- 1Laboratory for Energy and Nano Science, Department of Mechanical Engineering, Khalifa University of Science and Technology, Abu Dhabi, United Arab Emirates
- 2Department of Physics and Technology, UiT The Arctic University of Norway, Tromsø, United Arab Emirates
Concentrating photovoltaics (CPV) have long been held up as a solution to low power density in photovoltaics, but due to the requirement of sun tracking have been largely unable to realize high power densities in practically useful settings. The emerging concept of tracking-integrated CPV, in which the sun tracking apparatus is incorporated into the module itself, has the potential to finally achieve this goal by allowing CPV use in building integrated or rooftop settings. In this article, we will provide a status update on TI-CPV and an evaluation of its technical and economic potential with focus on diffuse light collection. We will seek to demonstrate how TI-CPV concepts that are now nearing commercialization are viable to offer, for the first time, the chance for CPV to actually deliver high power densities and high-efficiency utilization of the solar resource in practical settings such that it represents one of the best prospects for CPV to finally gain a foothold in large commercial markets. We identify TI-CPV designs with integrated mechanical tracking and diffuse light transmittance as the closest at resent to commercial feasibility, as the transmitted light offers a potential valuable secondary output. A semi-empirical performance model of such a system yields an annual electrical output of >300 kWh/m2 and 59.4 million lux-hours optical output that are equivalent to 593.4 kWh/m2 if that light would otherwise be provided by LED lamps with 100 lumen/W luminous efficacy. This would indicate that full-system capex of up to $1,600/kW could be viable relative to conventional rooftop PV systems, providing a benchmark for future manufacturing and design improvements.
Tracking-Integrated Concentrator Photovoltaics: Concept and Past Work
In the last decade, the adoption of solar photovoltaics has accelerated dramatically under the influence of falling hardware costs and increased investor confidence in the bankability of solar energy (Apostoleris et al., 2019a) Some significant challenges remain for photovoltaics deployment in some settings, in particular the issue of low power density which can limit the usefulness of photovoltaics in space-constrained settings such as rooftops and urban environments. Since its development decades ago, concentrator photovoltaics has promised to increase power density and improve the economics of photovoltaic systems by enabling the use of expensive, high-performance solar cells. However, in practice, these high power densities have seldom been realized, primarily due to the loss of the diffuse component of the solar resource, and the requirement of sun tracking. The inability of CPV to collect diffuse sunlight means that module efficiencies obtained under standard concentrator test conditions (CSTC) do not translate to power output in the same way as non-concentrating module efficiencies. The sun-tracking requirement limits CPV in a number of ways: by requiring ground-mounted CPV modules to be spaced widely, increasing the land use requirements for a CPV plant of a given MW size; and by, in most cases, entirely precluding it from being used in rooftop and building-integrated settings where high power density would have the most value. Over the past decade, the concept of tracking integration, in which the sun-tracking mechanism is incorporated into the CPV module itself, has arisen as a potential avenue to finally realizing high power densities in high-value applications from CPV, by allowing CPV modules to be installed at fixed orientation in the same settings as conventional PV. In 2016, some of the present authors surveyed the various physical designs that had been proposed or demonstrated for tracking-integrated CPV (TI-CPV) modules and systems. In the intervening years, significant progress has been made towards the refinement and commercialization of TI-CPV, to the point where it now stands on the cusp of becoming a viable photovoltaic technology with unique application potential. In part this progress has taken the form of new variations on existing TI-CPV concepts; however, there has also been a more fundamental shift in the way that CPV systems are designed to maximize overall value, rather than seeking kWh-for-kWh parity with conventional PV. To date, this shift has been laid out only partially and in piecemeal fashion in the literature. In this synthetic review article, we will address this shift directly through the implementation of a quantitative value model for TI-CPV, in addition to surveying progress in TI-CPV design since 2015.
The concept of tracking-integrated concentrator photovoltaics (TI-CPV) dates back at least to 2005 (Pender, 2005) with published research beginning in earnest around 2010 (Hallas et al., 2010). Published work through 2015 was reviewed by several of the present authors when the technology was still in the experimental phase, and its application potential remained unclear (Apostoleris et al., 2016). TI-CPV was originally conceived at a time when concentrator photovoltaics (CPV) generally were still viewed as a promising technology to bring down the cost of solar energy to commercially viable levels (Swanson, 2000). The requirement of sun tracking presented a major impediment to CPV as solar energy was seen largely as a rooftop technology, and the need for a sun tracking mechanism made CPV unfeasible for installation on most roofs. For this reason, a substantial amount of work was put into finding a solution for sun tracking that could be implemented in a static module, capable of being mounted in a fixed position like a conventional solar panel. Proposed systems could be classified, broadly, into three categories: micro-tracking systems, in which the cells, optics or both are realigned continuously to maintain illumination of the cells over a wide range of solar incidence angles; beam steering systems, in which the incident light is redirected to maintain normal incidence on a concentrator array; and miscellaneous concepts to which an example are reactive or self-tracking systems, a grab-bag of different designs sharing the feature of responding directly to the solar incidence angle, dynamically modifying themselves to maintain illumination of the cells without the need for external control. Within these categories, designs can be broken into mechanical and non-mechanical systems, depending on whether the cells or optical components are physically moved, or whether the redirection of light is accomplished through a modification of some component’s optical properties, such as refractive index or transparency.
The initial review (Apostoleris et al., 2016) done by some of the present authors was published at a time when the transformation of solar energy economics was just becoming clear, as new refining capacity produced a glut of polysilicon, driving down material costs, and the boom in global PV manufacturing set silicon module prices on a headlong rush down the learning curve that continued throughout the rest of the decade (Green, 2019). CPV researchers and manufacturers increasingly struggled to find a market for their innovations as silicon photovoltaics first reached, and in many places fell below, parity with fossil fuels as an energy generation technology (Apostoleris et al., 2018). While in principle the higher efficiency of CPV systems based on high-performance multijunction solar cells made them a promising candidate for space-constrained applications such as rooftops, in practice this higher efficiency rarely translates into significantly higher annual energy production (Ong et al., 2013). This is due to the limited tracking range of TI-CPV systems, the strong dependence of optical coupling efficiency on incidence angle, and the loss of the diffuse component of sunlight that is inherent to solar concentrator systems. Developments of the past several years have made progress towards addressing these challenges and moved towards the construction of TI-CPV designs that can actually deliver substantially higher daily and annual energy densities than even high-performance silicon PV products. In addition, laboratory-scale work has expanded the design space for TI-CPV systems in ways that raise interesting possibilities for its future development.
New Progress in TI-CPV
The categories of systems surveyed in this section will be organized similarly to the review of Apostoleris et al. (2016), with each section spanning a range of developments from design concepts to fully realized pre-commercial modules.
Beam Steering
A number of advancements in broad-band beam-steering systems have been realized in recent years; these can be used not only as tracking systems for solar concentrators but also for other systems such as smart windows or skylights. Electrowetting-based liquid prism beam steering systems represent the one major area where non-mechanical approaches to sun tracking have seen development. The most traditional beam steering mechanism is visualized in Figures 1A,B.
