- Department of Microbiology, Centre for Microbial and Fermentation Technology, University College of Science, Osmania University, Hyderabad, India
A comprehensive study was carried out to assess the efficiency of different chemicals on the pretreatment of plant biomass, corn cobs. The efficiency was assessed based on its effect on subsequent hydrolysis of biomass for the conversion to fermentable sugars. Both alkali- and acid-based pretreatments were carried out under optimized conditions to avoid the formation of inhibitors and also to yield more sugars. Among the different chemicals used, 2% NaOH (w/v) was found to be effective with the delignification efficiency of 84.32%, and furthermore, the biomass saccharification efficiency was found to be 86.28% using 1.20% (v/v) HNO3 at 100°C for 90 min, whereas the pretreatment with other chemicals such as KOH, NaClO2, Na2SO3, and NH3 had significantly (p < 0.05) low delignification, which ultimately reduced the saccharification efficiency. In acid–base-based pretreatment, 1.5% (v/v) HNO3 resulted in 63.42% delignification along with 68% digestion of hemicelluloses. The effect of pretreatment efficiency on digestibility was investigated by biomass characterization using SEM, FTIR, and XRD analyses before and after the treatment process. The imaging studies clearly indicated that 2% NaOH efficiently digested the complex matrix alignment and enhanced the surface area of the carbohydrate polymer which had shown a positive impact on the rate of hydrolysis. The study concludes that alkali-based pretreatment is the efficient and cost-viable technique for the effective valorization of corn cobs for the production of fermentable sugars which can be further converted to various valuable bioproducts.
Introduction
With the rapid increase in population and industrialization, there has been an alarming rise in energy demand. This not only led to the depletion of fossil reserves but also had irrevocable climate change, which is a serious global issue of concern. The only option left is to transit toward renewable and sustainable energy resources that can gradually decrease the reliance on petroleum-based fuels. Plant biomass is one of the most abundant, renewable, and organic sources that can be utilized for sustainable bioethanol production (Sunkar et al., 2020). Different types of agricultural wastes are available and have been used so far which have different ratios of chemical composition with cellulose, hemicellulose, and lignin. Choosing the best feedstock for the production of value-added products is critically important (Soltani et al., 2015). Selecting a substrate that is abundantly available throughout the season is also an important point to consider for the continuous production of bioproducts. Among the various agricultural feedstocks available, corn crop produces an annual biomass of 4 tons per acre land and are estimated to obtain 1 kg of biomass with 1 kg of grain (Kim and Dale, 2004).
Although utilization of biomass for biofuel production is not a novel idea, the challenges related to biomass digestibility, total sugar extraction, and the release of inhibitors during the process and detoxification issues still exist. The main hurdle in the biomass conversion process is its recalcitrance to access for digestion (Baruah et al., 2018). Different lignocellulosic feedstocks have different chemical composition and polymerization, and therefore, it requires specific chemicals that aid in the digestion process. Different pretreatment methods have been adopted for different biomasses owing to their compositional diversity. Primarily, pretreatment methods are characterized into physical, chemical, biological, or a combination of two based on the substrate used for the conversion process. Among the aforementioned methods, chemical pretreatment methods are well developed and in practice with various biomasses due to their effective yield (Zhu et al., 2015).
Cellulose, hemicellulose, and lignin are tightly packed in biomass, leaving very small pores, which limit digestion. Pretreatment is a process that removes the packed lignin with holocellulose, thereby increasing the pores in the biomass, which aids in digestion. The type of pretreatment method chosen and the reaction conditions adopted will decide the rate of hydrolysis and yield of fermentable sugars (Brodin et al., 2017). The pretreatment efficiency will have a major influence and effect on downstream hydrolysis, consequently the sugar recovery and ultimately bioethanol yield (Hernandez-Mendoza et al., 2021).
On a general note, the pretreatment process takes above 20% of the total production cost, which is a quite high energy-consuming step in the bioconversion process (Seidl and Goulart, 2016). The use of dilute acids is the most common pretreatment methods, but it requires corrosion-resistant equipment, and the process unavoidably results in impeding chemical compounds like furfurals and various phenolic compounds, which hamper the fermentation process, whereas in the alkali-based pretreatment process, sodium, potassium, calcium, or ammonium hydroxides are employed at standard temperature and reaction time for the delignification process. Among them, sodium hydroxide is reported to be more efficient and helps remove uronic and acetyl groups from hemicellulose, which helps in the digestion of hemicelluloses (Chang and Holtzapple, 2000). A combination of physicochemical pretreatment methods such as the application of steam explosion with KOH for the pretreatment of rice straw has also been studied for the substantial increase in the yield of fermentable sugars (Banoth et al., 2017). In order to assess the efficacy of various chemicals for the pretreatment of lignocellulosic biomass, the yield of fermentable sugars obtained from various biomasses is compared.
To evaluate and confirm the efficiency of various chemicals for pretreatment, both physical and chemical changes that take place during the process must be studied. The reaction conditions and the reaction time must be carefully optimized such that there should be maximum removal of lignin with minimum sugar loss that helps in the sustainability of the pretreatment method (Sunkar et al., 2020). Determining the biomass characteristics often requires a variety of analytical techniques that help in the validation of pretreatment. The most commonly used techniques are scanning electron microscopy (SEM), X-ray crystallography (XRD), and Fourier transform infrared (FTIR), which helps in interpreting the potential of biomass pretreatment on the rate of hydrolysis.
The present study aimed at revealing the significance of pretreatment on the rate of hydrolysis of corn cob residues. Various acid-based chemicals including nitric acid, sulfuric acid, hydrochloric acid, and phosphoric acid, and alkali-based chemicals including sodium hydroxide, potassium hydroxide, sodium chlorite, sodium sulfite, and aqueous ammonia solution have been used for the pretreatment of corn cob and evaluated their potential in rate of hydrolysis based on the level of fermentable sugars produced. Thus, the efficiency of pretreatment was correlated with the biomass digestibility and efficiency of saccharification. The effect of different chemical pretreatments on morphological, physical, and chemical changes was analyzed using the SEM, XRD, and FTIR methods. The effect of pretreatment was justified by the SEM images, and delignification was clearly observed in FTIR spectra.
Materials and Methods
Corn Cob Collection and Processing
Corn cobs were selected as a carbon source for the production of fermentable sugars in the present study. The corn cobs were collected from nearby farms, around Hyderabad city, Telangana State, India. The cobs were initially Sun-dried and mechanically milled to reduce the particle size. Then, the residue was sieved using a 10-mesh screen (2.00 mm) and oven-dried at 60°C to remove the leftover moisture and to acquire constant weight, and then stored in clean dry polythene bags at room temperature until further analysis. All the chemicals used for the analysis are of analytical grade obtained from HiMedia and Merck. All the pretreatment and hydrolysis experiments were performed on a dry weight basis.
Chemical Composition Analysis
The main components of biomass such as cellulose, hemicellulose, and lignin were chemically analyzed by standard TAPPI methodology (TAPPI Technical Association of Pulp and Paper Institute, 1992).
