- 1Department of Architecture, School of Human Settlement and Civil Engineering, Xi’an Jiaotong University, Xi’an, China
- 2Department of Architecture, Pusan National University, Busan, South Korea
- 3School of Computer Science and Technology, Xi’an Jiaotong University, Xi’an, China
- 4College of Railway Engineering, Zhengzhou Railway Vocational and Technical College, Zhengzhou, China
The high levels of energy consumption commonly seen today have severe consequences. For example, energy consumption in buildings traditionally takes the form of electricity generated from fossil fuels, which causes serious air pollution. Hence, the efficient application of clean energy systems in buildings is crucial. An increasing number of small-scale apartment buildings, such as single-room apartments and dormitories, have emerged as modern culture, and societal trends have led to a greater number of people living on their own. In this study, two strategies were proposed to improve the efficiency of solid oxide fuel cell co-generation systems (SOFC–CGSs) in small-scale apartments. A small-scale apartment building in Busan, Korea, was selected as the research object to verify the effect of the proposed strategies. One strategy was sharing a single SOFC–CGS among multiple households, and the other was integrating an SOFC–CGS with a photovoltaic (PV) system. The efficiency of the SOFC–CGS is expected to improve under these two operational strategies. In addition, the two proposed operational strategies were combined to improve the efficiency of the SOFC–CGS. To verify the proposed strategies, various simulations were conducted in this study. The simulation results indicate that each of the proposed strategies can improve the efficiency of the SOFC–CGS and save energy to varying degrees.
Introduction
Research Background
In recent years, the significant energy consumption arising from modern life has led to energy shortages and environmental problems. Because the energy consumption of buildings accounts for a large proportion of total energy consumption, clean energy systems for buildings have been developed and studied by researchers in recent years. There are two main energy resources used in buildings in Korea: electricity and natural gas. Natural gas, as a primary energy resource, emits minimal air pollutants when used. In contrast, although electricity is usually advertised as a clean energy resource because it emits no air pollutants when used, the process of electricity generation may emit large amounts of air pollutants. In Korea, 31% of electricity is produced by combusting coal, and only 4% of electricity is produced using renewable energy (KEPCO, 2016). Chang and Lee (2019) demonstrated that electricity generated from a public power plant cannot be considered a clean energy resource in Korea. As a clean energy device, the solid oxide fuel cell (SOFC) consumes hydrogen or natural gas and generates electricity through chemical interactions, and this procedure has no negative impacts on the environment (Lee et al., 2009). However, the electricity generation process can cause significant heat dissipation. To utilize dissipated heat and thereby increase the efficiency of the SOFC, the solid oxide fuel cell co-generation system (SOFC–CGS) was developed. An SOFC–CGS, which combines an SOFC with a water tank and a backup boiler, can collect heat dissipated from the cell stack and use it to heat a tank of water.
Recent fuel cell–related studies have explored methods for improving the efficiency of residential fuel cells mainly by considering the working principles of the fuel cell subsystems. In residential buildings, to optimize the subsystem efficiency of proton exchange membrane fuel cell hybrid power systems, Bizon et al. (2018) applied the global extremum seeking algorithm. Adam et al. (2015) proposed a more efficient heat recovery subsystem for fuel cells. Arsalis et al. (2015) designed a coupled system including a vapor-compression heat pump and a micro-combined heat and power (micro-CHP) to improve the heat use efficiency of residential fuel cells. Milcarek et al. (2020) considered using fuel-rich combustion from a residential furnace to enhance the power for fuel cell startup. Hoseintabar–Marzebali (2019) applied a dynamic strategy to properly control DC/AC (direct current/alternating current) converters, and they introduced a utilization factor to improve the transient performance of residential fuel cells. An energy management system can also boost the efficiency of residential fuel cell use (Rouholamini and Mohammadian, 2015; Aki et al., 2016). Ou et al. (2021) applied the optimal Pontryagin minimum principle to build an energy management system for realizing fuel minimization, battery protection, and hot water supply. An energy demand–oriented approach was studied to improve the efficiency of residential fuel cells (Ozawa and Kudoh, 2018a). Ozawa and Kudoh (2018a) tested the properties of the fuel cell cogeneration system in different types of residential houses. Nguyen and Ishihara (2021) proposed a peer-to-peer energy supply method to increase the self-efficiency of such dwellings.
Residential fuel cells and fuel cell vehicles can provide power to support each other. To compensate for the shortage of hydrogen stations in Japan, Haneda et al. (2017) proposed a method that uses the fuel processor system in the residential fuel cell to supply hydrogen to fuel cell vehicles. Robledo et al. (2019) proposed a vehicle-to-grid charging method that uses the power generated from fuel cell scooters for residential use.
From the perspectives of techno-economics and policy, Pellegrino et al. (2015) analyzed the feasibility of applying residential fuel cells in Germany and Denmark. From the perspective of environmental impact, some researchers demonstrated that residential fuel cells can significantly lessen residential greenhouse gas emissions (Koki and Akihito, 2013; Koki et al., 2014; Ozawa and Kudoh, 2018b). To find the best approach to improve fuel cell efficiency, Longo et al. (2019) conducted a life cycle energy and environmental analysis of a residential solid oxide fuel cell micro-combined of heat and power (micro-CHP) system. The results revealed that the operation step causes more than 97% of the energy impact; hence, improving the operation efficiency of the system can be an effective approach to reducing the energy impact.
Recent studies on improving the efficiency of residential fuel cells have mainly concentrated on typical apartments or houses. However, pluralistic culture and lifestyles in the modern society have led to a growing number of small-scale apartments for youths living alone in Korea. Therefore, the focus of this study was the operational efficiency improvement of SOFC–CGSs in small-scale apartments. The strategies proposed herein can guide the installation of SOFC–CGSs in small-scale Korean apartments in the future.
Research Contribution
The goal of this research is to improve the efficiency of SOFC–CGS in small-scale apartments. In order to clarify the novelty and contribution of this study, the typical approaches to improve the fuel cell efficiency of recent studies were organized in Table 1, and the typical technical methods were compared with the strategies proposed in this study.