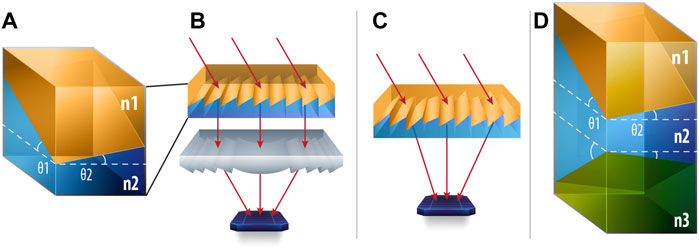
FIGURE 1. Evolution of electrowetting-based beam-steering tracker concepts, from (A) an array of simple EW prisms with a single water-oil interface, modifying the propagation angle of sunlight to (B) maintain uniform illumination of a Fresnel lens, to (C) independent control of the voltage and consequent wetting angle in each prism to achieve simultaneous beam steering & concentration without the need of a separate concentrating lens (Khan et al., 2020); and (D) realization of larger steering angles by use of a compound prism with two interfaces between three different immiscible liquids (Chen et al., 2021).
Khan et al. (2020) designed a two-axis beam steering hexagonal cell array that eliminates the need of further concentration by a Fresnel lens, as shown in Figure 1C. Compared to a square cell, a hexagonal shape attains a slightly higher “fill factor” and encourages higher degrees of freedom in controlling its rotation. The fill factor in this context (distinct from the photovoltaic IV curve parameter) is the distance between opposite faces of the cell for a fixed volume and thickness. CFD simulation using the Laminar Two-phase Flow Moving Mesh (LTPFMM) module of COMSOL Multiphysics software and experimental tests show that applying 26 V to one of the electrodes in the cell caused a maximum contact angle of 44°. Moreover, a small difference of 0.2 in the refractive indices of the fluids revealed a 4.5° deflection in path. The same authors provided additional review of the latest technologies and research utilizing electrowetting-controlled liquid lenses for solar beam steering (Khan et al., 2017), detailing the process and components with insight onto the future possibilities and guidelines on design and component selection.
Chen et al. (2021) developed a programmable beam-steering compound prism (PCP) powered by a triboelectric nanogenerator (TENG) and a resistance-capacitor (RC) circuit that converts AC output signal from the TENG into DC to eliminate the additional costs and maintenance of a DC power supply. The RC circuit controls the prism angle by changing the resistance which in turn modifies the applied voltage. The DC voltage is required to activate the interface between two oils and water in the prism (Figure 1D) and cause the deflection of sunlight. The steered, now perpendicular, light is then concentrated onto a multi-junction cell by a Fresnel lens. The proposed design was able to steer light at an acceptance incident angle of 15° which is 38% higher than conventional single prisms. Strong concentration was seen as the power collected by the multijunction cell increased to 1.288 mW from 0.088 mW in the case of its absence.
Mechanical approaches to beam steering have been demonstrated as well with (Johnsen et al., 2020) developing a method for optimizing the optical design of lens-based beam steering arrays which are adjusted by mechanically shifting the different optical layers relative to each other. In this regard the approach has commonalities with what has come to be called micro-tracking, which represents the largest area of activity in the tracking-integration space.
Micro-Tracking
Price et al. (2017) demonstrated a high-performance planar micro-tracking CPV minimodule based on their previously presented concept of combining refractive and reflective optics to achieve image-field flattening. The design in Figure 2A entails a catadioptric stack which consists of a planoconvex top refractive and bottom reflective optics sandwiching a central glass sheet at which a 3J microscale cell is placed. The glass sheet laterally slides between the optics using an index-matching oil and is controlled by a microcontroller running an algorithm derived from feedback of the measured short-circuit current. Optical efficiency exceeds 90% at 0° angles of incidence and begins dropping beyond 70° at a practical concentration that ranges between ×300 and ×400 with standard optical materials and up to ×660 with higher index optics. The electrical conversion efficiency remains around 30% between 10:00 and 14:00 in outdoor testing and exhibits a lower efficiency than the bare 3J cell due to cell heating (cell heating, a persistent challenge for CPV, can be significantly reduced by moving towards a “micro-CPV” paradigm in which sub-mm cells are utilized to boost heat dissipation from the edge (Domínguez et al., 2017). This performance approaches that predicted by (Grede et al., 2016) in the same group, who established a phase space procedure to optimize planar micro-tracking CPV, discussed its thermodynamic limits and provided design guidelines for such systems along with the practical limits induced by absorption and dispersion.
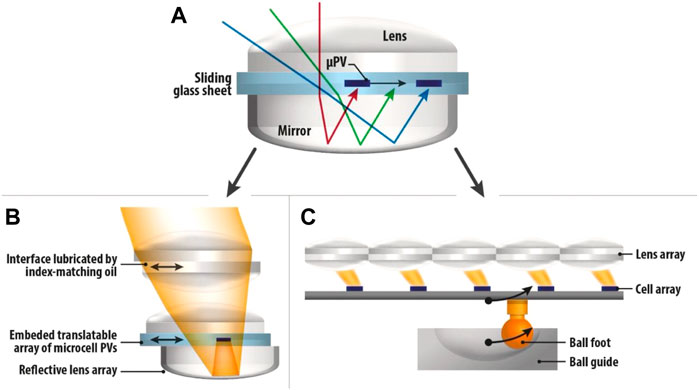
FIGURE 2. (A) Ray tracing diagram showing the capability of the middle glass sheet in sliding to align the cell with the focal spot at different angles (Price et al., 2017). (B) Increased concentration due to the additional stack and movement of lenses in a curved trajectory relative to each other (Johnsen et al., 2019). (C) XY and complimentary Z movement of the cell array is controlled by a ball foot and ball guide combination (Ito et al., 2018).
Additional work by Johnsen et al. (2019) applied the methodology of their previous work on beam-steering to optimize the concept previously outlined (Price et al., 2017) by modifying the lens curvatures and translation paths; three variations were reported. Simulation of the final and most complex configuration (represented in Figure 2B) with a 0.27° divergence half-angle of sunlight showed a concentration factor of ×5,000 compared to ×400 in the first (similar to the design of Price et al.) with a tracking range of ±60°.
Other researchers (Ito et al., 2018) studied the performance of a fixed ×127 bi-convex aspheric lens array and a micro-scale PV cell array that moves in three dimensions to match the image field curvature. Two actuators move the cell array in the XY directions while the movement is controlled by a ball foot and ball guide combination that enables a complimentary tracking mechanism in the Z direction as presented in Figure 2C. The lens aperture is made hexagonal to allow integration into a module-scale array. Results show that the proposed lens outperforms an optimized bi-convex lens having the same concentration in terms of the direct light that can converge onto the cells out of the yearly available by ∼1.7 times. It also demonstrates a higher optical efficiency over a wider range of incidence angles in addition to a shorter focal length. With an acceptance angle of ±40°, the maximum optical efficiency achieved is 82% which remains high at incidence angles below 50° and then steeply declines at higher angles. Compared to a conventional 17%-efficient Si cell, the power generated by the 3J cell in the proposed design is 1.32 times larger per lens aperture area of 929 Wh/m2.
A dual-shell spherical lens variation on this design was tested (Nakatani and Yamada, 2018) in which the shells are composed of different materials with different refractive indices. Optimization of the shells’ material and radii based on the normalized focal length led to an improved maximum allowable angle of 44° and a 76% maximum optical efficiency. Further analysis (Nakatani and Yamada, 2019) validated experimentally showed that compared to a conventional homogenous spherical lens, the proposed core-shell lens is superior in terms of optical and electric efficiencies and CPV cell’s fill factor due to the illumination uniformity on the cell and less chromatic aberrations. The effect of increasing the focal length also had a smaller impact. Performance was found to be sensitive to optical manufacturing defects, a potential challenge to commercial viability.
While exhibiting high performance at concentration of direct light, the previously discussed class of designs suffers from complete loss of the diffuse component of sunlight, which is reflected out the from surface. Designs which allow diffuse light to pass through allow uses for this “lost” component to be found, which will be shown to be one of the key factors to realizing high power densities and economic value. Numerous variations on this concept have been demonstrated.