Alkali-Based Pretreatment of Corn Cob
The powder of corn cobs was pretreated using different alkali-based chemicals including NaOH, KOH, NaClO2, and Na2SO3. The concentrations of different chemicals used were 0.5, 1.0, 1.5, 2.0, 2.5, and 3.0% (on mass basis). Five grams of the ground substrate was taken in a 250-ml Erlenmeyer flask and added to each chemical at a solid-to-liquid ratio of 1:10, and the flasks were incubated overnight at 65°C with an rpm set to 120 in a rotary shaker incubator. The conditions were chosen based on our previous studies (Sunkar and Bhukya, 2021). In addition to this, a flask with only water was taken, instead of a chemical, and used as a control for the comparison. After incubation, all the five flasks were taken out of the incubator, and the reaction mixture was filtered through muslin cloth, followed by filtration using a Whatman no. 1 filter paper, and the obtained filtrate was analyzed for lignin. The left over residue was thoroughly washed under a running tap water until it gets neutral pH, dried in an oven at 60°C overnight, and analyzed for the main components in biomass for the validation of pretreatment efficiency.
Aqueous Ammonia Pretreatment of Corn Cobs
The raw corn cob powder was treated with aqueous ammonia at different concentrations ranging from 10, 15, 20, 25 to 30%. For the reaction set up, 5 g of raw corn cob was taken and added at a specific concentration of aqueous ammonia at a solid-to-liquid ratio of 1:10, and the flasks were incubated overnight at 65°C. For comparison, a flask with water and without chemicals was kept for incubation as control. After incubation, all the flasks were brought to room temperature and filtered by through the Whatman no. 1 filter paper, and the obtained filtrate was used for the analysis of phenols for the evaluation of delignification.
Acid-Based Pretreatment of Corn Cobs
Along with bases, acids were also used in the present study to understand their efficiency in the delignification of biomass. In the present study, four acids were used for the pretreatment process including HNO3, H2SO4, HCl, and H3PO4 at four different concentrations (0.5, 1.0, 1.5, and 2.0%). Five grams of the milled and sieved substrate were taken in a 250-ml Erlenmeyer flask, added to each dilute acid at a solid-to-liquid ratio of 1:10 at specific concentrations, and incubated at 100°C for 120 min in a boiling water bath. Along with the aforementioned mixture, an additional flask containing water at 1:10 ratio, instead of acid, was used as a control for comparison. After precise incubation, all the flasks were removed from the water bath, cooled at room temperature, and filtered through a Whatman no. 1 filter paper. The obtained filtrate was used for the analysis of phenolic compounds along with sugars. The leftover residue was washed under a running tap and oven-dried overnight at 60°C, and then the chemical composition was analyzed and used to validate the efficiency of pretreatment on the further hydrolysis step.
Dilute Acid Hydrolysis
The alkali-pretreated solid residue was subjected to saccharification using nitric acid as the hydrolyzing solvent (Sunkar and Bhukya, 2021). In brief, 5 g each of differently pretreated corn cob substrates were taken in a 250-ml Erlenmeyer flask and added to 1.5% nitric acid at a solid-to-liquid ratio of 1:10 and incubated in a boiling water bath for 120 min, and at every 30-min interval, 2 ml sample was collected in a sterile Eppendorf tube and analyzed for sugars produced in the reaction mixture. After 120 min of reaction time, total biomass was filtered through muslin cloth followed by a Whatman no. 1 filter paper, and the residue was washed thoroughly to remove the acid bound to biomass and dried overnight in an oven at 60°C. The dried biomass was used for the extraction of glucose using enzyme hydrolysis.
Enzyme Hydrolysis
The leftover residues from all the nine chemical pretreatments were subjected to enzyme saccharification. The efficiency of each chemical pretreatment on hydrolysability was validated based on the fermentable sugars produced. In the present study, 5 g of the solid leftover fraction was taken in a 250-ml Erlenmeyer flask and was added to commercial cellulose (60 FPU/ml activity) obtained from Sigma-Aldrich, United States (C8546, P Code. 1001000381). The activity of the enzyme was calculated as per the standard protocol, recommended in the Commission on Biotechnology, IUPAC (Ghose, 1987). The enzyme load was kept constant at 30U/gds based on our previous study (Sunkar and Bhukya, 2021), with a solid-to-liquid ratio of 1:10 using 0.05 M acetate buffer (pH 5.0). To the reaction mixture, 0.2% (on a weight basis) sodium azide was added to prevent any bacterial contamination. The enzyme substrate mixture was incubated at 50°C in a rotary shaker incubator for 72 h at 120 rpm. Subsequently, 2 ml of sample was collected at every 12-h interval in a sterile Eppendorf tube and centrifuged at 10,000 rpm for 10 min, and the filtrate thus obtained was analyzed for the glucose recovered.
Biomass Characterization
The impact of different treatments on biomass both physically and chemically were analyzed by SEM, XRD, and FTIR analyses.
Scanning Electron Microscopy
An imaging study was performed to visualize the changes in the surface physical properties and microstructures of raw corn cob and residue left after different treatments. Microscopy was performed using a Zeiss scanning electron microscope, with an acceleration voltage maintaining 10 kV. The processed samples were mounted over the stubs with a double-sided carbon conductive tape, and a thin layer of gold coat over the samples was carried out by using an automated sputter coater (Model-JEOL JFC-1600) for 3 min and scanned using a scanning electron microscope (JoEl-JSM 5600) at the required magnifications as per the standard procedure (Bozzola et al., 1998).
X-Ray Diffraction Studies of Corn Cob Residue
The crystal nature of differently treated corn cobs along with raw cobs powder was analyzed using an X-ray diffractometer (Philips, X’Pert Pro). The samples were scanned using a Cu X-ray tube, with a voltage maintained at 40 kV and 30 mA current. The divergence and scatter slits were used with 1° each, with a receiving slit of 0.3 mm. The scanning was performed with a 2 theta drive axis with a scan range of 10–80° in a continuous mode with a speed of 4.0°/min.
Fourier Transform Infrared Analysis
Raw- and different chemicals-treated corn cobs were investigated for the chemical changes using FTIR analysis. For this, the raw, pretreated, and hydrolyzed corn cob residues were used for analysis. The biomass samples were mixed with KBr of 200 mg concentration in a ratio of 100:1. The substrate is ground to powder and pelleted using the press. The sample pellets were then scanned by Fourier transform infrared spectroscopy, with a wavelength range of 500–4,000 cm−1.
Analytical Methods
Estimation of Sugars
The total reducing sugars in the acid and enzyme hydrolysates were determined by using the dinitrosalicylic acid (DNS) method (Miller, 1959). Furthermore, the sugar analysis was carried out by the high-pressure liquid chromatography (HPLC) method as described by Gupta et al. (2012). Briefly, the HPLC (Shimadzu, Kyoto, Japan) system was equipped with an RI detector, monosaccharide-zodiac Carb 87C column (300 × 7.8 mm), LC-20AD PUMP-A, LC-20AD PUMP-B, DGU-20A3 degasser, and LC solutions software that was used for this study. The column temperature was set at 30°C, and the flow rate was adjusted to 0.6 ml/min. HPLC-grade water was used as the solvent after filtration and degassing was performed by an inline degasser. For each sample, 15-min run time was carried out, followed by the column equilibration with HPLC-grade water, the peaks were detected at 210 nm, and the results were expressed as equivalent to xylose and glucose (Sigma-Aldrich).
All the experiments were carried out in triplicates, and the mean values are presented with standard error. The data were analyzed using SPSS version 20.0 software, and the p value < 0.05 was considered a significant difference.
Results
Chemical Composition of Corn Cobs
The chemical composition was analyzed and mentioned in our previous study as follows, 34.7, 33.8, and 18.4% with respect to cellulose, hemicellulose, and lignin. The chemical composition of raw, pretreated, and hydrolyzed biomass is listed in Table 1.