According to Table 1, from a technical prospective, most of the recent studies concentrate on optimizing the subsystem and energy management system to improve fuel cell efficiency. As technology of the fuel cell vehicles is also rapidly developing in recent years, the integrated utilization of the residential fuel cell and fuel cell vehicle is also an effective approach to improve the efficiency of the devices. It is revealed that the efficiency of the residential fuel cell varies with different energy demand pattern. However, the studies of fuel cell efficiency improvement strategies through energy demand side analysis are comparatively less. Therefore, due to the increasing number of the small-scale buildings in recent years, the aim of this study was to explore the strategies to improve the efficiency of residential SOFC–CGSs in small-scale apartments from an approach of the energy demand side analysis. A small-scale apartment building in Korea was selected as a research object to verify the strategies proposed in this study. The contribution of this research is that two strategies were proposed to improve the efficiency of SOFC–CGS in terms of the energy usage pattern of the small-scale apartment building. The first strategy involves sharing a single SOFC–CGS between multiple households because a higher load factor can improve the efficiency of the SOFC–CGS. The second strategy involves integrating an SOFC–CGS with a small photovoltaic (PV) system. The PV system satisfies the low levels of the residential electricity demand during the mid-day hours. The efficiency of the SOFC–CGS can be regarded as 100% during these hours because when the PV system is fulfilling the electricity demand, there is no natural gas consumption in the SOFC–CGS; moreover, there is no need for restarting the device because the standby duration of the SOFC–CGS is 24 h; in other words, there is also no electricity usage from the SOFC–CGS during several hours of mid-day standby. In addition, the two strategies can be combined (that is, an integrated SOFC–CGS and PV system can be shared by multiple households) to improve efficiency. The conclusion section summarizes the advantages and disadvantages of each strategy. In order to conduct the simulation analysis, the building was modeled using commercial software, and a program for an SOFC–CGS was created based on a calibrated mathematical model (Sumiyoshi et al., 2015; Yamamoto, 2018; Yamamoto et al., 2018).
Materials and Methods
Research Methodology
In the first part of this study, the annual energy consumption of the target residential building was predicted using the software Design Builder (Version 5.5). The calculation model of Design Builder used in the study was EnergyPlus (Version 8.2.0). EnergyPlus is a sophisticated calculation engine used to analyze building heating, cooling, lighting, and ventilation, and Design Builder is a calibrated software system created based on EnergyPlus and has a clear user interface. In the second part of this study, strategies for improving the efficiency of SOFC–CGS were investigated. As the programing language Visual Basic.NET has excellent compiling speed and a mature Graphical User Interface module, it was chosen to build the programs to simulate the clean energy systems in this study, and the Visual Studio 2019 Community Version was used to compile the program. In the programs, the calibrated mathematical models of PV systems and SOFC–CGSs were referred from previous studies. Eventually, the effects of each strategy were verified, and the drawbacks and advantages of each strategy were identified.
Modeling the Small-Scale Apartment Building
Along with the drastic development in Korea, the average age of marriage has been increasing to approximately 30 years, and many young people are choosing not to get married. Thus, many young adults live on their own, and this phenomenon has led to the emergence of numerous small-scale apartments. Generally, a small-scale apartment is defined as an apartment that can accommodate one or two people and has an area of approximately 20–30 m2. Small-scale apartment buildings are usually developed near university campuses, factory sites, and suburban areas near business areas. In Korean residential houses or apartments, the conventional energy supply scenario for buildings is to use natural gas for room heating, cooking, and water heating and to use electricity from a grid for lighting, cooling, and electrical devices. As the problems of energy shortages and the environmental impacts of energy production are being paid increasing attention by the Korean government, clean energy devices such as residential fuel cells are being promoted for application in buildings. However, as the number of small-scale apartment buildings is significantly increasing, how to efficiently apply clean energy devices in this type of building should be considered. Therefore, in this study, strategies for improving the efficiency of SOFC–CGSs in small-scale apartment buildings were investigated under the traditional energy supply scenario. In this study, a small-scale apartment building near a university campus was selected to serve as an example, and a model of the selected small-scale apartment building was developed by using Design Builder. Based on the EnergyPlus Engine in Design Builder, the annual building energy consumption was analyzed. Figure 1 shows the model of the selected building.
Weather data in Busan were obtained from the American Society of Heating, Refrigerating, and Air-Conditioning Engineers (ASHRAE) database. The occupancy schedule of different parts of the small-scale apartment building was obtained from the ASHRAE standard 90.2, 2010 (American Society of Heating, 2010). The specifications of the small-scale apartment building are briefly summarized in Table 2. Other energy consumption standards, such as heating, cooling, lighting, and occupancy density, were obtained from the Korea Energy Agency and are shown in Table 3 (Kim et al., 2009; Jang et al., 2010; Jehean, 2017). According to the construction thermal specifications of Korean construction law, the thermal parameters of the exterior wall, interior wall, and roof of the building were set in the model as shown in Table 3. The hourly energy consumption of the selected building within a year was precisely predicted through the accurate input parameters.
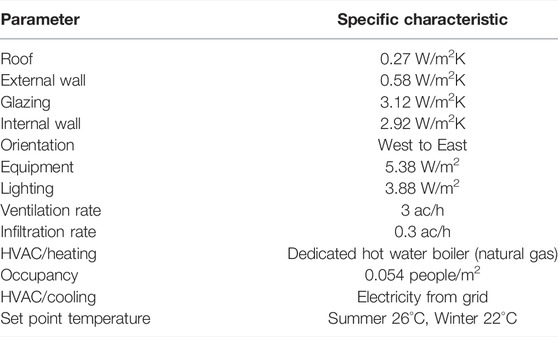
TABLE 3. Construction specifications of the selected apartment building (Kim et al., 2009; Jang et al., 2010; Jehean, 2017).
There are four floors with the same configuration in the selected apartment building, and each floor has five rooms. Because the calibrated commercial software Design Builder is used to predict the energy consumption of each room, it is assumed that the energy consumption pattern and amount in each floor should be the same. Furthermore, under this research method, the energy consumption patterns of the five rooms on each floor are assumed to be the same. Therefore, only the rooms on the first floor are selected to show the detailed data of this study. Busan has four distinctive seasons. January 15 and 16 are selected as the representative dates of winter; July 15 and 16 are selected as the representative dates of summer; and September 24 and 25 are selected as the representative dates of the intermediate seasons. Based on the ASHRAE standard schedule for residential houses (American Society of Heating, 2010), the hourly electricity and hot water consumption of Room 1 on the first floor on the representative dates are shown in Figure 2A. Because heating energy was provided directly by natural gas, the SOFC–CGS operation does not consider heat energy consumption. As shown in Figure 2A, without counting the heating energy demand, the electricity energy demand and hot water demand of Room 1 in winter and the intermediate seasons are the same; because of the fluctuating cooling energy consumption, the electricity demand in summer is higher than in other seasons. In contrast, the hot water demand in each season is the same since hot water demand is not influenced by weather conditions. Figure 2B shows the annual energy demand of each room on the first floor. According to the area of each room, the total annual natural gas demand (including heating energy need) and electricity demand of Rooms 1 and 2 are relatively low and those of Rooms 3, 4, and 5 are relatively high.
