). 3D Imaging of Fuel Cell Electrode Structure Degraded under Cell Voltage Reversal Conditions Using Nanoscale X-Ray Computed Tomography. ECS Trans. 6917, 443–453. doi:10.1149/06917.0443ecst
Mandal, P., Hong, B. K., Oh, J.-G., and Litster, S. (2018). Understanding the Voltage Reversal Behavior of Automotive Fuel Cells. J. Power Sourc. 397, 397–404. doi:10.1016/j.jpowsour.2018.06.083
Matsuura, T., Chen, J., Siegel, J. B., and Stefanopoulou, A. G. (2013). Degradation Phenomena in PEM Fuel Cell with Dead-Ended Anode. Int. J. Hydrogen Energ. 3826, 11346–11356. doi:10.1016/j.ijhydene.2013.06.096
Min, Y., Yuchan, Z., Li, D., Ruoxi, M., and Ailian, Z. (2017). IrO2-TiO2 Electrocatalysts for the Hydrogen Evolution Reaction in Acidic Water Electrolysis without Activation. New J. Chem. 4114, 6152–6159. doi:10.1039/c7nj00756f
Moore, C. E., Eastcott, J., Cimenti, M., Kremliakova, N., and Gyenge, E. L. (2019). Novel Methodology for Ex Situ Characterization of Iridium Oxide Catalysts in Voltage Reversal Tolerant Proton Exchange Membrane Fuel Cell Anodes. J. Power Sourc. 417, 53–60. doi:10.1016/j.jpowsour.2019.02.006
Nakashima, N. (2019). Nanocarbons for Energy Conversion: Supramolecular Approaches. Recent Prog. Non-precious Metal Fuel Cel Catalysts 11, 253–276. doi:10.1007/978-3-319-92917-0
Pan, T., Shen, J., Sun, L., and Lee, K. Y. (2019). Thermodynamic Modelling and Intelligent Control of Fuel Cell Anode Purge. Appl. Therm. Eng. 154, 196–207. doi:10.1016/j.applthermaleng.2019.03.009
Pandy, A., Yang, Z., Gummalla, M., Atrazhev, V. V., Kuzminyh, N. Y., Sultanov, V. I., et al. (2013). A Carbon Corrosion Model to Evaluate the Effect of Steady State and Transient Operation of a Polymer Electrolyte Membrane Fuel Cell. J. Electrochem. Soc. 1609, F972–F979. doi:10.1149/2.036309jes
Paul, S., Yong, C., and Rajnish, K. C. (2015). The Effect of Low Platinum Loading on the Efficiency of PEMFC's Electrocatalysts Supported on TiO2–Nb, and SnO2–Nb: An Experimental Comparison between Active and Stable Conditions. Energ. Convers. Manage. 103, 681–690. doi:10.1016/j.enconman.2015.06.050
Peng, H., Liu, F., Qiao, X., Xiong, Z., Li, X., Shu, T., et al. (2015). Nitrogen and Fluorine Co-doped Carbon Catalyst with High Oxygen Reduction Performance, Prepared by Pyrolyzing a Mixture of Melamine and PTFE. Electrochimica Acta 182, 963–970. doi:10.1016/j.electacta.2015.10.012
Peng, H., Mo, Z., Liao, S., Liang, H., Yang, L., Luo, F., et al. (2013). High Performance Fe- and N- Doped Carbon Catalyst with Graphene Structure for Oxygen Reduction. Sci. Rep. 3, 1765. doi:10.1038/srep01765
Peng, Y., Choi, J.-Y., Bai, K., Zhang, Y., and Banham, D. (2021). Pulsed vs. Galvanostatic Accelerated Stress Test Protocols: Comparing Predictions for Anode Reversal Tolerance in Proton Exchange Membrane Fuel Cells. J. Power Sourc. 500, 229986. doi:10.1016/j.jpowsour.2021.229986