Delignification Rate of Alkali-Based Pretreatment of Corn Cobs
The effect of alkali-based chemicals on the rate of delignification is described in Figure 1. In the present study, among all the four chemicals used for the delignification, 2% NaOH incubated overnight at 65°C had resulted in maximum delignification of 84.32 ± 2.57%, which is significantly high compared to the other chemicals used in the study. The other three chemicals such as KOH, NaClO2, and Na2SO3 resulted in 72.37 ± 3.11, 62.14 ± 2.47, and 62.61 ± 1.2% corresponding to 2.0, 2.5, and 2.5% concentrations, respectively (Figure 1).
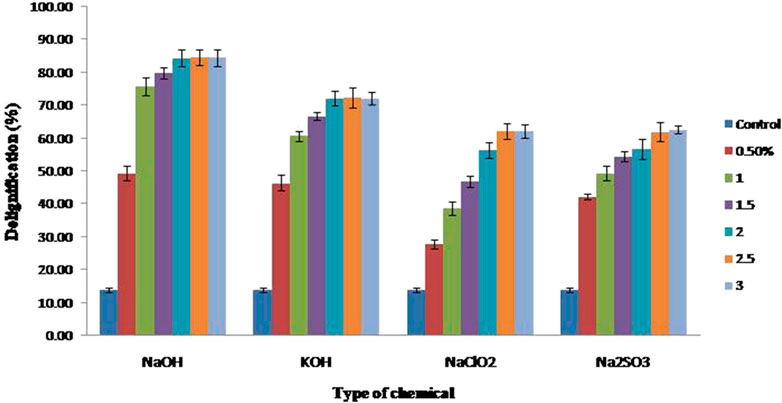
FIGURE 1. Effect of different alkali-based chemicals on delignification of corn cobs with respect to different concentrations.
The concentration of chemicals was directly related to the rate of delignification up to some level and thereafter had no effect was observed. Compared to our previous data (Sunkar and Bhukya, 2021), a rise in temperature and the reaction time increased the percentage of delignification, suggesting the importance of critical factors that significantly affect the delignification efficiency, which thereby affects the overall yield.
Delignification Using Aqueous Ammonia
Aqueous ammonia was used in the present study for the evaluation of delignification efficiency due to its cost effectiveness. The rate of delignification with respect to ammonia concentration is depicted in Figure 2. From the data, it is found that with the increase in concentration from 10 to 20%, a 50% increase in delignification was observed, and from 20 to 30%, there was not much difference noted. Maximum delignification was obtained at 25% concentration with 66.22 ± 3.09%, and at 30% concentration, the percentage of delignification was observed to be 66.09 ± 1.8.
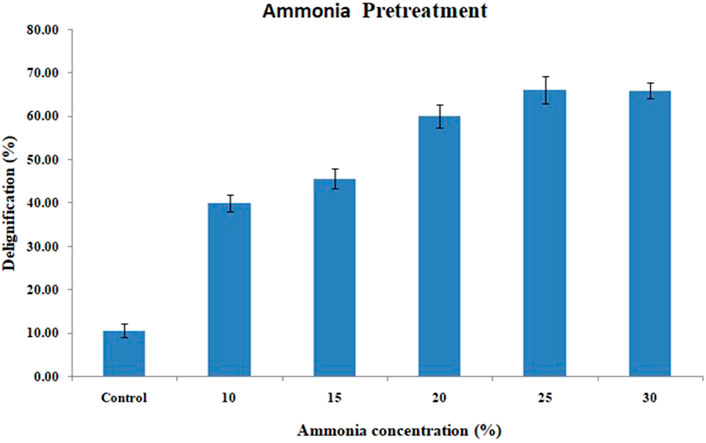
FIGURE 2. Effect of different concentrations of aqueous ammonia on delignification of the corn cob residue.
Delignification Using Dilute Acids
In order to evaluate and compare the alkali-based pretreatment with dilute acid pretreatment, corn cobs were delignified using four acids, and among them, HNO3 at 1.5% resulted in 63.42 ± 3.13% delignification along with 68% digestion of hemicellulose without showing the notable effect on the cellulose content, whereas the rest of the three acids such as H2SO4, HCl, and H3PO4 resulted in a maximum delignification of 57.89, 41.06, and 54.08%, respectively. Due to the acidic nature of chemicals, it led to hemicellulose digestion as well with the efficiency of 72.12, 49.98, and 41.18%, which is significantly high (p < 0.05) when compared to the control that resulted in 8.9% saccharification (Figures 3A,B).
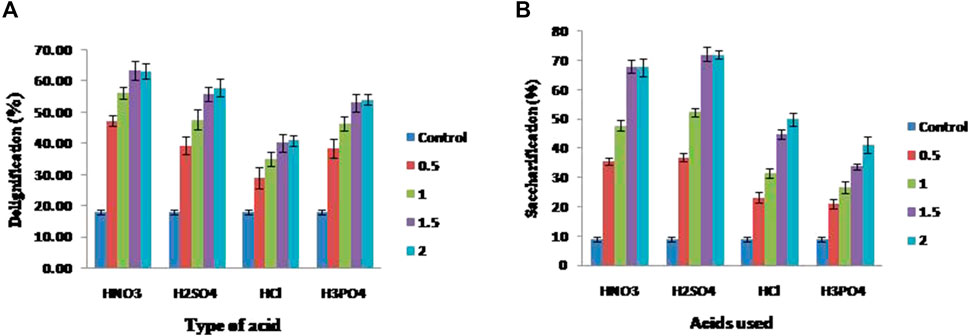
FIGURE 3. Effect of different acids on delignification and saccharification of corn cobs (A) delignification percentage of corn cobs and (B) xylose released during the saccharification.
Dilute Acid Hydrolysis of Alkali-Pretreated Biomass
Along with the removal of lignin, removal of hemicelluloses also contributes to enhancing the enzymatic digestibility; therefore, alkali-pretreated residues were subjected to acid hydrolysis for the hemicelluloses digestion. Among the different chemicals treated residues (NaOH, KOH, NaClO2, Na2SO3, and NH3), NaOH-pretreated residues resulted in maximum hemicellulose digestion of the biomass resulting in 25.92 g/L of xylose with 1.5% HNO3 whereas with 2%; it was 26.12 g/L which is not a notable difference. Therefore, 1.5% hydrolyzed residue was taken for further hydrolysis process, whereas the other four chemicals pretreated residues such as KOH, NaClO2, Na2SO3, and NH3 resulted in 21.24, 16.66, 17.98, and 21.32 g/L of xylose, respectively (Figure 4).
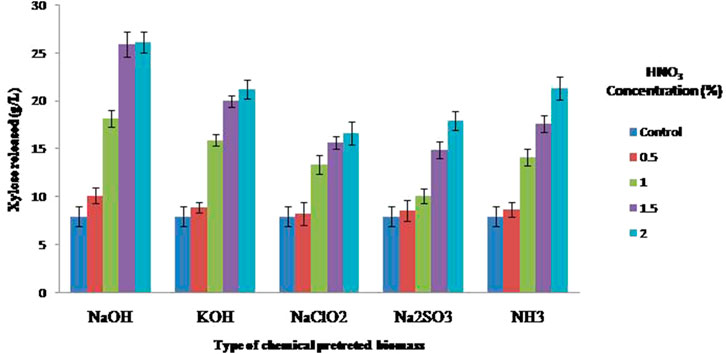
FIGURE 4. Xylose released during the dilute acid saccharification of different alkali-based pretreated corn cob residues.