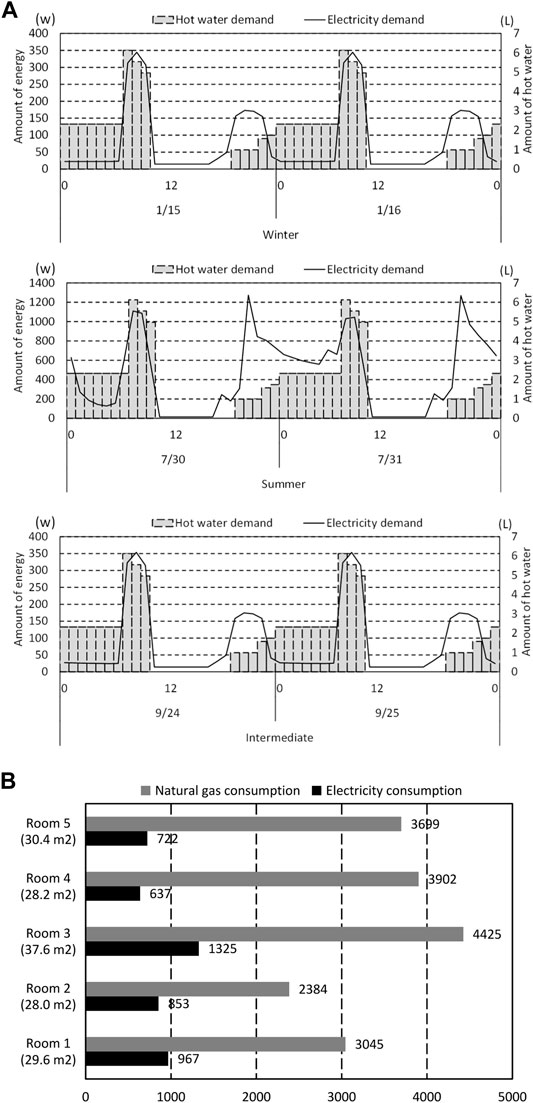
FIGURE 2. Energy demand analysis of the small-scale apartments: (A) hourly electricity and hot water demand of Room 1 on the representative dates in each season and (B) annual energy consumption for first floor rooms.
Modeling of Solid Oxide Fuel Cell Co-Generation System and Photovoltaic
Modeling of the Solid Oxide Fuel Cell Co-Generation System
The maximum efficiency of the SOFC device considered in this study is around 46.4%. However, the efficiency of the SOFC–CGS is improved to 77.4% because the efficiency of heat usage of the SOFC–CGS is a constant 31%. Within the SOFC–CGS, the water tube transfers heat from the cell stack to the water tank, and the subsystem radiator works when the water tank overheats. The limit control temperature of the water tank is 65°C. The water temperature before entering the backup boiler is adjusted to ≤33°C by mixing with city water; the backup boiler can reheat the water temperature to 40°C. Figure 3A presents the detailed working procedure of the SOFC–CGS. According to Figure 3A, the backup boiler also supports the supply of hot water for heating through another pipe, and the temperature of hot water for heating in Korea is generally around 35°C–45°C. The hourly simulation analysis was conducted as the hourly climate data were utilized in this study.
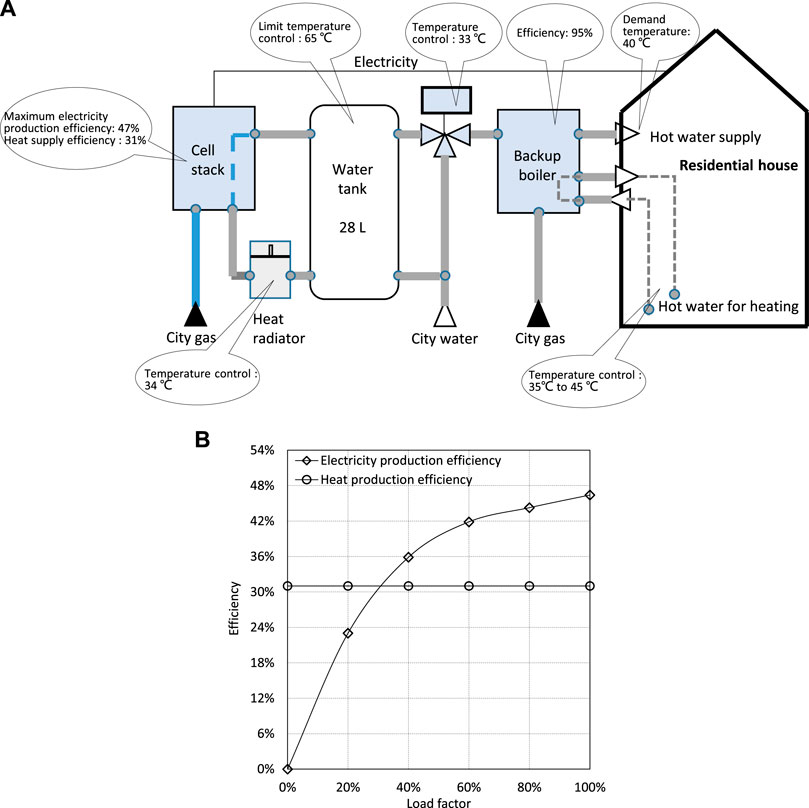
FIGURE 3. Working procedure and efficiency pattern of the SOFC–CGS: (A) working procedure of the SOFC–CGS and (B) correlation between load factor and efficiency of SOFC–CGS.
Figure 3B and Eq. 1 clarify the efficiency of SOFC–CGS. Table 4 organizes the specifications of the SOFC–CGS model in this study. Based on the data in Figure 3B, we can confirm that the efficiency increases when the load factor increases. However, the efficiency of heat usage is constant regardless of the load factor.
where LoadF (%) represents the load factor and EfRate (%) is the efficiency of electricity generation of the SOFC.
The SOFC–CGS model was retrieved from a previous study (Sumiyoshi et al., 2015; Yamamoto, 2018; Yamamoto et al., 2018). The detailed calculation of the SOFC–CGS working procedure follows Eq. 2 through (Eq. 10). Initially, the limit amount of electricity production at the current moment is calculated by utilizing the tracking velocity of electricity production and electricity production at the previous moment.
where Elmcur (kWh) is the limit amount of electricity production at the current moment, Elpre (kWh) is the electricity production at the previous moment, SPel (kWh/h) is the tracking velocity of electricity production, and Tinter (h) is the time interval of the calculation. If the electricity demand is greater than or equal to the limit amount of electricity production at the current moment, the electricity production of the SOFC at the current moment is the limit amount of electricity production; otherwise, it is equal to the electricity demand.
The next step is to calculate the load factor and natural gas consumption of the SOFC with Eqs 3, 4.
In Eqs 3, 4, LoadF (%) is the load factor, Elmax (kWh) is the limit electricity production of SOFC, Ngcon (kWh) is the natural gas usage, and EfRate (%) is the electricity production efficiency of the SOFC at the current moment. Meanwhile, the heat dissipation from the cell stack is calculated through Eq. (5).
where Htout (kWh) is the heat dissipation of the SOFC at the current moment and EfHt (%) is the efficiency of heat dissipation. As shown in Figure 3B, the heat dissipation efficiency is constantly 31% in this model.