Petch, M., Burton, S., Hodgkinson, A., O'Malley, R., and Turner, W. (2015). Mitigation of Fuel Cell Degradation through MEA Design. SAE Int. J. Passeng. Cars - Mech. Syst. 91, 1–5. doi:10.4271/2015-01-1777
Puthiyapura, V. K., Pasupathi, S., and Su, H. (2014). Investigation of Supported IrO2 as Electrocatalyst for the Oxygen Evolution Reaction in Proton Exchange Membrane Water Electrolyser. Int. J. Hydrogen Energ. 39, 51905–51913. doi:10.1016/j.ijhydene.2013.11.056
Roh, C.-W., Kim, H.-E., Choi, J., Lim, J., and Lee, H. (2019). Monodisperse IrOx Deposited on Pt/C for Reversal Tolerant Anode in Proton Exchange Membrane Fuel Cell. J. Power Sourc. 443, 227270. doi:10.1016/j.jpowsour.2019.227270
Rui, L. A. (2021). Investigation of Real-Time Changes and Recovery of Proton Exchange Membrane Fuel Cell in Voltage Reversal - ScienceDirect. Energ. Convers. Manage. 236, 114037. doi:10.1016/j.enconman.2021.114037
Scott, P., Chen, Y., Calay, R., and Bhinder, F. (2015). Experimental Investigation into a Novel Modular PEMFC Fuel Cell Stack. Fuel Cells 152, 306–321. doi:10.1002/fuce.201200212
Shen, J. (2020). Partial Flooding and its Effect on the Performance of a Proton Exchange Membrane Fuel Cell. Energ. Convers. Manage. 207, 1125371–1125378. doi:10.1016/j.enconman.2020.112537
Shin, D., Yoo, S., and Lee, Y.-H. (2020). On-line Water Contents Diagnosis of PEMFC Based on Measurements. Int. J. Precis. Eng. Manuf.-Green Tech. 76, 1085–1093. doi:10.1007/s40684-020-00232-4
Shin, S. (2020). Numerical Analysis on Transport Properties of Self-Humidifying Dual Catalyst Layer via 3-D Reconstruction Technique. Int. J. Hydrogen Energ. 4627, 14639–14650. doi:10.1016/j.ijhydene.2020.07.254
Sibul, R., Kibena‐Põldsepp, E., Ratso, S., Kook, M., Sougrati, M. T., Käärik, M., et al. (2020). Iron‐ and Nitrogen‐Doped Graphene‐Based Catalysts for Fuel Cell Applications. ChemElectroChem 7, 1739–1747. doi:10.1002/celc.202000011
Taniguchi, A., Akita, T., Yasuda, K., and Miyazaki, Y. (2008). Analysis of Degradation in PEMFC Caused by Cell Reversal during Air Starvation. Int. J. Hydrogen Energ. 339, 2323–2329. doi:10.1016/j.ijhydene.2008.02.049
Taniguchi, A., Akita, T., Yasuda, K., and Miyazaki, Y. (2004). Analysis of Electrocatalyst Degradation in PEMFC Caused by Cell Reversal during Fuel Starvation. J. Power Sourc. 130, 42–49. doi:10.1016/j.jpowsour.2003.12.035
Tovini, M. F., Damjanovi, A. M., and El-Sayed, H. A. (2020). Degradation Mechanism of an IrO2 Anode Co-catalyst for Cell Voltage Reversal Mitigation under Transient Operation Conditions of a PEM Fuel Cell. J. Electrochem. Socitety 168, 064521. doi:10.1149/1945-7111/ac0d39