Enzyme Hydrolysis
To correlate and compare the efficiency of different chemicals pretreatment on the efficiency of enzyme saccharification, all the pretreated residues were hydrolyzed. Among the alkali- and acid-based pretreated residue, the NaOH-pretreated residue resulted in the maximum glucose production of 25.87 g/L, holding the saccharification efficiency of about 86.18% with 30U enzyme load in 36 h of reaction time (Figure 5A). Similarly, the KOH-treated residue resulted in 19.4 g/L of glucose with the saccharification efficiency of 64.62% in 60 h of reaction time (Figure 5B), whereas NaClO2 and Na2SO3 residues resulted in 18.6 and 19.8 g/L of glucose with the hydrolysis efficiency of 61.96 and 65.96% in a reaction time of 72 and 60 h, respectively (Figures 5C,D). On the other hand, ammonia pretreatment had not much influence on improving enzymatic saccharification compared to alkali-pretreated residue. Aqueous ammonia-pretreated residue resulted in 18.4 g/L of glucose with 61.3% saccharification efficiency in 60 h of reaction time (Figure 5E). While the four acids such as HNO3, H2SO4, HCl, and H3PO4 pretreated residues resulted in 18.9, 19.6, 15.9, and 16.9 g/L of glucose with the saccharification efficiency of 62.96, 65.29, 52.96, and 56.3% in a reaction time of 48, 60, 72, and 60 h, respectively (Figures 5F–I).
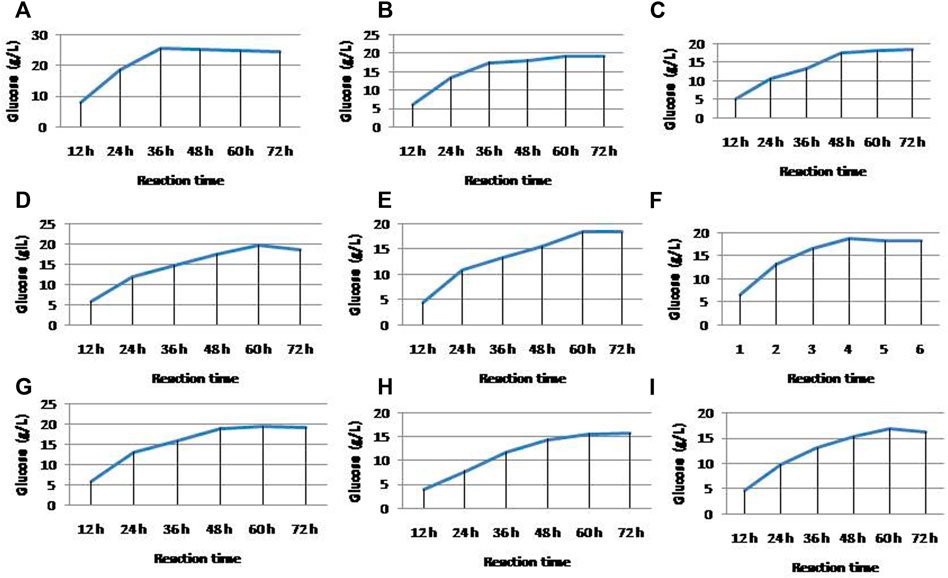
FIGURE 5. Enzyme hydrolysis of nine different chemicals pretreated corn cob residue, (A) enzyme hydrolysis of NaOH-pretreated and acid-hydrolyzed residues, (B) enzyme hydrolysis of KOH-pretreated and acid-hydrolyzed residues, (C) enzyme hydrolysis of NaClO2-pretreated and acid-hydrolyzed residues, (D) enzyme hydrolysis of Na2SO3-pretreated and acid-hydrolyzed residues, (E) enzyme hydrolysis of NH3-pretreated and acid-hydrolyzed residues, (F) enzyme hydrolysis of HNO3-pretreated residues, (G) enzyme hydrolysis of H2SO4-pretreated residues, (H) enzyme hydrolysis of HCl-pretreated and acid-hydrolyzed residues, and (I) enzyme hydrolysis of H3PO4-pretreated and acid-hydrolyzed residues.
Biomass Characterization
Biomass characterization is an essential step to establish the efficiency of biomass for the sustainable production of valued products. The raw and the pretreated corn cob biomass were subjected to characterization using SEM, XRD, and FTIR. With the aforementioned techniques, the rigorousness of different chemical pretreatments on the delignification and physicochemical properties of corn cobs was brought in to notice.
Scanning Electron Microscopy
A well-noticed difference in the microstructures and physical properties of pretreated and acid-hydrolyzed corn cob residues were observed from imaging studies (Figure 6). SEM micrographs of untreated raw corn cob showed a smooth and rigid surface (Figure 6A), whereas the NaOH-pretreated residue showed well-separated fibrils which aided in the efficient digestion of biomass (Figure 6B) (Alvarez-Barreto et al., 2021). Furthermore, the micrograph of ammonia-pretreated residue did not show much difference in the surface area and was almost similar in appearance with raw biomass (Figure 6C). In addition, acid-pretreated residue had well-separated fibrils and distortion in the biomass generated irregular cracks and cavities when compared to ammonia-pretreated residue (Figure 6D), whereas alkali-pretreated and dilute acid-hydrolyzed residue had well-separated fibrils and deep cavitation, which is well seen in Figure 6E, and the magnified image which showed a significant deep cavitation with increased surface area had a great positive impact on improving the enzymatic digestibility of biomass. The SEM images show the pretreatment biomass structural morphology, which subsequently had an impact on the efficiency of hydrolysis (Figure 6).
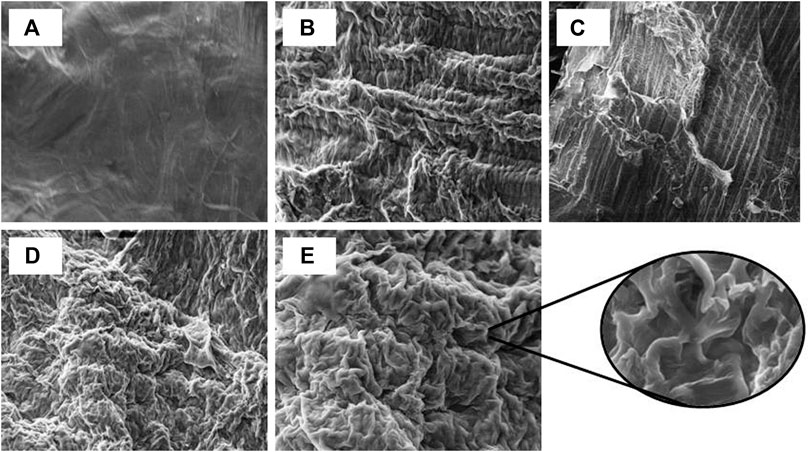
FIGURE 6. Scanning electron micrographs of different chemical-treated residues, (A) raw corn cob biomass, (B) NaOH-pretreated residues, (C) NH3-pretreated residues, (D) HNO3-pretreated residues, and (E) NaOH-pretreated and acid-hydrolyzed residues with magnification.
X-Ray Diffraction Analysis of Biomass
X-ray diffraction studies of raw, pretreated, and hydrolyzed biomass are illustrated in Figures 7A–D. As seen in diffractogram 7a, there is no sharp peak indicating the amorphous nature of biomass (Tongpoothorn et al., 2011). For raw corn cob biomass, the 2 theta values between 18 and 22° were owing to the nature of cellulose in biomass, which was not found to be sharp as seen in 7a–7c (raw, NaOH-pretreated and acid-pretreated corn cob residues), whereas the 7d diffractogram had a sharp peak, indicating the increase in crystalline nature of biomass with the removal of lignin and hemicelluloses in the residual samples.