The idea of coupling micro-scale CPV cells with an underlying PV cell to capture diffuse light has been explored extensively. Haney et al. (2014) proposed that a hybrid design at a concentration of ×100 for the CPV cells is capable of improving the energy production per area of a CPV module by 15%–40% depending on the location in the US. An abundance of prototypes, mostly without integrated tracking, have been made based on this concept (Yamada and Okamoto, 2013; Paap et al., 2014; Lee et al., 2016b; Li et al., 2018; Sato et al., 2019; Yamada et al., 2013), recently extending to the combination of CPV with bifacial solar cells to collect both diffuse and ground-reflected irradiance along with a standardized procedure proposed to rate the power output measured outdoors under standard test conditions (STCs) and standard operating conditions (SOCs) (Martínez et al., 2021). However, in the last few years the concept of simply transmitting diffuse light for illumination purposes (Hirai et al., 2015) has gained popularity. In combination with tracking integration, this has the potential to be a powerful technology for application in buildings or greenhouses (Apostoleris and Chiesa, 2019).
The most fully developed tracking integrated CPV systems at this time appear to be the commercially oriented modules of the Swiss start-up Insolight. A micro-tracking feature operated by electrical actuators is implemented in which the backplane moves laterally and vertically to maintain illumination of the cells. Based on high efficiency multi-junction solar cells, two different architectures were designed. Transparent biconvex aspheric lenses made into a hexagonal tile concentrate the direct light component at a factor of ×180 onto the cells for angles of incidence up to 55°. A secondary glass half-ball lens is added on each cell to mitigate manufacturing errors and boost the acceptant angle. In the first architecture (Figure 3A), diffuse light is converted in large-area silicon PV cells mounted on the back surface (Askins et al., 2019). In the second, the light is transmitted through to provide illumination as in Figure 3B. This product is being targeted at agricultural applications (Nardin et al., 2020). In an additional feature, dynamic control of the tracking allows the operational mode to be switch from electricity generation to maximizing light transmission by focusing or defocusing light on the cells.
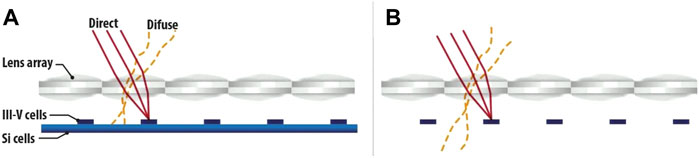
FIGURE 3. (A) In addition to micro-tracking, overall conversion rate is increased by embedding diffuse light onto Si cells (Askins et al., 2019). (B) Diffuse light being transmitted for daylight or agriculture applications (Nardin et al., 2020).
A number of additional demonstrations of new micro-tracking concepts have been presented in recent years. A planar solar tracking system automated to move in dual axis was studied (Lim et al., 2016) in which the overall system consists of three plates in which the top plate is fixed to the step motors that trigger the lateral displacement and the middle plate holds the micro-lens array and is attached to the top plate via spring-loaded poles. The lower plate supports the dual-junction cells and has four blocks that push or pull the middle plate vertically to hold the spot size constant as the top plate forces a lateral displacement to maintain light focus on the cell. An increase of short current density by ×6.8 times was recorded for a system with a geometric concentration factor of ×8.6.
Teng et al. (2020) presented a two-staged planar concentrator coupled with a hybrid tracking mechanism to guarantee a higher concentration ratio, increased efficiency, and more uniformed illumination. The first stage consists of a stack of sheet collectors cut into an arc shape and attached to a rectangular thin light guide plate (LGP) to form an array at which light normally hits onto the arc-segment and gets focused to be horizontally converged at the LGP exit. A wide, curved prism then redirects light onto a boundary. Consequently, light is received onto a transversely moving assembly of light guide channel (LGC) and compound parabolic collectors (CPCs). The LGC slides to align with the light spot exit at the sheet and guides the light onto the CPC uniformly for further concentration and finally, impingement onto the solar cell. The whole structure is mounted onto a single-axis tracker with a tilt angle consistent to the latitude. Optimization of the design parameters promises to yield throughout the year an average optical efficiency of 87%, uniformity of 0.875, and a relatively high concentration ratio of ×738. One main advantage that is not highly highlighted upon is the transparency of the system and parameters that characterize its transmissivity and usefulness for dual use.
Miscellaneous Concepts
A smaller body of research has focused on implementing tracking integration in solar thermal collectors. An optical system designed (Li et al., 2017) involves a set of five prism arrays mounted on a belt with rollers to manoeuvre their movement over a Fresnel lens through which light is redirected normally and then impinged onto a compound parabolic concentrator (CPC) and the stationary tube receiver. As the acceptance angle of the CPC is large, only hourly adjustments in the prism array are needed to maintain illumination of the receiver. The maximum optical efficiency, at 0° is around 90% with a drastic decrease beyond 45°.
Lower-concentration CPV systems have invited creative new approaches to integrating optics with tracking. Lee et al. (2016b) developed an array of origami-inspired hexagonal parabolic concentrators that concentrate light onto GaAs solar cells that are attached on the top tangential plane of sine-wave film of origami trackers as shown in Figure 4. The lateral movement of a plate by an actuator deviates the solar cells from the center of the trackers and is linearly translated to a change in the rotation angle of the solar cells. The shape and size of the concentrator were designed such that to maximize the energy-harvesting efficiency by analyzing the packing density of the usable concentrator area and the light collection efficiency which characterizes the amount of light directed at the solar cells from the concentrator. The light-harvesting efficiency obtained by an array of hexagonal parabolic concentrators is 88.5%. With a concentration up to six suns, a minimal energy requirement of 2.9 J/m2/day for tracking, and a tracking range of ±70°, the utilization of the semiconductor is diminished by 450% in this low-profile planar photovoltaic.
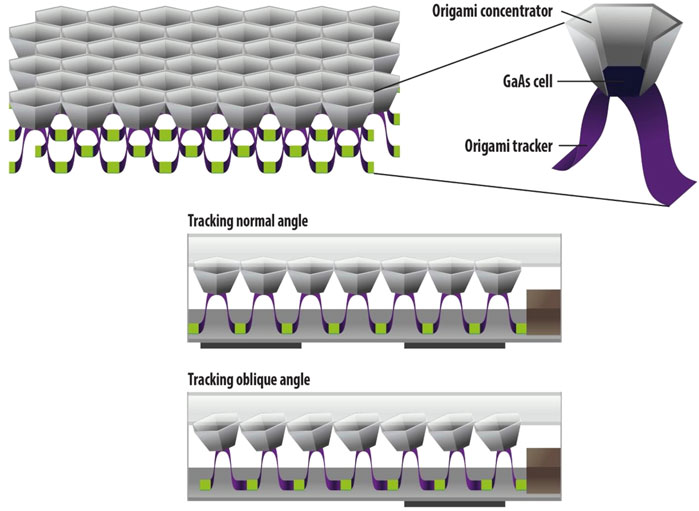
FIGURE 4. An array of “origami” concentrators is rotated by a laterally moving actuator that moves laterally to maintain alignment with incoming sunlight (Lee et al., 2016a).
