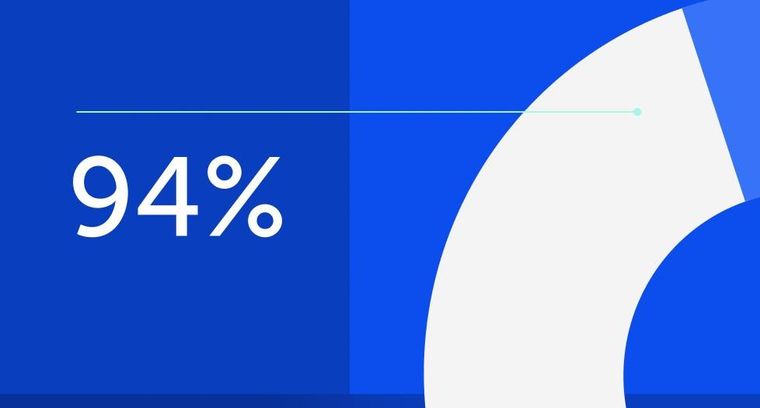
94% of researchers rate our articles as excellent or good
Learn more about the work of our research integrity team to safeguard the quality of each article we publish.
Find out more
ORIGINAL RESEARCH article
Front. Energy Res., 09 January 2023
Sec. Nuclear Energy
Volume 10 - 2022 | https://doi.org/10.3389/fenrg.2022.1100536
This article is part of the Research TopicAdvancements in Nuclear and Irradiation ExperimentsView all 6 articles
This paper focuses on laser-induced breakdown spectroscopy (LIBS) as an off-gas sensor and details a conceptual irradiation experiment to evaluate its performance in an environment that is more realistic of an operational molten salt reactor (MSR). MSRs are a promising advanced nuclear design that use high-temperature liquid salts as the coolant, fuel, or a combination of both. They will generate and subsequently evolve fission products into the reactor headspace during operation. These evolved fission products will necessitate an online off-gas system to treat the reactor cover gas before recycling it to the core. Such a system, especially during the early deployment phase, will benefit from online sensors to inform operators of the treatment system’s performance. Optical spectroscopy is well-suited to fit this task as it can be remotely deployed using fiber-optics and can detect most species at high frequencies. LIBS can detect nearly every element on the Periodic Table, stable or radioactive, making it an ideal candidate. The provided conceptual experiment discusses the facility requirements, salt capsule design, and detailed testing campaigns with corresponding simulated LIBS spectra.
Advanced nuclear reactor concepts continue to be pursued globally as a next-generation source of clean energy. One of these advanced reactor concepts is the molten salt reactor (MSR), which uses fluoride or chloride molten salts as either the coolant or the coolant and fuel (Serp et al., 2014). These reactors have the potential for high thermodynamic efficiency as they are typically designed to operate at several hundred degrees (i.e., 650°C) and low pressures, such as less than 1 bar above atmosphere (Serp et al., 2014; Forsberg, 2020). Because the primary circuit fluids have low volatility, the potential for a catastrophic accident involving pressure is very unlikely. The goal for operating these reactors is to have “walk away” safety. However, MSRs present challenges in terms of the chemistry of the salt and the configuration of the fuel, particularly in the case of salt-fueled reactors. Investigation of phenomena affecting the chemistry and speciation of fission products relevant to MSRs in the presence of a radiation field is critical for licensing. One of the goals of understanding fission product behavior is to develop online instrumentation to determine the chemistry of the fission products, which will inform their transport, deposition, and in some cases, the redox state of the salt in the core.
Molten salt–fueled reactors will evolve fission gas during burnup. The release of these radionuclides to the headspace of a reactor will require continuous monitoring and inventory control (Andrews et al., 2021a). Although many fission products are expected to be highly soluble in the salt (e.g., lanthanides), fission gases such as xenon and krypton will readily escape the salt. Reactive gases, such as hydrofluoric and hydrochloric acids, may accumulate in the headspace as a result of radiolysis. Interhalogens that include fission product iodine may also have significant volatility. Tritium can form from activation of 6Li in LiF–BeF2 carrier salt.
A conceptual off-gas system has been proposed with various treatment components to properly remove radioactive species, reactive gases, and the thermal load to recycle the gas back into the reactor headspace (Riley et al., 2019; Andrews et al., 2021a). However, the further development of this system’s design relies on two key capabilities: first, robust sensors that can measure many stable and radioactive elements, and second, the ability to model the gases and particles being removed from the headspace of an MSR so components can be designed appropriately.
For the first capability, optical spectroscopy has been proposed as a strategy for remotely monitoring an off-gas system (Hughey et al., 2020; Andrews et al., 2021b; Felmy et al., 2021; Andrews et al., 2022a; Andrews and Myhre, 2022). There are multiple forms of optical spectroscopy that, when coupled, can provide a molecular, elemental, and isotopic fingerprint in real time. Another benefit of optical spectroscopy is the ability to be deployed remotely using fiber optics. Sensitive equipment (i.e., spectrometer, laser, and computers) can be housed in a shielded location. The laser or light source is delivered through a fiber-optic cable to the sample, and the optical response is collected, focused, and returned to the spectrometer via a different fiber.
The second capability, accurate species evolution modeling, requires a fundamental understanding the release of species from the salt. Experiments on salt species volatility and gas solubility have been performed in recent years, but there is a significant lapse in the reported literature when it comes to irradiated salts where radiolysis may influence behavior (Moon et al., 2022).