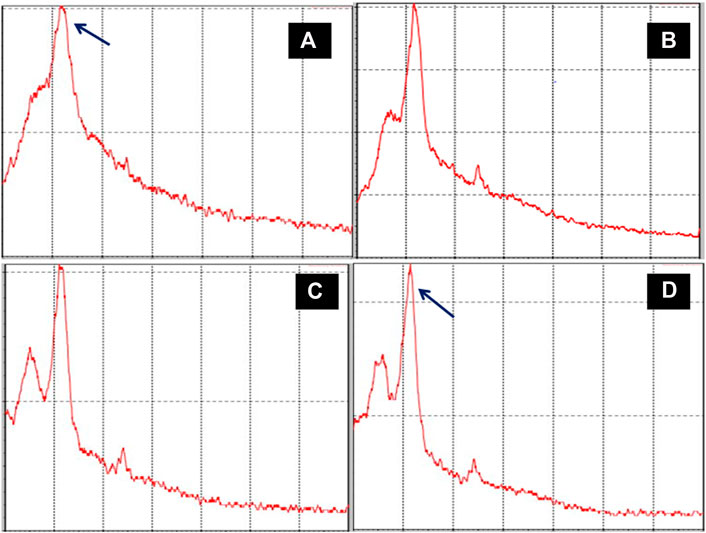
FIGURE 7. X-ray diffractograms of different chemical pretreated residues, (A) raw corn cob biomass, (B) NaOH-pretreated residues, (C) HNO3-pretreated residues, and (D) NaOH-pretreated and acid-hydrolyzed residues.
Fourier Transform Infrared Analysis of Biomass
The impact of pretreatment on biomass chemistry was studied by FTIR analysis (Figure 8). The functional group analysis was performed based on the peaks obtained at various regions from 600 to 4,000 cm−1 and compared between raw (Figure 8A) and treated residues (Figure 8B−D). The absorbance at 1,032 cm−1 corresponds to the aromatic C-H bond in plane, which is cleaved in the pretreated biomass (Figure 8B). Absorbance at 1,258 cm−1 corresponds to the ester linkage between lignin and hemicellulose. This peak also corresponds to guaiacyl ring; C-O stretch, which is seen in the guaiacyl aromatic methoxyl group present in lignin; and C-O stretch of ether linkage between carbohydrates and lignin. The absorbance at 1,258 cm−1 also corresponds to the ether linkage between carbohydrates and lignin, which is not seen in the case of pretreated sample, suggesting that lignin was solubilized during the pretreatment process. The peak at 1,315 cm−1 in the raw biomass is the C1-O vibration stretch of the syringyl unit of lignin (Zhao et al., 2008), which is not seen in pretreated biomass (Figure 4.9a and 4.9b).The peak at 1,651 cm−1 is related to the C=C carbonyl stretching of the aromatic skeletal vibration, a basic structural group in lignin which significantly decreased in pretreated biomass, suggesting that NaOH pretreatment had a significant impact on biomass chemistry (Zhao et al., 2008). The delignification process leads to a decrease in aromatic skeletal vibration of lignin that further decreases the absorbance. The peaks at 3,009 cm−1and 3,275 cm−1 in hydrolyzed biomass (Figures 8C,D) correspond to the O-H stretch of the carbonyl group, which is not seen in raw biomass (Figure 8A).
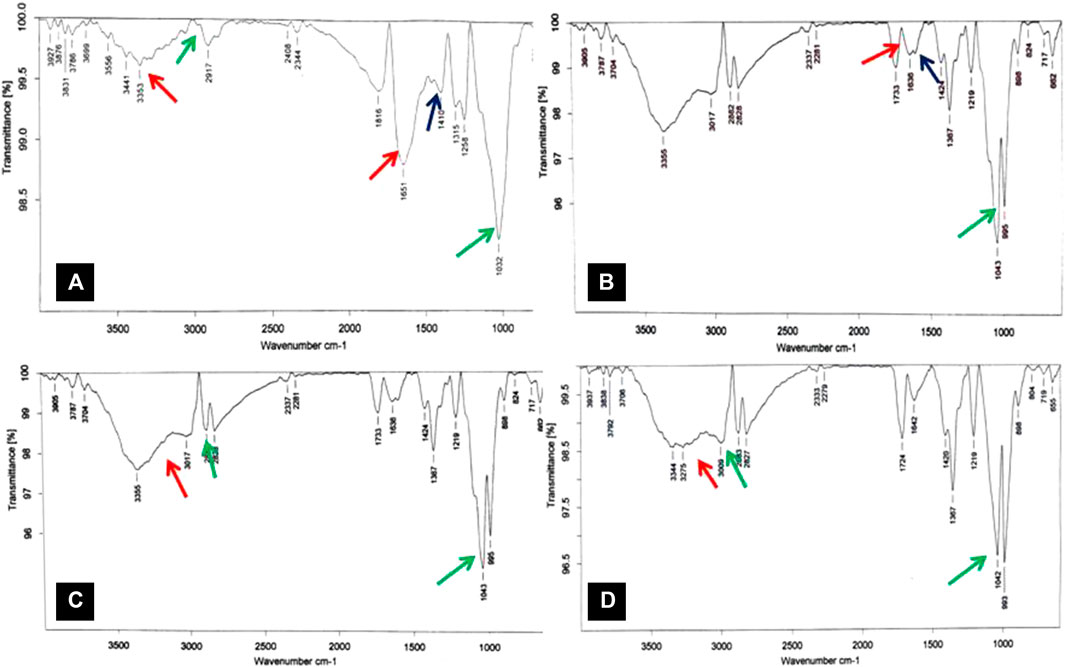
FIGURE 8. FTIR spectra of different chemical-treated corn cob residues, (A) spectra of raw corn cob biomass, (B) spectra of NaOH-pretreated residues, (C) spectra of HNO3-pretreated residues, and (D) spectra of sequential NaOH-pretreated and acid-hydrolyzed residues.
Discussion
The focus of the present study was to assess the effect of different chemical pretreatments and their impact on hydrolysis efficiency. This was accomplished by the chemical composition analysis, and the results are summarized in Table. 1, and this has led to some interesting inferences about the efficiency of chemicals on biomass composition. During the investigation of the pretreatment process, it is important to simultaneously look at the material balance by tracking the retention of both cellulose and hemicellulose. The retention of 95.6 and 93.49% of cellulose and hemicellulose in NaOH-pretreated biomass indicates the reliability of alkali in effective pretreatment.
Among the various chemicals used for delignification, 2% NaOH at 65°C overnight incubation resulted in the maximum delignification of 84.32 ± 2.57%, which is significantly high when compared to the rest of the chemicals used in the study. The present study is in line with our previous report (Sunkar and Bhukya, 2021), wherein the pretreatment of corn cobs with 2% NaOH at 55°C for 4 h resulted in 83.12% delignification. The slight increase may be due to the soaking of biomass overnight, which had a positive impact on hydrolysis efficiency in the proceeding step. Alkaline pretreatment significantly solubilizes lignin with a high pH (Garlock et al., 2011). Furthermore, the biomass pretreatment efficacy increases with the increase in temperature (Pobitra et al., 2019). This can be compared with the reports of Zuo et al. (2012), who reported 63.6 delignification of corn stover, using 1% NaOH and 8% NH4OH at 50°C for 48 h of soaking time. Similarly, Eliana et al. (2014) reported 88% delignification of elephant grass using 2% NaOH at 120°C for 1 h without being significantly affecting the cellulose fraction in the leftover residue. According to the reports of Li et al. (2014), soaking biomass in alkaline solvents improves the enzymatic digestibility of biomass with decreased enzyme loads. The aforementioned reports suggest that temperature, concentration of the chemical, and the reaction time are critical factors that enhance the delignification of biomass. However, the conventional and effective alkaline pretreatment has a drawback that lignin that is obtained in the wash is condensed and modified and therefore have limited application (Yoo et al., 2017). In other aspects with the extraction of total fermentable sugars, alkaline pretreatment would be a better option.