Wang et al. (2017) developed a macroscale beam steering mechanism highlighting planar smart, deployable, soft frame structures that encapsulate kirigami and origami reflective films. In terms of design structure, the difference between the kirigami and origami films is the orientation of the reflective surfaces in which the reflectors are aligned in a unidirectional manner in the kirigiami and bidirectional in the origami. The reflection/feature angle is achieved by the autonomous axial strain of the films imposed by the continuous deformation of the low-cost, lightweight structures. The large and reversible deformation of the structures is triggered by the longitudinal contraction of a shape-memory alloy (SMA) wire at its austenitic phase transition temperature as a result of Joule heating once electric current is applied. At both configurations, results show that a wide range of deflection angles can be achieved in controllable and reliable means. In the unidirectional reflection kirigami, reflective angle ranges from 0° to 75°. For the bidirectional origami structure, light beaming at high feature angles is directed on two reflective surfaces and only one in the events of smaller angles bringing about two ranges are exhibited in which it decreases from 60° to 12.8°, then hops to 82.8° and declines to 0°.
Another dual-axis tracker that can be integrated with several optics based on kirigami was designed and tested for mechanical fatigue, optical operation and shadowing, electrical performance, and thermal dissipation (Evke et al., 2020). A 2D pattern of discontinuous concentric hexagonal rings expands in 3D to form a spring of three rings once a force is applied in the middle of the pattern. The spring displaces, forming a tilt angle that corresponds to the sun’s position with the addition of a photodiode and a hexagonal parabolic mirror inserted in the middle ring.
Lower-concentration systems also allow for the use of line-focus concentrators with single-axis tracking. A planar-type CPV system was proposed by (Lee et al., 2019) in which cylindrical lenses concentrate light onto thin compound semiconductor GaAs solar cells incorporated onto the inner rounded surface of the lenses. A microcontroller unit scans the angles and records the current in order to find the optimum incident angle for power conversion. To ensure that the width of the concentrated beam width remains uniform at all angles of incidence, reduce Fresnel losses, and guarantee that the incident light remains is directed in a normal direction to the cell, an automated single-axis tracker rotates the cylinder throughout the day via a motor, as demonstrated in Figure 5. Results reveal an increase in short circuit current of roughly ×8 in comparison to a reference cell without concentration.
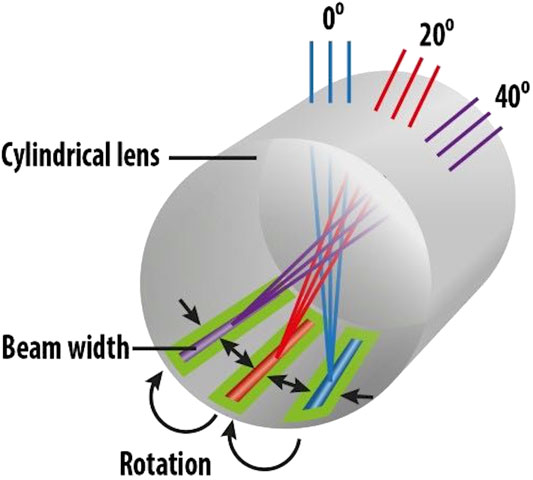
FIGURE 5. A line-focus concentrator based on continuous rotation of a cylindrical lens focusing onto a “strip” of solar cells (Lee et al., 2019).
Finally, a rare innovation in reactive tracking that avoids the use of conventional actuators is considered. To eliminate the need of a motor in the design of Price et al. (2017), Grede et al. (2018) proposed the utilization of shape memory alloy (SMA) wires that can apply the force instead to translate the cells. By means of resistive heating, the wires change lengths and move the cell array sheet at a high accuracy if optical feedback can be provided. Power consumption of such a mechanism did not exceed 1% of the output a full-scale module.
In addition to the concepts described here, Alves et al. (2019) provided further insight into compact concentrator technologies in their review on fabrication of thin-film micro solar cells.
To summarize, there remains a large conceptual space being explored by tracking-integration researchers. In particular, a wide variety of designs have been implemented on the lab-prototype scale seeking to avoid the need for any mechanical tracking. This is worthwhile goal as small mechanical components required for microtracking can be expected to impose at least some additional maintenance cost and risk of failure. However, the basic problem of CPV design—the simultaneous optimization of optical throughput, concentration factor and tracking range, while maintaining manufacturability and long-term reliability—is a convoluted one with many counteracting physical principles in play (Apostoleris et al., 2019b). While non-mechanical systems such as fluidic beam-steering systems represent impressive feats of design, the path to large-scale manufacturability at present remains unclear. Perhaps in recognition of this, high-level “attention” in terms of large research grants and startup financing has gravitated towards the more conservative concepts—generally those based on planar microtracking—with growing interest in utilization of diffuse sunlight. Indeed, it is primarily these that have been practically demonstrated on a module scale, as indicated by the summary of experimentally confirmed performance parameters in Table 1. This indicates that major players see at least the potential for economic success in these technologies. In the subsequent sections, an argument is built up for the economic viability of tracking-integrated CPV with diffuse light utilization, and the conditions that should be met in order for this success to be realized are considered.
Modeling TI-CPV Performance
Model Description
In order to evaluate TI-CPV performance accurately, it is necessary to go beyond raw figures such as efficiency and consider the interaction with the full solar resource in the location and over the time period of interest—in other words, to implement a performance model. Companies developing TI-CPV products have developed their own modeling tools which are specific to their products, and furthermore are typically proprietary and as such of limited use to the general research community. Nardin et al. (2020) described a modeling approach for general TI-CPV systems in the Python PVLIB framework, which uses a single-diode model for both CPV and PV components of a hybrid system as described in the previous section, adding correction factors for temperature and spectral impacts on optical performance. This model was validated with real-world data from outdoor tests of Insolight’s modules at Madrid Polytechnic University and is under continued development as a high-accuracy modeling tool.
In the past, the present authors have used a scaled-down performance modeling methodology for “ballpark” estimates and particularly for comparison to established PV technologies. Intended for ease of use, the electrical performance model is semi-empirical model and depends only on the plane-of-array direct irradiance and direct light incidence angle, in addition to the solar-to-electric efficiency as a function of the incidence angle. This is often approximated as a step function characterized by a single efficiency, typically measured at normal incidence, and a maximum tracking angle. All parameters should be measured under “real” conditions to the extent possible—e.g., use an outdoor measurement of efficiency, in a climate similar to that where it will be deployed, rather than one at standard test conditions. For more accurate modeling and extrapolation to other climates, factors to account for ambient temperature and spectral variations should be included; accurately evaluating these is the subject of ongoing work. The same approach can be taken to evaluating light-splitting hybrid systems—either CPV-PV or semi-transparent CPV.
These semi-empirical models can be “built up” in four stages, which can be implemented depending on the resolution of the available environmental data and the number of system parameters that can be defined. The “first pass” model, used for very rough estimations, simply separates the direct and diffuse components, where the direct is converted with a constant efficiency η and the diffuse is transmitted with a constant transmittance τ. This does not account for angle-of-incidence, spectral or thermal effects, but this can be roughly compensated by appropriate definition of the τ and η. The “second pass” model requires solar irradiance data of hourly or greater resolution, including the angle of incidence of the direct component on the plane of array. The power output and light transmittance is calculated for each timestep based on three parameters: η, τ and the maximum tracking angle θtrack, where the electrical power output Pel is given by:
And the transmitted optical power is approximated by
A third-pass model would evaluate the functions η(θ), τ(θ) and τdiffuse (which is simply the appropriately weighted integral of τ(θ)) and evaluate the instantaneous electrical and optical power outputs under a prescribed operation mode [which may optimize electrical power generation (E-mode) or alternate between power generation and maximizing light transmission (MLT-mode)]. To calculate the instantaneous efficiency of the module at each incidence angle stamp, the power factor, as in the value of power relative to the power at 0° incidence angle, is multiplied with the constant efficiency value used in first and second passes. The power factor is extracted from graphs generated by the manufacturer that have been corrected to account for cosine projection. The electric power generated takes into account the ambient temperature effects, in which within the tracking range:
in which
The transmittance is a bit more complicated as it differs between the E-mode and MLT- mode, as shown in Table 2.