Velikokhatnyi, O. I., Kadakia, K., Datta, M. K., and Kumta, P. N. (2013). Fluorine-Doped IrO2: A Potential Electrocatalyst for Water Electrolysis. J. Phys. Chem. C 11740, 20542–20547. doi:10.1021/jp308947h
Vichard, L., Petrone, R., Harel, F., Ravey, A., Venet, P., and Hissel, D. (2020). Long Term Durability Test of Open-Cathode Fuel Cell System under Actual Operating Conditions. Energ. Convers. Manage. 212, 112813. doi:10.1016/j.enconman.2020.112813
Vivas, F. J., Heras, A. d. l., Segura, F., and Andújar, J. M., (2019). Cell Voltage Monitoring All-In-One. A New Low Cost Solution to Perform Degradation Analysis on Air-Cooled Polymer Electrolyte Fuel Cells. Int. J. Hydrogen Energ. 4425, 12842–12856. doi:10.1016/j.ijhydene.2018.12.172
Wang, H., Gao, Q., Sun, S., Zhang, W., and Yao, S. (2020). CoP@NRGO Composite as a High-Efficiency Water Electrolysis Catalyst for Hydrogen Generation. J. Solid State. Chem. 290, 121596. doi:10.1016/j.jssc.2020.121596
Wang, J., Zhou, X., Li, B., Yang, D., Lv, H., Xiao, Q., et al. (2020). Highly Efficient, Cell Reversal Resistant PEMFC Based on PtNi/C Octahedral and OER Composite Catalyst. Int. J. Hydrogen Energ. 4515, 8930–8940. doi:10.1016/j.ijhydene.2020.01.054
Wang, T., Wu, H., Feng, C., Zhang, L., and Zhang, J. (2020). MoP@NiCo-LDH on Nickel Foam as Bifunctional Electrocatalyst for High Efficiency Water and Urea-Water Electrolysis. J. Mater. Chem. A. 835, 18106–18116. doi:10.1039/d0ta06030e
Wang, Y., Xie, X., Zhou, C., Feng, Q., Zhou, Y., Yuan, X.-Z., et al. (2020). Study of Relative Humidity on Durability of the Reversal Tolerant Proton Exchange Membrane Fuel Cell Anode Using a Segmented Cell. J. Power Sourc. 449, 227542. doi:10.1016/j.jpowsour.2019.227542
Wh, A. (2021). Performance Prediction of Proton-Exchange Membrane Fuel Cell Based on Convolutional Neural Network and Random forest Feature Selection. Energ. Convers. Manage. 2432, 114367. doi:10.1016/j.enconman.2021.114367
Xiong, Z. a., Liao, S., Dang, D., Tian, X., Hou, S., Liu, F., et al. (2015). Enhanced Water Management in the Cathode of an Air-Breathing PEMFC Using a Dual Catalyst Layer and Optimizing the Gas Diffusion and Microporous Layers. Int. J. Hydrogen Energ. 4010, 3961–3967. doi:10.1016/j.ijhydene.2015.01.091
Yajun, W., Chang, Z., and Xiaojun, X. (2019). Study of Failure Mechanisms of the Reversal Tolerant Fuel Cell Anode via Novel In-Situ Measurements. Int. J. Hydrogen Energ. 451, 996–1007. doi:10.1016/j.ijhydene.2019.10.189
Yanzhou, Q., Du, Q., Mingzhe, F., Yafei, C., and Yan, Y. (2017). Study on the Operating Pressure Effect on the Performance of a Proton Exchange Membrane Fuel Cell Power System. Energ. Convers. Manage. 142, 357–365. doi:10.1016/j.enconman.2017.03.035
YehLai, H., and Rapaport, P. A. N (2013). Stack Operation Method Aimed at Cell Reversal Prevention. US patent, 8524408B2. (September 25, 2009).