In addition, aqueous ammonia pretreatment of corn cobs in the present study resulted in 66.22 ± 3.09% delignification with overnight soaking, which is in agreement with the reports of Kim and Lee (2007) which reported 62% delignification of corn stover using 15% ammonia concentration at 60°C for 12 h. It was found in the present study that with the increase in the concentration of chemicals, the delignification percent also increased up to a certain level. It was found that there was a 50% increase in delignification from 10 to 20% concentration, which might be a specific critical range where maximum delignification occurs, and beyond that, there was no significant difference in the percentage of delignification. The reports of Kim and Lee (2005) reported 73.5% delignification of corn stover with 29.5% ammonia concentration in 60-day incubation, which is higher than that the present study. The reports suggest that the residence time, concentration of chemicals, and the temperature are the critical factors in the process of chemical delignification of biomass. In addition, the ratio of lignin to polymers also plays a critical role in the delignification process with different biomasses and different chemicals, whereas Sipponen and Osterberg (2019) reported 44.07% delignification of hydrothermally treated wheat straw using 12% ammonia at 160°C. However, using high temperatures during the pretreatment process results in the loss of pentose sugars; therefore, it is an important factor to balance the parameters with reduced severity factors. In addition, the main drawback of ammonia pretreatment is its toxicity and its less efficiency in delignification compared to alkali pretreatment which ultimately impacts the total productivity. Compared to alkali-based pretreatment, aqueous ammonia pretreatment is less efficient in delignifying lignocellulosic biomass. In addition, recycling the chemical when applied in a large scale is an important point of consideration.
During the dilute acid pretreatment process, both the concentration of acid and the residence time play a critical role. An increase in either of the two parameters leads to an increase in delignification and also reduces the solid recovery due to the sugar loss during the process, which has been envisaged in our previous report (Sunkar and Bhukya, 2021). With the increase in reaction time in the acid-based pretreatment process, the xylan recovery is significantly affected compared to glucan recovery. Therefore, to retain the total carbohydrate content without being significantly affected, the concentration of acid and reaction time must be optimized and balanced.
Among the various acids used in the study, HNO3 was found to be potent, resulting in 63.42 ± 3.13% delignification, which is 49.44% more than the control (13.44 ± 0.76). In addition, the acid-based pretreatment also led to 68% of hemicellulose digestion producing 21.02 g/L of xylose in the hydrolysate produced. This is in agreement with Ascencio et al. (2020) which reported hemicellulose digestibility with nitric acid pretreatment with a production of 14.22 g/L of xylose using 1% nitric acid with a reaction temperature set at 120°C for 30 min. Similarly, Rodriguez-Chong et al. (2004) reported 18.6 g/L of xylose and 1.32 g/L furfurals using 6% nitric acid for the sugarcane bagasse pretreatment at 122°C for 9.3 min.
Dilute H2SO4 pretreatment in the present study resulted in 63.31 ± 2.83% delignification at 2% concentration, whereas 1.5% concentration of acid resulted in 62.75 ± 2.20% delignification, which does not show any significant difference. The hemicelluloses digestibility was 72.12 and 72.7% with respect to 1.5 and 2%, respectively. The hydrolysate with 1.5% H2SO4 resulted in 19.92 g/L of xylose, which is in agreement with the reports of Martin et al. (2007) who used 2% H2SO4 for the pretreatment of sugarcane bagasse at 122°C for 60 min and produced 19.1 g/L of xylose. Among the four acids used in the study, the aforementioned two acids (HNO3 and H2SO4) had shown to have similar effect in terms of delignification and hemicellulose digestibility. It was common with all the acids that with the increase in concentration, the delignification and hydrolysis of polymer increased up to a certain level and thereafter, had no significant impact. Therefore, the digestibility depends on the reaction conditions and the concentration of chemical used. The acid-based pretreatment will unavoidably produce some inhibitors like furfurals, and phenols in particular show detrimental effects on the growth of fermentable microorganisms. Therefore, acid-based pretreated liquid needs to be detoxified prior to the fermentation process (Sunkar and Bhukya, 2021). In the large-scale production process, detoxification alone takes up 22% of the total production cost; therefore, it would be the best option to optimize the conditions and avoid detoxification (Koppram et al., 2012). Therefore, compared to acids, bases are more effective in the selective delignification process and in enhancing the accessibility of biomass without any significant loss in fermentable sugars.
Sequential treatment of biomass with alkali, followed by acid, removed 93.8% lignin and 83.2% of hemicellulose with the retention of 86.5% cellulose. With the absence of polymers, lignin, and hemicellulose, the leftover biomass is much accessible for enzyme hydrolysis. The present study is in agreement with the reports of Kim et al. (2012), who sequentially treated the empty palm fruit bunch fiber with acid, followed by alkali, which retained 82% of the cellulose in the leftover residue. Furthermore, with the removal of lignin and hemicelluloses, the surface area of the cellulose gets completely exposed for the enzymes to act on. The sequential pretreatment would be an effective approach to extract total fermentable sugars from both the hemicellulose and cellulose fractions of the biomass.
Maximum saccharification was obtained with 2% NaOH-treated residues, with 86.2% saccharification in 36 h of reaction time, which is significantly high compared to the rest of the eight chemical-pretreated residues, whereas least saccharification was observed with the HCl-treated residue, with 53% saccharification efficiency in 72 h of reaction time. This might be due to poor delignification during the pretreatment process that left the biomass with significant proportion of lignin, which affected the enzyme saccharification process. The results suggested that the more the percentage of delignification, the more the saccharification rate with fewer enzyme loads and the reaction time.
Delignification is the crucial step in extracting maximum fermentable sugars from the biomass. More lignin removal makes the biomass widely accessible for digestion and thereby more sugar yield (Wyman et al., 2005). Therefore, when the alkali- and acid-based delignification processes were performed, alkali worked most effectively in removing lignin, and thus enhances the biomass digestibility.
With the chemical analysis of corn cobs, it is clearly understood that the cellulose and hemicellulose retention is maximum in the case of alkali pretreatment compared to acids. However, the alkali-pretreated residue had maximum cellulose and hemicellulose retention, which may not be cellulose-accessible; therefore, the delignified residue is subjected to dilute acid hydrolysis prior to the enzymatic digestion. The efficient extraction of sugars from hemicelluloses aids in improving enzymatic digestion with minimum enzyme loads, whereas in the case of acid pretreatment, acids act both on lignin and hemicellulose partially, leading to the production of phenols along with sugars in the hydrolysate, which is again a cost-involving step to separate and use for the ethanol conversion process. In addition, acids act mainly on the hemicellulose, and therefore, the residue still does have lignin, which may interfere with the enzymatic digestibility. The lignin in the biomass forms the molecular architecture along with the non-phenolic compounds and forms a complex 3D network with a variety of ether and C-C bonds (Harmsen et al., 2010). Moreover, these residues may need more enzyme loads for the digestion process due to the inaccessibility of biomass than the alkali-pretreated residues.