The heat transfer method from the cell stack to hot water tank is through a water tube; the water in the water tube absorbs the heat from the cell stack and transfers it to the water tank. The water temperature in the water tank is controlled by adjusting the water flow velocity in the tube according to the input water temperature to the radiator. The controlled temperature of the radiator is 34°C; if the temperature is more than 34°C, the water velocity can be determined by Eq. 6; otherwise, it can be determined by Eq. 7. Moreover, Eq. 8 can be applied to calculate the heat loss from the radiator when the water temperature entering the radiator is more than 34°C.
In Eqs 6–8, Vlw (L/h) represents the water velocity; Twout (°C) is the limit water temperature out from the cell stack, and it is a constant value of 65°C in the model; Twin (°C) is the water temperature entering the radiator at the current moment; Cpw (kWh/(L/h) °C) represents the specific heat capacity of the water. Htrad (kWh) is the heat loss from the radiator; Twbo (°C) and Twbi (°C) are the water temperatures entering and exiting the radiator, respectively.
The natural gas usage from the backup boiler is determined at last in this model. Through Eqs 9, 10, the effective heat supply and natural gas usage from the boiler are determined.
In these equations, Ngbp (kWh) is the natural gas usage from the boiler; Htbs (kWh) is the effective heat supply from the boiler; Efbp (%) is the boiler efficiency, which is 95% in the model; Twdd (°C) represents the demanded temperature of the hot water; Twbi (°C) is the water temperature entering the boiler at the current moment; and Vodd (L/h) represents the demanded hot water quantity at the current time.
The program flowchart is presented in Figure 4. In the program, the weather data and system specifications are set first, and then the electricity consumption, natural gas consumption, heat usage, and heat dissipation are calculated through the mathematical model. Based on this calculation process, the calculation loop will continue until data are no longer input.
Modeling of the Integrated PV and Solid Oxide Fuel Cell Co-Generation System
One of the strategies proposed in this study for improving the efficiency of the SOFC–CGS is integrating the SOFC–CGS with a PV system. This works for two reasons. The first reason is that the PV system can satisfy a low electricity demand from each room during the mid-day hours. The PV system generates its peak electricity during the mid-day hours, and a small PV system is able to generate enough electricity during those hours to satisfy the room energy demand. The second reason is that during the hours when the PV system generates enough electricity for the room, there is no need for any electricity generation from the SOFC–CGS. Therefore, the efficiency of the SOFC–CGS can be assumed to be 100% during those hours.
To analyze the integrated SOFC–CGS and PV system, a mathematical model of the PV system was developed in this study based on the previous research by Yoza et al. (2014). Table 5 shows the specifications of the PV model. The amount of electricity generated by the model can be calculated by the following Eq. (11).
where η is the conversion efficiency of the PV panel, n is the number of PV panels, SPV is the area of a single PV panel, IPV is the effective solar radiation on an inclined panel surface, and tCR is the ambient temperature. The best installation angle for PV panels in Busan, Korea, is 30° (Oh et al., 2012). Moreover, the effective solar radiation on an inclined panel surface can be calculated by using the method proposed by Erbs et al. (1982).
Using the mathematical models of the SOFC–CGS and PV, a program for the integrated SOFC–CGS and PV system was created. The calculation flowchart of this program is shown in Figure 5.
Effect of Applying Solid Oxide Fuel Cell Co-Generation System or Photovoltaic in the Building
Effect of Applying Solid Oxide Fuel Cell Co-Generation System in the Small-Scale Apartment
First, the effect of using an SOFC–CGS in each room of the small-scale apartment was analyzed. There are numerous hourly simulation results from all rooms, so just the sample simulation data for Room 3 were chosen to present in Figures 6A,B, show the electricity production and heat supply from the SOFC and the energy reduction from the radiator. Based on the data in Figures 6A,B, we can confirm that the electricity and hot water demand are mainly concentrated in the morning and evening, and the energy supply pattern corresponds with the energy demand. As the representative date in this figure is in the summer, the electricity demand fluctuates because of the fluctuating cooling energy demand. Therefore, the electricity production from the SOFC is at the limited value when the electricity demand is at the peak value. Figure 6C summarizes the efficiency performance of the system under this energy supply scenario. The efficiency of the system is the highest in Room 3 as the energy demand and load factor are the highest among the five rooms. The results show that a higher energy demand leads to higher efficiency performance of the system. Based on the working principles of the SOFC, two strategies were proposed for improving the efficiency of the system.
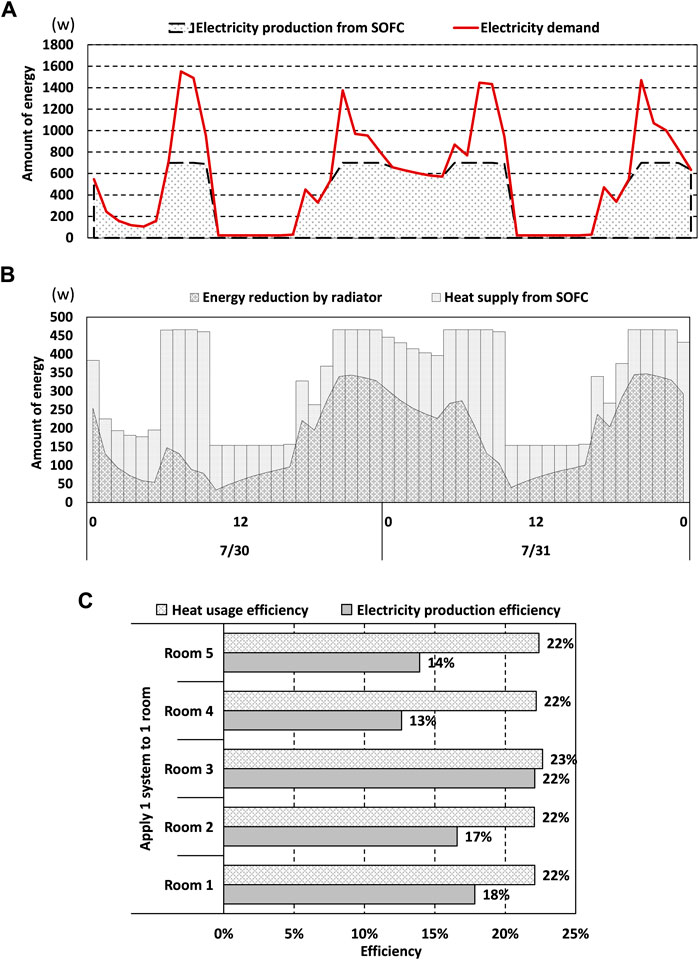
FIGURE 6. Energy performance of using one SOFC–CGS in each room: (A) electricity production and demand of SOFC–CGS simulation for Room 3, (B) heat dissipation and usage of SOFC–CGS simulation for Room 3, and (C) annual electricity production and heat usage efficiency of one SOFC–CGS/room.