To clarify, in E-mode, within the tracking range, none of the beams impinging at the plane of array affect the transmittance as opposed to the diffuse component which is assumed to be transmitted at a constant τ as in the first and second passes of the model. All other values of direct component transmittance are calculated as a function of angle of incidence using graphs generated by the manufacturer. The transmitted power is then the sum of the direct and diffuse transmitted irradiance.
A fourth-pass model would incorporate the effect of temperature T and spectral matching factor S to evaluate the functions η(θ, T, S) and τ(θ, S) (with limited temperature dependence of the optical transmittance assumed). These functions may be predicted by thermal, optical, and electrical modeling, or fit to experimental data as functions of environmental parameters.
Model Results
Studies are currently underway in collaboration with Insolight to conduct detailed optical, thermal, and electric characterization of their semi-transparent THEIA module in Abu Dhabi, UAE which provides a foundation for implementing this model and use it to predict the yearly performance of the module in addition to validate the model for further developments and adaption for other technologies.
The module is oriented towards the south at an optimum tilt angle throughout the year in Abu Dhabi of 20° Figure 6. The test rig accommodates instruments to measure parameters like transmittance, PAR light levels, light intensity, electric power accumulated, and cell and module temperatures at different locations. Readings are taken at a timestamp of 1 min, but the data for this model was averaged per hour to match TMY2 data.
To have an outlook on the generated results, the module was compared to a conventional PV module used in the rooftops in Abu Dhabi and the fixed parameters of both modules are in Table 3. For the PV, values are based on the average range while for the Insolight CPV module, values are either extracted from the manufacturer’s data sheet or established from the experiments carried out between January and April 2022.
The highest stage feasible to implement at this point is the third-pass model. The first and second pass models overestimate the yearly output electric power by 26% and 12.5%, respectively, due to the ignorance of the second pass model with regards to varying efficiency at different angles of incidence and effect of temperature in addition to the independence of the first pass model on the tracking range. The trends in electric power output for the PV and TI-CPV module using the third pass model are displayed in Figure 7.
It can be realized that the production is higher in the CPV but that is compensated in days where the ratio of direct to total POA irradiance is low, as shown in the purely yellow lines between February and April and in November. Hence, the accumulated electric powers per year are generally close at 317.3 kWh/m2 for the CPV Insolight and 309.5 kWh/m2 for the PV module. However, as previously explained, this is not the sole output stream in case of the CPV module in which another output is the electric power avoided due to the daylighting captured by the transmissive module and totals up to 593.9 kWh/m2 yearly. The method for this conversion is detailed in the next section. Figure 8 illustrates the different output streams for both systems in addition to the yearly direct and diffuse components.
Looking at the CPV behaviour more closely, Figure 9 shows how the electric power and transmitted power vary throughout the day at different incidence angles when the module is operating in E-mode. As modelled, the electric power starts and ends accumulation when the incidence angle equals the tracking angle (±55°) and follows the change in POAdirect. As for the transmittance, it is higher outside the tracking range because in this incident, both components of light are transmitted and its dependent on the GHI while during tracking, only the diffuse is allowed through the module. Its important to notice that in the hour before and after tracking, the transmittance continues to increase and falls as soon as tracking initiates, but this behavior cannot be observed in this model due to the hourly resolution.
After the model has been validated and furtherly developed, it can be used to size and design a system utilizing an array of the modules or be adapted for other TI-CPV technologies. At present, however, these models have value for considering the economic prospects for TI-CPV.
Economic Considerations
One thing that has become clear is that future economic value from CPV systems will not come from a (typically modest) boost in electrical output alone, but in the division of the solar resource into multiple output streams (e.g. heat, light, electricity) as recently described by several of the present authors (Apostoleris et al., 2021a). To evaluate the economic potential of these systems requires a slightly more involved computation than the familiar LCOE estimation, although the same underlying equation applies. To illustrate, a semi-transmissive CPV module is considered based on that demonstrated by Insolight, which splits light between an electrical and a transmitted light component. The results of the third-pass model are used implemented expressing the output electric power in kW/m2 of module and the transmitted light in lumens/m2 of module. This procedure indicates a first-year electrical output of 317.3 kWh/m2 and 59.4 million lumen-hours/m2 of module optically. From Figure 8, it can be seen that the optical power is around 2.5 times this value if the module is operating in MLT mode since the full GHI resource is used for transmission.
To evaluate the overall economic potential of this system, it is necessary to assign values to each of the two output streams. The value of the transmitted light depends on how it is utilized. In a building-integrated setting the transmitted light is assumed to be used for daylighting, where standard recommended lighting levels (500–1,000 lux) are an order of magnitude lower than the light transmitted through the module. Over a year, one square meter of module at 20° tilt transmits roughly 63,500,000 lumen-hours under Abu Dhabi weather conditions. If the entirety of this light can be used for daylighting and offsets artificial light, 600–1,200 kWh of lighting energy are avoided per year, if that light would otherwise be provided by LED lamps with 50–100 lumen/W luminous efficacy—exceeding the energy produced by the module itself. The transmitted light, if it can be used to offset artificial lighting, effectively doubles the output of the module if both generated and avoided electricity are counted. Since this light is only available while the sun is shining, it can treated as displacing solar electricity with the same value in c/kWh.
The value of the electrical output is straightforwardly given by the price at which the electricity can be sold—in sunny parts of the world. For this study, the price of electricity was set equal to the LCOE of the PV module using the equation below:
where C is the CapEx in ($/kW), Oy is the operating cost in year y, r is the discount rate, Egen is the electricity generated, and L is the system lifetime.
Setting a CapEx of 920 $/kW to account for EPC (equipment, design, labor, etc.) as per NREL’s benchmark for commercial rooftops PV systems, operating costs scaled up at a 2% of the CapEx yearly, a discount rate equal to the project internal rate of return (∼4%), a lifetime of 25 years, and the power generated by the PV module in the model the LCOE is 4.2 c/kWh which is a reasonable or even conservative value for when-generated solar electricity, as generation costs & contract prices have dropped rapidly in recent years. In previous work (Apostoleris et al., 2021b), it was shown that large-scale photovoltaic installations in the Gulf region can achieve generation costs of 3 c/kWh at capital costs of ∼$1/W and operating costs of $10/kW annual with an IRR of ∼4%.
A generally applicable metric (Apostoleris and Chiesa, 2019) for evaluating the economic potential of an energy (or other infrastructure) investment is the net present value (NPV) taken as the sum of all discounted revenues minus the sum of all discounted costs. As the transmitted light has already been converted into units of electricity consumption avoided, the NPV can be expressed as:
where Pel is the electricity price (assuming the same price for generated and avoided electricity) and Eav is the avoided electricity consumption.
Without deeply considering the specific contexts in which TI-CPV would be deployed, the module cost premium that could be tolerated if all output streams are fully utilized and other costs remain unchanged can be considered. The LCOE now represents the minimum sustainable price of electricity and a worst-case, conserved scenario of 100 lumens/W luminous efficacy (LED) for the electricity avoided by the transmitted light is set. In Figure 10, the NPV under these conditions is displayed as a function of system price, given in USD per m2 of module on the lower x-axis and the equivalent USD/W for a 20% efficient conventional PV module array on the upper x-axis. This rough analysis suggests that a cost premium in excess of $965/m2 could be tolerated under these conditions. If all of this additional cost were concentrated in the module, that would represent a maximum viable module price roughly more than triple that of a conventional Si module. The overall increase in solar resource utilization should be owed to the mixed effect of integrated tracking and the transmissive behaviour of the module.