Yi, Y. (2012). A Review on Performance Degradation of Proton Exchange Membrane Fuel Cells during Startup and Shutdown Processes: Causes, Consequences, and Mitigation Strategies. J. Power Sourc. 2052, 10–23. doi:10.1016/j.jpowsour.2012.01.059
You, C., Liao, S., Li, H., Hou, S., Peng, H., Zeng, X., et al. (2014). Uniform Nitrogen and Sulfur Co-doped Carbon Nanospheres as Catalysts for the Oxygen Reduction Reaction. Carbon 69, 294–301. doi:10.1016/j.carbon.2013.12.028
You, E., Lee, S. W., You, D., Lee, B., and Pak, C. (2020). Effect of Metal Composition and Carbon Support on the Durability of the Reversal-Tolerant Anode with IrRu Alloy Catalyst. Catalysts 108, 932. doi:10.3390/catal10080932
Zhang, G., Shen, S., Guo, L., and Liu, H. (2012). Dynamic Characteristics of Local Current Densities and Temperatures in Proton Exchange Membrane Fuel Cells during Reactant Starvations. Int. J. Hydrogen Energ. 37 (2), 1884–1892. doi:10.1016/j.ijhydene.2011.04.120
Zhang, X., Yang, Y., Guo, L., and Liu, H. (2017). Effects of Carbon Corrosion on Mass Transfer Losses in Proton Exchange Membrane Fuel Cells. Int. J. Hydrogen Energ. 427, 4699–4705. doi:10.1016/j.ijhydene.2016.08.223
Zhang, Y., Tan, J. T., and Pan, M. (2018). Study on Durability of Gas Diffusion Layers in the Proton Exchange Membrane Fuel Cells. Dissertation for the Master's Degree. Wuhan: Wuhan University of Technology.
Zhao, N. (2020). Effects of Fuel Cell Operating Conditions on Proton Exchange Membrane Durability at Open-Circuit Voltage. Fuel Cells 2, 176–184. doi:10.1002/fuce.201900173
Zhong, D., Lin, R., Liu, D., and Cai, X. (2018). Structure Optimization of Anode Parallel Flow Field for Local Starvation of Proton Exchange Membrane Fuel Cell. J. Power Sourc. 403, 1–10. doi:10.1016/j.jpowsour.2018.09.067
Zhou, F., Andreasen, S. J., and Kær, S. K. (2015). Experimental Study of Cell Reversal of a High Temperature Polymer Electrolyte Membrane Fuel Cell Caused by H2 Starvation. Int. J. Hydrogen Energ. 4020, 6672–6680. doi:10.1016/j.ijhydene.2015.03.148
Zhou, X., Ji, H., Li, B., and Zhang, C. (2020). High-Repetitive Reversal Tolerant Performance of Proton-Exchange Membrane Fuel Cell by Designing a Suitable Anode. ACS Omega 5, 10099–10105. doi:10.1021/acsomega.0c00638
Zhou, X., Yang, Y., Li, B., and Zhang, C. (2021). Advanced Reversal Tolerant Anode in Proton Exchange Membrane Fuel Cells: Study on the Attenuation Mechanism during Fuel Starvation. ACS Appl. Mater. Inter. 132, 2455–2461. doi:10.1021/acsami.0c16541
Keywords: voltage reversal, oxygen evolution reaction, carbon corrosion, water electrolysis, durability, reversal tolerance anode
Citation: Xiong Z, Wen B, Banham D, Chan SH, Xie Z, Liang Y and Liao S (2022) Methods for Remit Voltage Reversal of Proton Exchange Membrane Fuel Cells. Front. Energy Res. 10:844729. doi: 10.3389/fenrg.2022.844729
Received: 28 December 2021; Accepted: 04 March 2022;
Published: 30 March 2022.
Edited by:
Daniel Zanetti De Florio, Federal University of ABC, BrazilReviewed by:
Chanho Pak, Gwangju Institute of Science and Technology, South KoreaCopyright © 2022 Xiong, Wen, Banham, Chan, Xie, Liang and Liao. This is an open-access article distributed under the terms of the Creative Commons Attribution License (CC BY). The use, distribution or reproduction in other forums is permitted, provided the original author(s) and the copyright owner(s) are credited and that the original publication in this journal is cited, in accordance with accepted academic practice. No use, distribution or reproduction is permitted which does not comply with these terms.
*Correspondence: Zhiyong Xie, eHp5NTA3QGNzdS5lZHUuY24=; Shijun Liao, Y2hzamxpYW9Ac2N1dC5lZHUuY24=
Disclaimer: All claims expressed in this article are solely those of the authors and do not necessarily represent those of their affiliated organizations, or those of the publisher, the editors and the reviewers. Any product that may be evaluated in this article or claim that may be made by its manufacturer is not guaranteed or endorsed by the publisher.
Research integrity at Frontiers
Learn more about the work of our research integrity team to safeguard the quality of each article we publish.