Effect of Applying the Photovoltaic System in the Small-Scale Apartment
Generally, peak energy demand in residential buildings occurs in the morning and evening. In contrast, the PV system generates the largest amount of electricity around mid-day. Therefore, using a large PV system without using an energy storage system, such as a storage battery, will degrade the efficiency performance of the PV system. On the contrary, the energy consumption of residential rooms during the mid-day hours is low, and Figure 3B shows that the electricity generation efficiency of SOFCs is lower because of lower load factors. If a small PV system is used to satisfy the low electricity demand from the rooms during the mid-day hours, the SOFC–CGS operational status changes from running to standby, and the efficiency of the SOFC–CGS can be regarded as 100% due to the absence of natural gas consumption during these hours. Another advantage of this strategy is that a small PV system will not cause a heavy financial burden. Therefore, a single PV panel with a 1.3 m2 area was selected to be simulated in each room before simulating an integrated PV and SOFC–CGS.
Figure 7A shows the simulation results for Room 3. Even though the amount of electricity generated from a 1.3-m2 PV system is small, it is enough to satisfy the electricity demand from the room during the mid-day hours. Figure 7B shows the annual electricity generation and supply from the PV system for the representative rooms.
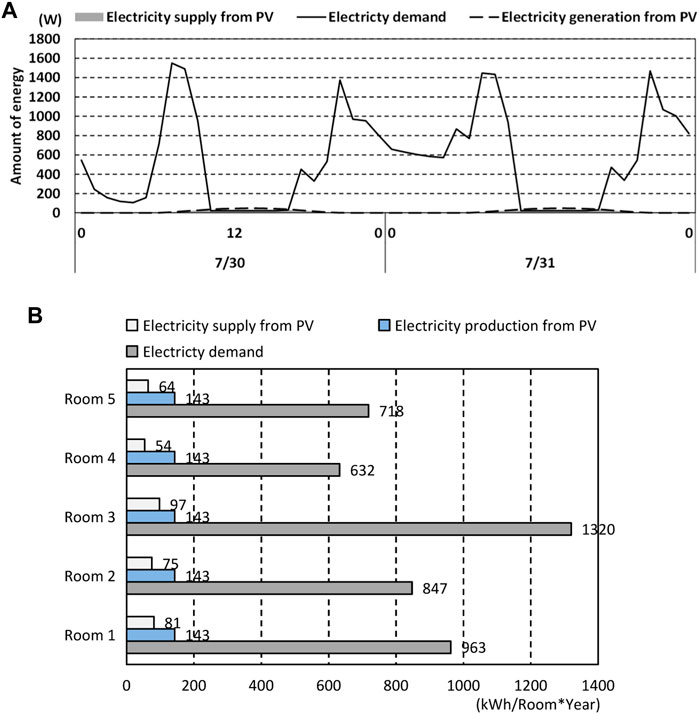
FIGURE 7. Energy performance of only using a 1.3-m2 PV system in each room: (A) PV simulation results for Room 3, (B) annual electricity demand, and PV system generation and supply.
Analysis of the Proposed Strategies
Analysis of Hourly Simulation Results
The first strategy proposed in this study to enhance the efficiency of the system was sharing one SOFC–CGS among multiple (two or three) rooms. It is shown in Figure 2B that from the view of annual energy consumption/room, the annual energy demand of Room 2 was the lowest and that of Room 3 was the highest. To maintain the balance of the energy demand in different rooms, it was decided that Rooms 2 and 3 would share one SOFC–CGS and Rooms 1, 4, and 5 would share one SOFC–CGS. Figure 8 shows the hourly electricity demand and generation together with the heat output of the cell stack and heat dissipation of the radiator for Rooms 2 and 3 on the representative date of a year. These can be compared to Figure 6, which shows similar data for only Room 3 when operating one system.
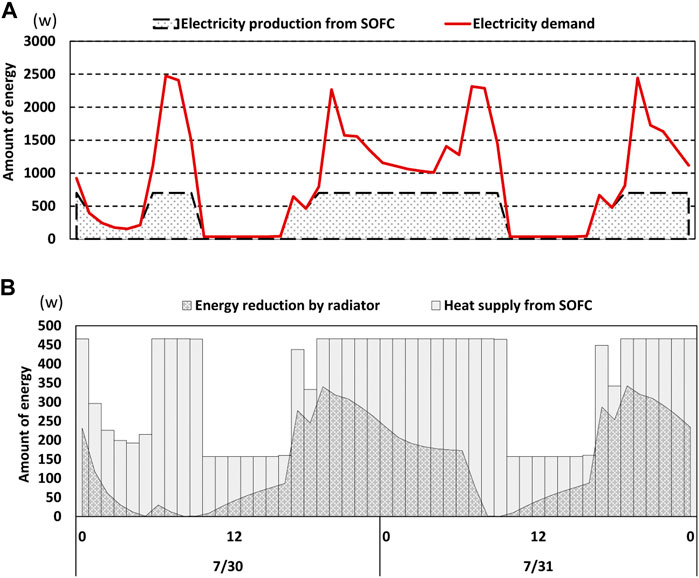
FIGURE 8. SOFC–CGS simulation for Rooms 2 and 3: (A) electricity production and demand and (B) heat dissipation and usage.
In addition, Figure 8 also shows that when multiple rooms shared one system, the energy demand from the rooms and the load factor of the system were increased, thereby improving the electricity generation efficiency of the system because of the efficiency mathematical model of the system. In addition, when the system was shared, the heat dissipation from the radiator was reduced, which improved the heat usage efficiency for hot water since the hot water demand increased when sharing one system among multiple rooms.
The second strategy proposed in this study for increasing the efficiency of the system was using an SOFC–CGS integrated with a small PV of 1.3 m2 in the room. As mentioned in the previous section, the energy demand in a residential building is mainly concentrated in the morning and evening, and the energy demand in the mid-day hours is low. Hence, it was hypothesized that a small PV would be able to satisfy the low electricity demand from Room 3 during the mid-day hours to increase the efficiency of the system. Figure 9 shows the hourly results of the electricity supply from each device and the heat reduction and supply from the SOFC–CGS on the representative dates. It also indicates that the small PV satisfied the electricity demand during the mid-day hours from Room 3, which eliminated the need for heat supply from the SOFC during these hours. Therefore, the heat loss from the radiator was also nil. The natural gas usage during those hours for the system was nil, hence, the efficiency of the system can be regarded as 100% during those hours.