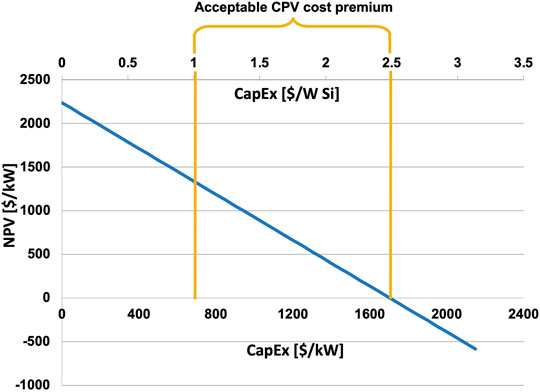
FIGURE 10. Net present value of a TI-CPV system based on the performance model; the cost premium is the increase in CapEx over a conventional ground-mounted system that can be tolerated while still breaking even at current prevailing solar electricity prices.
A full consideration of the costs involved in a more realistic building-integrated application will provide fruitful ground for future study and will help to establish the conditions necessary for novel TI-CPV systems to become viable. The work of Wright et al. (2018) on “pure” TI-CPV without diffuse light utilization offers a strong starting point for such analysis.
It is worth noting that if the transmitted component were instead captured by silicon solar cells and converted with (optimistically) 20% efficiency, the electricity generated would be several times less than the electricity use avoided if the entire transmitted component can be used for daylighting, offsetting electric lights. This is a simple illustration of the power of the “resource splitting” design approach that has advocated previously in our earlier study (Apostoleris and Chiesa, 2020), as opposed to seeking only to maximize electricity generation. While splitting between light and electricity is emphasized here, the same framework can also be applied to photovoltaic-thermal systems that output both electricity and heat, a concept that is again gaining popularity (Widyolar et al., 2020).
Discussion & Outlook
While interesting concepts in tracking integration have proliferated in recent years, mechanical micro-tracking has seen the most advanced demonstration and has made the most progress towards commercialization. CPV systems adopting integrated tracking and diffuse collection are able to provide multiple output streams in conjunction with electricity like daylight and heat simultaneously.
A number of technical challenges must be addressed in order to realize commercially viable TI-CPV modules. The concept at which the tracking motion is triggered needs to be reliable and prompt since it affects the overall performance. Predominantly in small cells, any minor misalignment or a lagging reaction to the point of array’s incident angle will waste the potential of irradiance accumulation.
Therefore, one design feature that requires more focus is the alignment error between the cell array and the lenses. A study by study by José et al. (2020) explains a method to characterize the misalignments for micro-CPVs and quantify them in metric values as distance between centres. The process starts with capturing a magnified image of the receiver with a camera followed by pre-processing the image like increasing its contrast. Then, the images are segmented on MATLAB using Superpixel Oversegmentation and Fast Marching Method. Finally, Hough Transform helps realise the circles’ pixels. Moreover, depending on the acceptance angle limits, the rate at which trackers respond to the change in incidence angle and actuate has a considerable effect on the power production yield and heating of the cell.
Programmable techniques in micro-CPVs are promising towards preventing these mishaps. One technique is calculating the incidence angle at each timestamp based on the geographical location, date, and tilt angle then establishing the tracking movement accordingly when the incidence angle lies within the tracking range. In addition to that, another concept is predicting the maximum power production at each timestamp and acquiring the exact coordinates of the cells relative to the optics needed to realize that power. The limitation to this approach is that the tracking process will cease to function as expected in an event of a disrupted load cycle, as experienced in the experimental characterization of the Insolight module. The different tracking modes available can be efficiently used to serve different applications. While it might seem unwise to acquire a CPV module to operate it in MLT-mode (Maximum Light Transmission), an optimized switching behaviour between it and E-mode (electrical generation) can help in regulating the transmitted light in agriculture and daylighting applications or keeping it constant throughout the day albeit the incidence angle. In the case of low direct light irradiance, contrary to typical CPV modules with no silicon cells in the back-pane, the solar resource would still be utilized for transmission. In agriculture, some plants require a certain level of instantaneous PAR (Photosynthetically Active Radiation) or a constant total accumulated level daily to flourish. In daylighting, it would be preferable to maintain a regulated level of light in lumens/m2 at all times of the day when sunlight is available. In both applications, it’s worth noting that this switching mechanism also has an effect on the temperature within.
The location and application of the TI-CPV module need to be taken into consideration during the manufacturing and material selection process. From “big league” components like the top glass pane to “small fry” components like the glue between the lenses in an array, the environmental conditions like temperature, sand, and humidity need to be taken into consideration. The glue between the lenses should withstand the ambient temperature or else its melting behaviour would cause disintegration of the lens array or clinging of sand and dust which would ultimately decrease the aperture area. Regarding the top pane, a flat glass surface would protect the optics from soiling and provide an undemanding cleaning routine, considering that it should not largely affect the intensity of transmitted irradiance. Another undermined parameter that could facilitate the commercialization of the modules is the weight of the module frame, especially in roofing greenhouses and buildings.
A particular challenge has been the trade-off between the concentration factor and the tracking range. Actually realized TI-CPV systems have simultaneously achieved tracking angles in excess of ±50° with concentration factors around ×200. However, this concentration is roughly ×2 lower than what is needed to make III-V multijunction cells, which are required to achieve the performance assumed in the preceding analyses, economically feasible to use. While continued research & development to boost the performance of tracking-integrated optical systems continues, advances in multijunction cell production—such as a breakthrough in the long-sought goal of III-V wafer reuse, or the development of a stable, high-efficiency perovskite-on-silicon multijunction—could represent a critical development that would transform the economics of TI-CPV.
Performance parameters depend on the application as well as the location of the TI-CPV system, whereas for a greenhouse application in a country with harsh climates, the savings in cooling load prevail the sizing requirements. In countries where field space serves as the main constraint, TI-CPV systems not only save space by the elimination of tracker hardware but also by co-serving power production and agricultural or building needs.
To compete with mature solar technologies, TI-CPV technology must be able to follow the same economic trajectory as these established technologies. An economically promising TI-CPV design should have a clear path to reduce costs via industrial scaling—that is, it should avoid relying on high-cost components that would set a high floor for future manufacturing costs—and should have a clear area of application where it can add value in the near term. A deeper investigation into improving the manufacturability of TI-CPV systems, extending beyond the mechanical systems being commercially developed today to non-mechanical sun-tracking and beam-steering systems such as those surveyed in the literature review presented here, could represent a fruitful area for future study. This survey has attempted to highlight promising areas of development that satisfy these two conditions and offer potential pathways to finally realizing the promise of high CPV power densities by the industrial adoption of TI-CPV.
Author Contributions
KY prepared the literature survey with guidance of HA. HA, KY, and MB carried out performance & economic study with preliminary results needed for the model taken from an experiment conducted by MB. AG and MC provided project mangement & guidance
Conflict of Interest
The authors declare that the research was conducted in the absence of any commercial or financial relationships that could be construed as a potential conflict of interest.
Publisher’s Note
All claims expressed in this article are solely those of the authors and do not necessarily represent those of their affiliated organizations, or those of the publisher, the editors and the reviewers. Any product that may be evaluated in this article, or claim that may be made by its manufacturer, is not guaranteed or endorsed by the publisher.