Based on biomass characterization using the imaging studies by SEM, there was a clear difference in the raw- and alkali-pretreated biomass (Figures 6A,B). The raw corn cob was observed with the soft intact surface with rigid morphology without being affected during the initial physical processing steps. The pretreated images revealed interesting morphological changes that took place during the pretreatment process. However, aqueous ammonia (Figure 6C) could not show a significant impact on the biomass morphology compared to alkali- and acid-treated biomass (Figures 6B,D). In addition, the sequential removal of lignin and hemicellulose by alkali and acid treatments significantly enhanced the biomass porosity and the surface area that would greatly enhance the enzymatic digestibility, which is clearly seen in the magnified image (Figure 6E). The X-ray diffractogram patterns of raw-, alkali-, and acid-treated residues had no much difference in the peaks, but with the sequential alkali and acid treatment, the peak intensity was increased, which is clearly seen in Figure 7D. This may be due to the removal of both lignin and hemicellulose, which lead to an increase in the cellulose content in the biomass. The FTIR spectra of raw and pretreated biomasses were noted with great difference in the functional groups, which is clearly indicated with the colored arrows in Figure 8.
Compared to physical and biological pretreatment methods, chemical pretreatment is the most effective in terms of higher delignification efficiency with lower energy inputs and specified short time period.
Conclusion
The aforementioned results of the study on chemical pretreatment of corn cobs highlighted the critical importance of biomass delignification to yield maximum fermentable sugars. Among all the nine chemicals tested for their efficiency in the pretreatment process, sequential NaOH-pretreated and acid-hydrolyzed residues resulted in more than 80% yield of fermentable sugars from both cellulose and hemicellulose. Acid pretreatment due to its more action on hemicellulose than lignin, the leftover lignin part hampered the enzyme hydrolysis process. The study had clearly shown the correlation of delignification with the rate of hydrolysis. Furthermore, biomass characterization studies such as SEM, XRD, and FTIR have also confirmed that alkali pretreatment followed by dilute acid hydrolysis is the effective method of treatment. Overall, the present study reported that the sequential hydrolyzed residue requires fewer enzyme loads and reaction time, which makes the strategy more economic and reliable for large-scale operations.
Data Availability Statement
The raw data supporting the conclusion of this article will be made available by the authors, without undue reservation.
Author Contributions
BS carried out all the experiments and prepared the manuscript. BB designed the concept, monitored the experiments, and corrected and finalized the manuscript. Both the authors approved the final manuscript.
Conflict of Interest
The authors declare that the research was conducted in the absence of any commercial or financial relationships that could be construed as a potential conflict of interest.
Publisher’s Note
All claims expressed in this article are solely those of the authors and do not necessarily represent those of their affiliated organizations, or those of the publisher, the editors, and the reviewers. Any product that may be evaluated in this article, or claim that may be made by its manufacturer, is not guaranteed or endorsed by the publisher.
Acknowledgments
The authors are grateful to the Ministry of Human Resource Development, govt. of India, RUSA 2.0 program and DST-PURSE program of Osmania University for financial assistance and University Grants Commission, govt. of India, for BSR-RFSMS fellowship to carry out the research work.
References
Alvarez-Barreto, J. F., Larrea, F., Pinos C, M. C., Benalcázar, J., Oña, D., Viteri, D. A., et al. (2021). Chemical Pretreatments on Residual Cocoa Pod Shell Biomass for Bioethanol Production. Rev. Bionatura. 6, 1490–1500. doi:10.21931/RB/2020.06.01.9
Ascencio, J. J., Chandel, A. K., Philippini, R. R., and da Silva, S. S. (2020). Comparative Study of Cellulosic Sugars Production from Sugarcane Bagasse after Dilute Nitric Acid, Dilute Sodium Hydroxide and Sequential Nitric Acid-Sodium Hydroxide Pretreatment. Biomass Convers. Biorefin. 10, 813–822. doi:10.1007/s13399-019-00547-6
Banoth, C., Sunkar, B., Tondamanati, P. R., and Bhukya, B. (2017). Improved Physicochemical Pretreatment and Enzymatic Hydrolysis of rice Straw for Bioethanol Production by Yeast Fermentation. 3 Biotech. 7 (334), 1–11. doi:10.1007/s13205-017-0980-6
Baruah, J., Nath, B. K., Sharma, R., Kumar, S., Deka, R. C., Baruah, D. C., et al. (2018). Recent Trends in the Pretreatment of Lignocellulosic Biomass for Value-Added Products. Front. Energ. Res. 6, 141. doi:10.3389/fenrg.2018.00141
Bozzola, J. J., and Russell, L. D. (1998). Electron Microscopy Principles and Techniques for Biologists. 2nd Edn. Sudbury, MA: Jones and Barlett publishers.
Brodin, M., Vallejos, M., Opedal, M. T., Area, M. C., and Chinga-Carrasco, G. (2017). Lignocellulosics as Sustainable Resources for Production of Bioplastics - A Review. J. Clean. Prod. 162, 646–664. doi:10.1016/j.jclepro.2017.05.209
Chang, V. S., and Holtzapple, M. T. (2000). “Fundamental Factors Affecting Biomass Enzymatic Reactivity,” in Twenty-First Symposium on Biotechnology for Fuels and Chemicals. Applied Biochemistry and Biotechnology. Editors M. Finkelstein, and B. H. Davison (Totowa, NJ: Humana Press). doi:10.1007/978-1-4612-1392-5_1
Eliana, C., Jorge, R., Juan, P., and Luis, R. (2014). Effects of the Pretreatment Method on Enzymatic Hydrolysis and Ethanol Fermentability of the Cellulosic Fraction from Elephant Grass. Fuel 118, 41–47. doi:10.1016/j.fuel.2013.10.055
Garlock, R. J., Balan, V., Dale, B. E., Ramesh Pallapolu, V., Lee, Y. Y., Kim, Y., et al. (2011). Comparative Material Balances Around Pretreatment Technologies for the Conversion of Switchgrass to Soluble Sugars. Bioresour. Technol. 102, 11063–11071. doi:10.1016/j.biortech.2011.04.002
Ghose, T. K. (1987). Measurement of Cellulsase Activities, (Recommendations of Commission on Biotechnology IUPAC). Pure Appl. Chem. 59, 257–268. doi:10.1351/pac198759020257
Gupta, R., Mehta, G., and Kuhad, R. C. (2012). Fermentation of Pentose and Hexose Sugars from Corncob, a Low Cost Feedstock into Ethanol. Biomass Bioenergy 47, 334–341. doi:10.1016/j.biombioe.2012.09.027
Harmsen, P., Huijgen, W., Bermudez, L., and Bakker, R. (2010). Literature Review of Physical and Chemical Pretreatment Processes for Lignocellulosic Biomass. Wageningen UR Food Biobased Res. 978 (90), 757–800.