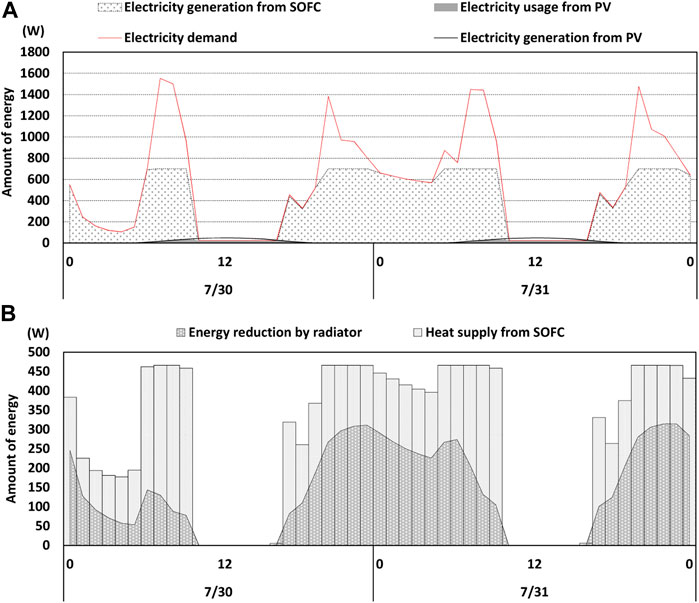
FIGURE 9. PV/SOFC–CGS simulation for Room 3: (A) electricity supply/system and (B) heat reduction and supply.
System Efficiency Analysis
The electricity production efficiency and heating utilization rate of the system in each case are shown in Figures 10A,B. In these figures, there are two groups of data. The first group in the 3D bar charts is the red column, which shows the results of the cases of using an SOFC–CGS in one or multiple rooms. The other group is the blue column, which shows the results of the cases of using an integrated SOFC–CGS and PV system in one or multiple rooms.
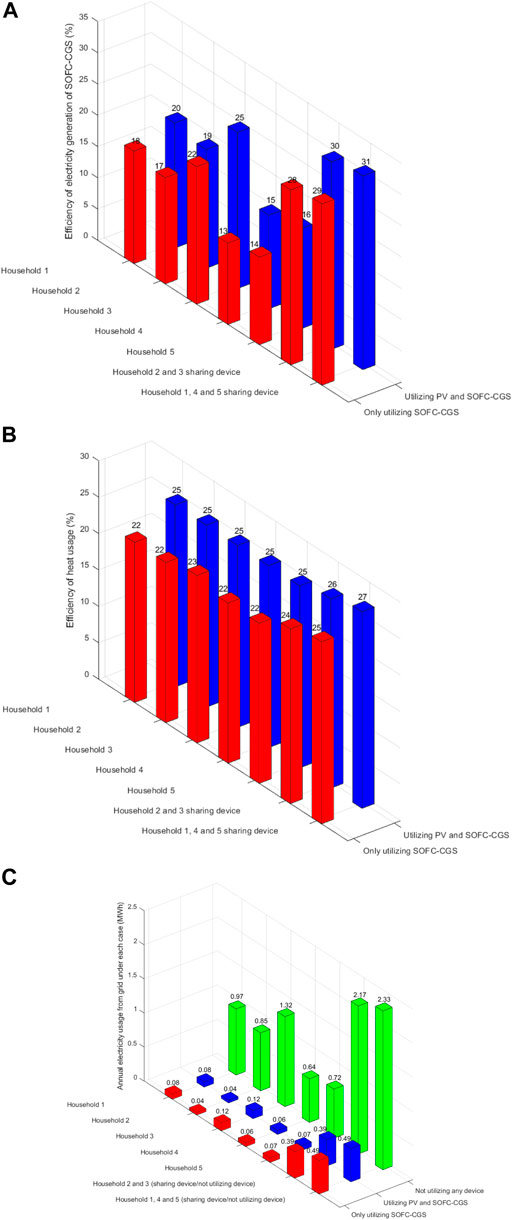
FIGURE 10. Energy performance of the proposed strategies: (A) electricity production efficiency of the SOFC–CGS for each case, (B) heat usage efficiency of the SOFC–CGS for each case, and (C) annual amount of electricity drawn from the grid for all cases.
As shown in the red columns of Figure 10A, the high load factor of electricity generation of the system resulted in high efficiency; when the demand was increased, the load factor of electricity generation also increased. When multiple rooms shared one system, the electricity generation efficiency was 6%–11% higher than that when each room used one system. According to the second strategy, the electricity demand was low during the mid-day hours, which results in a low load factor and low efficiency of electricity generation of the cell stack. When the PV system satisfies the low electricity demand during the mid-day hours, the efficiency of electricity generation of the system can be regarded as 100% in all cases with a PV in the system. Therefore, by comparing the blue columns with the red columns, it can be determined that for both cases; the efficiency of electricity production of the integrated SOFC–CGS and PV system was 2%–3% higher than that of the SOFC–CGS.
The red columns in Figure 10B show that the heat usage efficiency of the SOFC–CGS was 1%–3% higher when used for multiple rooms than when used for one room. The hot water demand was significantly higher for multiple rooms, so the heat loss was reduced because more heat transferred from the cell stack to water tank was utilized for hot water, which also increased the heat usage efficiency. The blue columns in Figure 10B show that the heat usage efficiency of the integrated SOFC–CGS and PV system was 2%–3% higher than that of the SOFC–CGS in both cases. The heat supply from the SOFC–CGS and loss from the radiator is nil during the mid-day hours when the PV satisfies the electricity demand, which also indicates that the heat usage efficiency during these hours can be regarded as 100%; therefore, the efficiency of heat usage is higher in these cases.
In Figure 10C, there are three groups of data; the red columns and blue columns show the amount of electricity drawn from the grid for the cases shown in Figures 10A,B. The green columns show the amount of electricity drawn from the grid when neither device is used. Figure 10C compares the annual amount of electricity drawn from the grid for each case. The annual amount of electricity drawn from the grid was the same whether an SOFC–CGS was used on its own or together with a PV system because the amount of electricity supplied by the devices in each case was the same.
The amount of electricity drawn from the grid when sharing a system between multiple rooms was 2.4 times higher than when not sharing. However, the amount of electricity drawn from the grid when using an SOFC–CGS with or without a PV system was much less than the cases that did not use any devices.
Therefore, the two strategies proposed in this study have a significant effect of improving the efficiency of an SOFC–CGS with mild influence on the electricity usage from the grid.
Conclusion
As modern life is driving an increasing number of youths to live on their own, small-scale apartments are being rapidly produced by real-estate developers. Therefore, efficient operation strategies for clean energy systems in small-scale apartment buildings should be explored. In this study, based on the operation regulations of residential SOFC–CGSs and the energy demand pattern of the small-scale apartment, two strategies were proposed to improve system efficiency in small-scale apartments. Then, a small-scale apartment building in Busan, Korea, was selected as the research object for simulation analysis. Positive results were obtained, and it is verified that the strategies proposed in this research can noticeably improve the efficiency of SOFC-CGS in small-scale apartments. The specific conclusions are presented below.
(1) The first strategy proposed in this study was to use one system for multiple rooms because higher power demand can lead to higher cell stack electricity production load factor and higher electricity production efficiency. In addition, higher hot water demand can lead to higher heat usage to reduce heat loss from the radiator. According to the result of the verification analysis, the operating conditions of applying one system in each room and sharing one system among multiple rooms were both simulated. In the case of applying one system to one room, the electricity production efficiency was 13%–22%, and the heat usage efficiency was 22%–23%. In the case of applying one system to multiple rooms, the electricity production efficiency increased to 28%–29%, and the heat usage efficiency increased to 24%–25%. Therefore, it is confirmed that the first strategy can significantly improve the efficiency of the SOFC-CGS in the small-scale apartments.