References
Alves, M., Pérez-Rodríguez, A., Dale, P. J., Domínguez, C., and Sadewasser, S. (2019). Thin-Film Micro-Concentrator Solar Cells. J. Phys. Energy 2, 012001. doi:10.1088/2515-7655/ab4289
Aposoleris, H., and Chiesa, M. (2019). “The Role of Financing in Realizing Ultra-low Solar Electricity Prices in the Middle East,” in 2019 IEEE 46th Photovoltaic Specialists Conference (PVSC), Chicago, IL, June 16–21, 2019 (IEEE). doi:10.1109/PVSC40753.2019.8980633
Apostoleris, H., Al Ghaferi, A., and Chiesa, M. (2021b). What Is Going on with Middle Eastern Solar Prices, and what Does it Mean for the Rest of Us? Prog. Photovolt. Res. Appl. 29, 638–648. doi:10.1002/pip.3414
Apostoleris, H., and Chiesa, M. (2019). “High-concentration Photovoltaics for Dual-Use with Agriculture,” in AIP Conference Proceedings 2149, Chicago, IL, June 16–21, 2019, 050002. doi:10.1063/1.5124187
Apostoleris, H., and Chiesa, M. (2020). “Solar Concentration, Solar Resource Utilization, and Sustainability,” in Nonimaging Optics: Efficient Design for Illumination and Solar Concentration XVII 11495, San Francisco, CA, February 1–6, 2020 (SPIE). doi:10.1117/12.2569051
Apostoleris, H., Sgouridis, S., Stefancich, M., and Chiesa, M. (2019a). Utility Solar Prices Will Continue to Drop All over the World Even without Subsidies. Nat. Energy 4 (10), 833–834. doi:10.1038/s41560-019-0481-4
Apostoleris, H., Stefancich, M., and Chiesa, M. (2018). Concentrating Photovoltaics (CPV): The Path Ahead. Cham: Springer International Publishing.
Apostoleris, H., Stefancich, M., and Chiesa, M. (2021a). The CPV "Toolbox": New Approaches to Maximizing Solar Resource Utilization with Application-Oriented Concentrator Photovoltaics. Energies 14, 795. doi:10.3390/en14040795
Apostoleris, H., Stefancich, M., and Chiesa, M. (2016). Tracking-integrated Systems for Concentrating Photovoltaics. Nat. Energy 1, 18. doi:10.1038/nenergy.2016.18
Apostoleris, H., Wheeler, D., Shen, Y., Cooper, T. A., Boriskina, S., and Chiesa, M. (2019b). “A Solar Concentrator Based on Photonic Angular Selectivity,” in AIP Conference Proceedings, Fes, Morocco, June 25–27, 2019 (AIP Publishing LLC). doi:10.1063/1.5124200
Askins, S., Jost, N., Aguilar, F., Anglade, L., Nardin, G., Duchemin, M., et al. (2019). “Performance of Hybrid Micro-concentrator Module with Integrated Planar Tracking and Diffuse Light Collection,” in 2019 IEEE 46th Photovoltaic Specialists Conference (PVSC), Chicago, IL, June 16–21, 2019 (IEEE). doi:10.1109/pvsc40753.2019.8980519
Chen, G., Wang, Y., Jiang, D., Wang, H., Wang, K., Tan, J., et al. (2021). A Programmable Compound Prism Powered by Triboelectric Nanogenerator for Highly Efficient Solar Beam Steering. Nano Energy 80, 105524. doi:10.1016/j.nanoen.2020.105524
Domínguez, C., Jost, N., Askins, S., Victoria, M., and Antón, I. (2017). “A Review of the Promises and Challenges of Micro-concentrator Photovoltaics,” in 13th International Conference on Concentrator Photovoltaic Systems (CPV-13), Ottawa, Canada, May 1–3, 2017 (AIP Conference Proceedings 1881), 080003. doi:10.1063/1.5001441
Evke, E. E., Huang, C., Wu, Y.-W., Arwashan, M., Lee, B., Forrest, S. R., et al. (2020). Kirigami‐Based Compliant Mechanism for Multiaxis Optical Tracking and Energy‐Harvesting Applications. Adv. Eng. Mat. 23, 2001079. doi:10.1002/adem.202001079
Grede, A., Brulo, G., Ren, A., Lenko, M., Rahn, C., and Giebink, N. (2018). “Motorless Microtracking for Rooftop CPV,” in (2018 IEEE 7th World Conference on Photovoltaic Energy Conversion, WCPEC 2018), Waikoloa, HI, June 10–15, 2018 (IEEE). doi:10.1109/pvsc.2018.8547714
Grede, A. J., Price, J. S., and Giebink, N. C. (2016). Fundamental and Practical Limits of Planar Tracking Solar Concentrators. Opt. Express 24, A1635. doi:10.1364/oe.24.0a1635
Green, M. A. (2019). How Did Solar Cells Get So Cheap? Joule 3, 631–633. doi:10.1016/j.joule.2019.02.010
Hallas, J. M., Karp, J. H., Tremblay, E. J., and Ford, J. E. (2010). “Lateral Translation Micro-tracking of Planar Micro-optic Solar Concentrator,” in Proc. SPIE 7769, High and Low Concentrator Systems for Solar Electric Applications, San Diego, CA, 24 August 2010 (SPIE), 7769. doi:10.1117/12.860980
Haney, M., Gu, T., and Agrawal, G. (20142014). “Hybrid Micro-scale CPV/PV Architecture,” in IEEE 40th Photovoltaic Specialist Conference (PVSC), Denver, CO, June 8–13, 2014 (IEEE), 2122–2126. doi:10.1109/pvsc.2014.6925343
Hirai, D., Okamoto, K., and Yamada, N. (2015). “Fabrication of Highly Transparent Concentrator Photovoltaic Module for Efficient Dual Land Use in Middle DNI Region,” in 2015 IEEE 42nd Photovoltaic Specialist Conference (PVSC), New Orleans, LA, June 14–19, 2015 (IEEE). doi:10.1109/pvsc.2015.7355759
Ito, A., Sato, D., and Yamada, N. (2018). Optical Design and Demonstration of Microtracking CPV Module with Bi-convex Aspheric Lens Array. Opt. Express 26, A879. doi:10.1364/oe.26.00a879
Johnsen, H. J. D., Aksnes, A., and Torgersen, J. (2020). High-performance Stationary Solar Tracking through Multi-Objective Optimization of Beam-Steering Lens Arrays. Opt. Express 28, 20503. doi:10.1364/oe.396477
Johnsen, H. J. D., Torgersen, J., and Aksnes, A. (2019). High-Concentration Wide-Angle Tracking Integration with Stacked Lens Arrays. AIP Conf. Proc. 2149, 1–6. doi:10.1063/1.5124204
José, L. S., Vallerotto, G., Herrero, R., and Antón, I. (2020). Misalignments Characterization for Micro-CPV Modules. AIP Conf. Proc. 2298, 1–6. doi:10.1063/5.0032292
Khan, I., Castelletto, S., and Rosengarten, G. (2020). A Novel Hexagonal Beam Steering Electrowetting Device for Solar Energy Concentration. Micromachines 11, 1016. doi:10.3390/mi11111016
Khan, I., Castelletto, S., and Rosengarten, G. (2017). Fabrication of Solar Beam Steering Electrowetting Devices-Present Status and Future Prospects. J. Phys. D. Appl. Phys. 50, 403001. doi:10.1088/1361-6463/aa84c5
Lee, K., Chien, C.-W., Lee, B., Lamoureux, A., Shlian, M., Shtein, M., et al. (2016a). Origami Solar-Tracking Concentrator Array for Planar Photovoltaics. ACS Photonics 3, 2134–2140. doi:10.1021/acsphotonics.6b00592