Hernandez-Mendoza, A. G., Saldana-Trinidad, S., Martínez-Hernandeza, S., Perez-Sarinana, B. Y., and Láinez, M. (2021). Optimization of Alkaline Pretreatment and Enzymatic Hydrolysis of cocoa Pod Husk (Theobroma Cacao L.) for Ethanol Production. Biomass and Bioenergy 154, 106268. doi:10.1016/j.biombioe.2021.106268
Kim, S., and Dale, B. E. (2004). Global Potential Bioethanol Production from Wasted Crops and Crop Residues. Biomass Bioenergy 26 (4), 361–375. doi:10.1016/j.biombioe.2003.08.002
Kim, S., Park, J. M., Seo, J.-W., and Kim, C. H. (2012). Sequential Acid-/Alkali-Pretreatment of Empty Palm Fruit Bunch Fiber. Bioresour. Technol. 109, 229–233. doi:10.1016/j.biortech.2012.01.036
Kim, T. H., and Lee, Y. Y. (2005). Pretreatment of Corn Stover by Soaking in Aqueous Ammonia. Appl. Biochem. Biotechnol. 124, 1119–1132. doi:10.1385/abab:124:1-3:1119
Kim, T. H., and Lee, Y. Y. (2007). Pretreatment of Corn Stover by Soaking in Aqueous Ammonia at Moderate Temperatures. Appl. Biochem. Biotechnol. 137-140, 81–92. doi:10.1007/s12010-007-9041-7
Koppram, R., Albers, E., and Olsson, L. (2012). Evolutionary Engineering Strategies to Enhance Tolerance of Xylose Utilizing Recombinant Yeast to Inhibitors Derived from Spruce Biomass. Biotechnol. Biofuels. 5, 32. doi:10.1186/1754-6834-5-32
Li, J., Zhou, P., Liu, H., Wu, K., Kang, X., Gong, Y., et al. (2014). A Comparison of Fermentation Strategies for Cellulosic Ethanol Production from NaOH-Soaked Sugarcane Bagasse at High Solid Loading with Decreased Cellulase Loading. Ind. Crops Prod. 62, 446–452. doi:10.1016/j.indcrop.2014.09.007
Martin, C., Alriksson, B., Sjöde, A., Nilvebrant, N.-O., and Jönsson, L. J. (2007). Dilute Sulfuric Acid Pretreatment of Agricultural and Agro-Industrial Residues for Ethanol Production. Appl. Biochem. Biotechnol. 137-140, 339–352. doi:10.1007/s12010-007-9063-1
Miller, G. L. (1959). Use of Dinitrosalicylic Acid Reagent for Determination of Reducing Sugar. Anal. Chem. 31, 426–428. doi:10.1021/ac60147a030
Pobitra, H., Kundua, S., Patel, S., Parthasarathya, R., Pramanik, B., Paz-Ferreiroa, J., et al. (2019). TGA-FTIR Study on the Slow Pyrolysis of Lignin and Cellulose-Rich Fractions Derived from Imidazolium-Based Ionic Liquid Pre-Treatment of Sugarcane Straw. Energ. Convers. Manage. 200, 112067. doi:10.1016/j.enconman.2019.112067
Rodriguez-Chong, A., Ramírez, J. A., Garrote, G., and Vázquez, M. (2004). Hydrolysis of Sugar Cane Bagasse Using Nitric Acid: A Kinetic Assessment. J. Food Eng. 61 (2), 143–152. doi:10.1016/S0260-8774(03)00080-3
Seidl, P. R., and Goulart, A. K. (2016). Pretreatment Processes for Lignocellulosic Biomass Conversion to Biofuels and Bioproducts. Curr. Opin. Green. Sustain. Chem. 2, 48–53. doi:10.1016/j.cogsc.2016.09.003
Sipponen, M. H., and Osterberg, M. (2019). Aqueous Ammonia Pre-Treatment of Wheat Straw: Process Optimization and Broad Spectrum Dye Adsorption on Nitrogen-Containing Lignin. Front. Chem. 7, 545. doi:10.3389/fchem.2019.00545
Soltani, S., Rashid, U., Yunus, R., and Taufiq-Yap, Y. H. (2015). Synthesis of Biodiesel through Catalytic Transesterification of Various Feedstocks Using Fast Solvothermal Technology: A Critical Review. Catal. Rev. 57 (4), 407–435. doi:10.1080/01614940.2015.1066640
Sunkar, B., and Bhukya, B. (2021). Bi-phasic Hydrolysis of Corncobs for the Extraction of Total Sugars and Ethanol Production Using Inhibitor Resistant and Thermotolerant Yeast, Pichia Kudriavzevii. Biomass and Bioenergy 153, 106230. doi:10.1016/j.biombioe.2021.106230
Sunkar, B., Kannoju, B., and Bhukya, B. (2020). Optimized Production of Xylanase by Penicillium Purpurogenum and Ultrasound Impact on Enzyme Kinetics for the Production of Monomeric Sugars from Pretreated Corn Cobs. Front. Microbiol. 11, 772. doi:10.3389/fmicb.2020.00772
TAPPI Technical Association of Pulp and Paper Institute (1992). TAPPI Test Methods, 1992-1993. Atlanta, Georgia, USA: TAPPI.
Tongpoothorn, W., Sriuttha, M., Homchan, P., Chanthai, S., and Ruangviriyachai, C. (2011). Preparation of Activated Carbon Derived from Jatropha Curcas Fruit Shell by Simple Thermo-Chemical Activation and Characterization of Their Physico-Chemical Properties. Chem. Eng. Res. Des. 89, 335–340. doi:10.1016/j.cherd.2010.06.012
Wyman, C. E., Dale, B. E., Elander, R. T., Holtzapple, M., Ladisch, M. R., and Lee, Y. Y. (2005). Coordinated Development of Leading Biomass Pretreatment Technologies. Bioresour. Technol. 96, 1959–1966. doi:10.1016/j.biortech.2005.01.010
Yoo, C. G., Li, M., Meng, X., Pu, Y., and Ragauskas, A. J. (2017). Effects of Organosolv and Ammonia Pretreatments on Lignin Properties and its Inhibition for Enzymatic Hydrolysis. Green. Chem. 19 (8), 2006–2016. doi:10.1039/c6gc03627a
Zhao, X., Wang, L., and Liu, D.-H. (2008). Peracetic Acid Pretreatment of Sugarcane Bagasse for Enzymatic Hydrolysis: A Continued Work. J. Chem. Technol. Biotechnol. 83, 950–956. doi:10.1002/jctb.1889
Zhu, M.-Q., Wen, J.-L., Su, Y.-Q., Wei, Q., and Sun, R.-C. (2015). Effect of Structural Changes of Lignin during the Autohydrolysis and Organosolv Pretreatment on Eucommia Ulmoides Oliver for an Effective Enzymatic Hydrolysis. Bioresour. Technol. 185, 378–385. doi:10.1016/j.biortech.2015.02.061
Keywords: corn cobs, pretreatment, hydrolysis, SEM, FTIR, XRD
Citation: Sunkar B and Bhukya B (2022) An Approach to Correlate Chemical Pretreatment to Digestibility Through Biomass Characterization by SEM, FTIR and XRD. Front. Energy Res. 10:802522. doi: 10.3389/fenrg.2022.802522
Received: 26 October 2021; Accepted: 21 March 2022;
Published: 03 May 2022.
Edited by:
Kalam Azad, Central Queensland University, AustraliaReviewed by:
Jitendra Kumar Saini, Central University of Haryana, IndiaJunLi Ren, South China University of Technology, China
Copyright © 2022 Sunkar and Bhukya. This is an open-access article distributed under the terms of the Creative Commons Attribution License (CC BY). The use, distribution or reproduction in other forums is permitted, provided the original author(s) and the copyright owner(s) are credited and that the original publication in this journal is cited, in accordance with accepted academic practice. No use, distribution or reproduction is permitted which does not comply with these terms.
*Correspondence: Bhima Bhukya, bhima.ou@gmail.com