(2) The second strategy was using small PV systems (single panel with 1.3 m2 area) together with a residential SOFC–CGS. Small PV systems can satisfy the low electricity demand from the rooms during the mid-day hours, and the electricity production and heat usage efficiency of the system can be regarded as 100% during these times owing to there being no energy demand or supply from the SOFC during these times. In terms of the result of the verification analysis, the efficiency of electricity production of an SOFC–CGS integrated with a small PV system (1.3 m2) was between 1% and 3% higher than that of an SOFC–CGS operating on its own when being used for one or multiple rooms. The heat usage efficiency of the SOFC–CGS integrated with a small PV system was between 2% and 3% higher than that of an SOFC–CGS operating on its own when being used for one or multiple rooms. Although the efficiency improvement effect of the second strategy is not as high as the first strategy, it has the advantage of utilizing renewable energy to substitute for the primary energy usage from the fuel cell. Furthermore, there will not be a large financial burden when a small PV system (1.3 m2) is used.
(3) Compared to the device efficiency when only applying one strategy, the verification analysis showed that the electricity production efficiency and heat usage efficiency of an SOFC–CGS can be improved by 2% if both strategies are used (that is, an integrated SOFC–CGS and PV system is shared among multiple rooms). Therefore, the application of these two strategies simultaneously is recommended in practice.
(4) Due to the fact that 31% of electricity is produced by combusting coal in Korea, the usage of the electricity drawn from the grid has a negative effect to the environment. According to the analysis results in this study, the amount of electricity drawn from the grid when sharing an SOFC–CGS with or without a PV system among multiple rooms was 2.4 times higher than when using one system in each room. However, the amount of electricity drawn from the grid when using the SOFC–CGS with or without a PV system than not using any device was much less. Thus, the strategies proposed in this research can not only improve the efficiency of the SOFC–CGS but also benefit the environment.
The limitation of this study is that only the efficiency improvement strategies of the residential SOFC–CGS for the small-scale apartment buildings were discussed. However, the rapid development of the modern technologies such as information technology creates various life styles which can noticeably influence the energy consumption pattern of the households. Therefore, the efficiency improvement strategies of the residential SOFC–CGS for the households with various life styles will be investigated in the future.
Data Availability Statement
The original contributions presented in the study are included in the article/Supplementary Material, further inquiries can be directed to the corresponding authors.
Author Contributions
HC conceived and completed this manuscript. I-HL supported the building modeling. B-QZ supported the software utilization. Y-JY supported the program coding. Y-LH supported the writing and review of this manuscript.
Funding
This work was supported by the National Natural Science Foundation of China (61872224, 12090021), and the Key Scientific Research Projects of Colleges and Universities in Henan Province (Grant No. 22B560020).
Conflict of Interest
The authors declare that the research was conducted in the absence of any commercial or financial relationships that could be construed as a potential conflict of interest.
Publisher’s Note
All claims expressed in this article are solely those of the authors and do not necessarily represent those of their affiliated organizations, or those of the publisher, the editors, and the reviewers. Any product that may be evaluated in this article, or claim that may be made by its manufacturer, is not guaranteed or endorsed by the publisher.
References
Adam, A., Fraga, E. S., and Brett, D. J. L. (2015). Options for Residential Building Services Design Using Fuel Cell Based Micro-Chp and the Potential for Heat Integration. Appl. Energ. 138, 685–694. doi:10.1016/j.apenergy.2014.11.005
Aki, H., Wakui, T., and Yokoyama, R. (2016). Development of an Energy Management System for Optimal Operation of Fuel Cell Based Residential Energy Systems. Int. J. Hydrogen Energ. 41 (44), 20314–20325. doi:10.1016/j.ijhydene.2016.09.079
American Society of Heating (2010). Refrigeration, and Air-Conditioning Engineers. Energy Efficient Design for Low-Rise Residential Buildings. Atlanta, GA, USA: ASHRAE.
Arsalis, A., Kær, S. K., and Nielsen, M. P. (2015). Modeling and Optimization of a Heat-Pump-Assisted High Temperature Proton Exchange Membrane Fuel Cell Micro-Combined-Heat-and-Power System for Residential Applications. Appl. Energ. 147, 569–581. doi:10.1016/j.apenergy.2015.03.031
Bizon, N., Mazare, A. G., Ionescu, L. M., and Enescu, F. M. (2018). Optimization of the Proton Exchange Membrane Fuel Cell Hybrid Power System for Residential Buildings. Energ. Convers. Manage. 163, 22–37. doi:10.1016/j.enconman.2018.02.025
Chang, H., and Lee, I. H. (2019). A Case Study of Decreasing Environment Pollution Caused by Energy Consumption of a Dormitory Building Which Only Using Electricity by Efficiently Simulating Applying Residential SOFC (Solid Oxide Fuel Cell). Archit. Res. 21, 21–29. doi:10.5659/AIKAR.2019.21.1.21
Erbs, D. G., Klein, S. A., and Duffie, J. A. (1982). Estimation of the Diffuse Radiation Fraction for Hourly, Daily and Monthly-Average Global Radiation. Solar Energy 28, 293–302. doi:10.1016/0038-092x(82)90302-4
Haneda, T., Ono, Y., Ikegami, T., and Akisawa, A. (2017). Technological Assessment of Residential Fuel Cells Using Hydrogen Supply Systems for Fuel Cell Vehicles. Int. J. Hydrogen Energ. 42 (42), 26377–26388. doi:10.1016/j.ijhydene.2017.08.152
Hoseintabar-Marzebali, M. (2019). Transient Performance Improvement of a Stand-Alone Fuel Cell for Residential Applications. Sustain. Cities Soc. 50, 101650. doi:10.1016/j.scs.2019.101650
Jahirul, M. I., Rasul, M. G., Schaller, D., Khan, M. M. K., Hasan, M. M., and Hazrat, M. A. (2022). Transport Fuel from Waste Plastics Pyrolysis - A Review on Technologies, Challenges and Opportunities. Energ. Convers. Manage. 258, 115451. doi:10.1016/j.enconman.2022.115451
Jang, C. V., Ahn, B., Kim, C., and Hong, W. (2010). A Study on the Insulation Performance of the Super Window Considering the Evaluation of Building Energy Rating. J. Korean Sol. Energ. Soc. 30, 1–6. Available at: http://www.riss.kr/link?id=A79730455 Accessed June 6, 2020.
Jehean, S. (2017). The Analysis of Environmental Impact Index Using Life Cycle Assessment for Korean Traditional Building. Doctor’s Dissertation. Busan, Korea: Department of Architecture of the Pusan National University. Available online: http://dcollection.pusan.ac.kr/public_resource/pdf/000000125135_20191014233329.pdf (Accessed on December 18, 2017).