Lee, K., Yao, Y., He, J., Fisher, B., Sheng, X., Lumb, M., et al. (2016b). Concentrator Photovoltaic Module Architectures with Capabilities for Capture and Conversion of Full Global Solar Radiation. Proc. Natl. Acad. Sci. U.S.A. 113, E8210–E8218. doi:10.1073/pnas.1617391113
Lee, T., Kim, J., Cho, S., Pyo, S., Song, K., and Lee, J. (2019). Planar‐type Concentrating Photovoltaics with Cylindrical Lenses Directly Integrated with Thin Flexible GaAs Solar Cells. Prog. Photovolt. Res. Appl. 28, 71–78. doi:10.1002/pip.3209
Li, D., Li, L., Jared, B., Keeler, G., Miller, B., Wood, M., et al. (2018). Wafer Integrated Micro‐scale Concentrating Photovoltaics. Prog. Photovolt. Res. Appl. 26, 651–658. doi:10.1002/pip.3034
Li, Q., Zheng, C., Shirazi, A., Bany Mousa, O., Moscia, F., Scott, J. A., et al. (2017). Design and Analysis of a Medium-Temperature, Concentrated Solar Thermal Collector for Air-Conditioning Applications. Appl. Energy 190, 1159–1173. doi:10.1016/j.apenergy.2017.01.040
Lim, T., Kwak, P., Song, K., Kim, N., and Lee, J. (2016). Automated Dual-Axis Planar Solar Tracker with Controllable Vertical Displacement for Concentrating Solar Microcell Arrays. Prog. Photovolt. Res. Appl. 25, 123–131. doi:10.1002/pip.2843
Martínez, J. F., Steiner, M., Wiesenfarth, M., Siefer, G., Glunz, S. W., and Dimroth, F. (2021). Power Rating Procedure of Hybrid Concentrator/Flat‐Plate Photovoltaic Bifacial Modules. Prog. Photovolt. Res. Appl. 29 (6), 614–629. doi:10.1002/pip.3410
Nakatani, M., and Yamada, N. (2018). “Characterization of Core-Shell Spherical Lens for Microtracking Concentrator Photovoltaic System,” in (Photovoltaic Specialists Conference (PVSC) 2019 IEEE 46th), Chicago, IL, June 16–21, 2019 (PVSC). doi:10.1109/PVSC.2018.8547953
Nakatani, M., and Yamada, N. (2019). Characterization of Core-Shell Spherical Lens for Microtracking Concentrator Photovoltaic System. Energies 12, 3517. doi:10.3390/en12183517
Nardin, G., Domínguez, C., Aguilar, Á. F., Anglade, L., Duchemin, M., Schuppisser, D., et al. (2020). Industrialization of Hybrid Si/III-V and Translucent Planar Micro‐Tracking Modules. Prog. Photovolt. Res. Appl. 29, 819–834. doi:10.1002/pip.3387
Ong, S., Campbell, C., Denholm, P., Margolis, R., and Heath, G. (2013). Land-Use Requirements for Solar Power Plants in the United States. Golden, CO: National Renewable Energy Laboratory.
Paap, S., Gupta, V., Tauke-Pedretti, A., Resnick, P., Sanchez, C., Nielson, G., et al. (2014). “Cost Analysis of Flat-Plate Concentrators Employing Microscale Photovoltaic Cells for High Energy Per Unit Area Applications,” in 2014 IEEE 40th Photovoltaic Specialist Conference (PVSC), Denver, CO, June 8–13, 2014 (IEEE). doi:10.1109/pvsc.2014.6925544
Pender, J. (2005). Motion-Free Tracking Solar Concentrator. Patent issued in the united states US6958868B1.
Price, J., Grede, A., Wang, B., Lipski, M., Fisher, B., Lee, K., et al. (2017). High-concentration Planar Microtracking Photovoltaic System Exceeding 30% Efficiency. Nat. Energy 2, 113. doi:10.1038/nenergy.2017.113
Sato, D., Lee, K.-H., Araki, K., Yamaguchi, M., and Yamada, N. (2019). Design and Evaluation of a III-V/Si Partial CPV Module for Maximization of Power Generation Per Unit Module Area. IEEE J. Photovolt. 9, 147–153. doi:10.1109/jphotov.2018.2877015
Swanson, R. M. (2000). The Promise of Concentrators. Prog. Photovolt. Res. Appl. 8, 93–111. doi:10.1002/(sici)1099-159x(200001/02)8:1<93:aid-pip303>3.0.co;2-s
Teng, T.-C., Kuo, C.-H., and Li, Y.-J. (2020). Planar Solar Concentrator Composed of Stacked Waveguides with Arc-Segment Structures and Movable Receiving Assemblies. Opt. Express 28, 34362. doi:10.1364/oe.405909
Wang, W., Li, C., Rodrigue, H., Yuan, F., Han, M.-W., Cho, M., et al. (2017). Kirigami/Origami-Based Soft Deployable Reflector for Optical Beam Steering. Adv. Funct. Mat. 27, 1604214. doi:10.1002/adfm.201604214
Widyolar, B., Jiang, L., Brinkley, J., Hota, S. K., Ferry, J., Diaz, G., et al. (2020). Experimental Performance of an Ultra-low-cost Solar Photovoltaic-Thermal (PVT) Collector Using Aluminum Minichannels and Nonimaging Optics. Appl. Energy 268, 114894. doi:10.1016/j.apenergy.2020.114894
Wright, D. J., Badruddin, S., and Robertson-Gillis, C. (2018). Micro-Tracked CPV Can Be Cost Competitive with PV in Behind-The-Meter Applications with Demand Charges. Front. Energy Res. 6, 97. doi:10.3389/fenrg.2018.00097
Yamada, N., Ijiro, T., Goto, W., Okamoto, K., Dobashi, K., and Shiobara, T. (2013). “Development of Silicone-Encapsulated CPV Module Based on LED Package Technology,” in 2013 IEEE 39th Photovoltaic Specialists Conference (PVSC), Tampa, FL, June 16–21, 2013 (IEEE). doi:10.1109/PVSC.2013.6744197
Keywords: tracking integration, beam steering, building integrated photo voltaic (BIPV), solar energy economics, concentrator photovoltaics (CPV), PV system modeling, agricultural photovoltaics, micro-CPV
Citation: Younes K, Apostoleris H, Bin Saad M, Al Ghaferi A and Chiesa M (2022) Realizing High Photovoltaic Power Densities With Tracking-Integrated Concentrator Photovoltaics. Front. Energy Res. 10:842201. doi: 10.3389/fenrg.2022.842201
Received: 23 December 2021; Accepted: 09 May 2022;
Published: 08 June 2022.
Edited by:
Mingke Hu, University of Nottingham, United KingdomReviewed by:
Muhammad Wakil Shahzad, Northumbria University, United KingdomDaniel Tudor Cotfas, Transilvania University of Brașov, Romania
Copyright © 2022 Younes, Apostoleris, Bin Saad, Al Ghaferi and Chiesa. This is an open-access article distributed under the terms of the Creative Commons Attribution License (CC BY). The use, distribution or reproduction in other forums is permitted, provided the original author(s) and the copyright owner(s) are credited and that the original publication in this journal is cited, in accordance with accepted academic practice. No use, distribution or reproduction is permitted which does not comply with these terms.
*Correspondence: Matteo Chiesa, bWF0dGVvLmNoaWVzYUBrdS5hYy5hZQ==