KEPCO (2016). Sustainable Report. Available online: www.kepco.co.kr (Accessed on December 6, 2020).
Kim, C., Ahn, B., Kim, J., and Jang, C. (2009). A Study on the Evaluation of Building Energy Rating Considering the Insulation Performance of the Window and wall in Apartment Houses. Available online: http://www.koreascience.or.kr/article/CFKO200921140051433.view (Accessed on June 26, 2018).
Koki, Y., Akihito, O., and Myonghyang, L. (2014). Study on Performance Evaluation of Residential SOFC Co-Generation Systems. Soc. Heat. Air-Cond. Sanit. Eng. Jpn. 203, 25–33. doi:10.18948/shase.39.203_25
Koki, Y., and Akihito, O. (2013). Study on Performance Evaluation of Residential SOFC Co-Generation Systems Part 2 Equipment Characteristics and Installation Effect. Soc. Heat. Air-Cond. Sanit. Eng. Jpn. 70, 281–284. doi:10.18948/shasekinki.2012.0_281
Lee, T. H., Choi, J. H., Park, T. S., Choi, H. Y., and Yoo, Y. S. (2009). Development and Performance Test of SOFC Co-Generation System for RPG. J. Korean Soc. New Renew. Energ. 6, 361–364. Available at: https://www.dbpia.co.kr/Journal/articleDetail?nodeId=NODE06189597 Accessed January 12, 2021.
Longo, S., Cellura, M., Guarino, F., Brunaccini, G., and Ferraro, M. (2019). Life Cycle Energy and Environmental Impacts of a Solid Oxide Fuel Cell Micro-Chp System for Residential Application. Sci. Total Environ. 685, 59–73. doi:10.1016/j.scitotenv.2019.05.368
Milcarek, R. J., DeBiase, V. P., and Ahn., J. (2020). Investigation of Startup, Performance and Cycling of a Residential Furnace Integrated with Micro-Tubular Flame-Assisted Fuel Cells for Micro-Combined Heat and Power. Energy 196, 117148. doi:10.1016/j.energy.2020.117148
Nguyen, D. H., and Ishihara, T. (2021). Distributed Peer-To-Peer Energy Trading for Residential Fuel Cell Combined Heat and Power Systems. Int. J. Electr. Power Energ. Syst. 125, 106533. doi:10.1016/j.ijepes.2020.106533
Oh, S., Ihm, P., and Lee, K. (2012). Optimal Electric Generation for Fixed-type Photovoltaic System Installed for Residential Building in Korea. GRI Rev. 14, 271–288. Available at: https://www.dbpia.co.kr/Journal/articleDetail?nodeId=NODE01953961 Accessed January 12, 2021.
Ou, K., Yuan, W.-W., and Kim, Y.-B. (2021). Development of Optimal Energy Management for a Residential Fuel Cell Hybrid Power System with Heat Recovery. Energy 219, 119499. doi:10.1016/j.energy.2020.119499
Ozawa, A., and Kudoh, Y. (2018). Performance of Residential Fuel-Cell-Combined Heat and Power Systems for Various Household Types in Japan. Int. J. Hydrogen Energ. 43 (32), 15412–15422. doi:10.1016/j.ijhydene.2018.06.044
Ozawa, A., and Kudoh, Y. (2018). Performance of Residential Fuel-Cell-Combined Heat and Power Systems for Various Household Types in Japan. Int. J. Hydrogen Energ. 43, 15412–15422. doi:10.1016/j.ijhydene.2018.06.044
Pellegrino, S., Lanzini, A., and Leone, P. (2015). Techno-Economic and Policy Requirements for the Market-Entry of the Fuel Cell Micro-Chp System in the Residential Sector. Appl. Energ. 143, 370–382. doi:10.1016/j.apenergy.2015.01.007
Robledo, C. B., van Leeuwen, L. B., and van Wijk, A. J. M. (2019). Hydrogen Fuel Cell Scooter with Plug-Out Features for Combined Transport and Residential Power Generation. Int. J. Hydrogen Energ. 44 (56), 29648–29657. doi:10.1016/j.ijhydene.2019.04.103
Rouholamini, M., and Mohammadian, M. (2015). Energy Management of a Grid-Tied Residential-Scale Hybrid Renewable Generation System Incorporating Fuel Cell and Electrolyzer. Energy and Buildings 102, 406–416. doi:10.1016/j.enbuild.2015.05.046
Sumiyoshi, D., Okuda, Y., Akashi, Y., Ozaki, A., and Watanabe, T. (2015). The Examination for Suitable Spec of Solid Oxide Fuel Cell Co-Generation System and the Proposal of Tank Minimization by Using Bas-Tab in Collective Housing. J. Environ. Eng. 80, 441–450. doi:10.3130/aije.80.441
Yamamoto, T., Amatatsu, K., and Sumiyoshi, D. (2018). Estimation Energy Saving Effects of Sharing Fuel Cell with Two Households by Simulation Program: Study on Effective Usage of the home Fuel Cell in the Apartment Housing. J. Environ. Eng. 83, 365–374. doi:10.3130/aije.83.365
Yamamoto, T. (2018). Analysis of Impact to Applying Residential Fuel Cell by Different Lifestyle. Doctor’s Dissertation. Fukuoka, Japan: Department of Architecture of Kyushu University. Available online: https://catalog.lib.kyushu-u.ac.jp/opac_download_md/1931681/hues0393.pdf (Accessed on June 16, 2020).
Keywords: system efficiency, energy saving, SOFC-CGS, PV, small-scale apartments
Citation: Chang H, Lee I-H, Zhai B-Q, Yang Y-J and Hou Y-L (2022) Proposing Strategies for Efficiency Improvement by Using a Residential Solid Oxide Fuel Cell Co-Generation System in a Small-Scale Apartment Building. Front. Energy Res. 10:788097. doi: 10.3389/fenrg.2022.788097
Received: 01 October 2021; Accepted: 15 March 2022;
Published: 13 April 2022.
Edited by:
Daniel Zanetti De Florio, Federal University of ABC, BrazilReviewed by:
Yi Zong, Technical University of Denmark, DenmarkJoel Melo, Federal University of ABC, Brazil
Copyright © 2022 Chang, Lee, Zhai, Yang and Hou. This is an open-access article distributed under the terms of the Creative Commons Attribution License (CC BY). The use, distribution or reproduction in other forums is permitted, provided the original author(s) and the copyright owner(s) are credited and that the original publication in this journal is cited, in accordance with accepted academic practice. No use, distribution or reproduction is permitted which does not comply with these terms.
*Correspondence: Bin-Qing Zhai, cmVzZWFyY2hnZWVrQHNpbmEuY29t; Yao-Long Hou, aHlsenp0bEAxNjMuY29t