We present an early-stage concept for an irradiation experiment to test spectroscopic off-gas sensors. These sensors would be used to perform exploratory studies, such as the detection of select gaseous fission products under irradiation conditions from representative molten salts. The focus of this paper is laser-induced breakdown spectroscopy (LIBS), though sensors based on other optical spectroscopy techniques, such as Raman and laser-induced fluorescence, could also be tested using the irradiation experimental capability described here. To study the release characteristics, a LIBS sensor will be installed inline to monitor the elemental composition of released gases, vapors, and aerosols. The LIBS sensor has been demonstrated on fission product relevant aerosols and noble gases in an idealized system; however, it has not yet been tested on irradiated salts to assess the effects of radiolysis on the equipment or analysis (Andrews et al., 2022a; Andrews and Myhre, 2022).
In this study, both an experimental plan and the corresponding anticipated spectral signatures of various species are provided. The experimental plan includes information on the salt capsule to be irradiated and the configuration of the LIBS sensor to be evaluated. The anticipated spectral signatures were produced using Saha-Boltzmann calculations to compare relative intensities and wavelengths of species anticipated to be measured during specific experiments. Key challenges and gaps in existing spectral databases are discussed as well.
The information provided in this study is the result of simulations and conceptual designs with the goal of guiding proposed experiments. From the conceptual salt cell design, several different experiments are recommended. For each of these experiments, artificial spectra were produced for the species anticipated to be released and detected by the LIBS system in order to evaluate their spectral signatures with respect to one another. These artificial spectra were generated using atomic spectral properties, along with Saha-Boltzmann methods, assuming that the spectra would be measured while the plasma was in a state of local thermodynamic equilibrium (LTE).
During LTE, the intensity of an emission peak emitted during the transition from an upper to a lower energy level can be described using Eq. 1
where
where Ne is the electron density of the plasma, Eionization is the ionization energy, and s1 and s2 refer to the neutral and singly ionized species, respectively (de Oliveira Borges et al., 2018). Eqs. 1, 2 can be used together to generate the emission intensities of all transitions for a given element if the transition properties are known. For further information on LIBS plasma transitions the reader is referred elsewhere (Cremers and Radziemski, 2013). In this study, the plasma temperature and electron density were held constant at typical LIBS levels of 1 eV and 1017 cm−3, respectively unless otherwise specified. All artificial spectra shown represent pure emission profiles (i.e., CS1 and CS2 sum to unity) and are normalized to their maximum emission intensity. The artificial spectra in this study were generated using an in house Python script based on the fundamental equations above.
The proposed irradiation experiment would be performed in a national laboratory or university research reactor. The experiment would require a sample well of sufficient size to fit a furnace or cartridge heaters for the salt capsule to be heated above the salt meting point (e.g., 650°C). In addition to the heating requirements, an outer container used to seal the salt capsule itself within a vacuum or inert atmosphere to mitigate leaking issues. An example illustration of a salt cartridge is shown in Figure 1.
FIGURE 1. Example schematic of salt test capsule with (1) sparge gas line to bubble gases through salt, (2) cover gas line to sweep salt gas interface, (3) gas outlet to send stream to inline measurement systems, (4) fluoride/chloride molten salt, (5) capsule headspace, and (6) potential corrosion coupons or graphite samples.
The temperature-controlled vessel would have lines into the cell for both a cover gas and sparge gas. These lines would be valved to perform tests where the salt cartridge is sealed, tests where the cartridge is intermittently vented, and continuous flow tests. When operating in a cover gas configuration the applied gas would be He or Ar. When testing in the cover gas configuration, the gas would only purge the headspace above the salt. When operating in a sparge gas configuration, the applied gas would either be pure He or Ar, or it may have trace amounts of Xe and Kr gases. The sparge gas tests would involve bubbling the gases through the salt. These cover and sparge gas tests would require a manifold of mass flow controllers to permit specific mixtures and flow rates to be applied to the sample.
In both continuous flow and vented tests, the salt cartridge outlet would direct gas out of the reactor channel to a sensor test bed. The sensor test bed would be located in a shielded area but would rely on the remote deployment capabilities of the proposed techniques. LIBS would be deployed using fiber optics. There have been many studies in the last decade developing reliable fiber-optic–LIBS (FO-LIBS) systems to leverage the technique in hazardous areas or areas with limited space (Fobar et al., 2018; Xiao et al., 2018; Nakanishi et al., 2020). Many of the successful studies have found that using the natural harmonic (1,064 nm) of the Nd:YAG laser permits better launching of the laser pulse into the fiber. Typically, larger core silica fibers (∼1,000 µm) or hollow-core fibers have been used with success (Rajavelu et al., 2022). On the sample end of the fiber, a simple two lens system would be used; the first lens would collimate the laser pulse from the fiber and then the second would focus it into the gas line to form the LIBS plasma. The emitted plasma light would be collected with lenses into a secondary fiber and routed to a spectrometer for measurement. Previous studies have shown that there is little effect to the LIBS signal from changes in flow rate causing misalignment of the collimating optics (Andrews and McFarlane, 2021). The use of a second fiber for light collection mitigates issues associated with internal laser damage to the fiber interfering with the light collection efficiencies.
LIBS requires a line of sight to the sampling point; however, a fraction of aerosols in the carrier gas would plate out on windows, degrading the optical system over time. Previous work has demonstrated the use of a sheath gas across the measurement region to protect optical lenses and windows (Andrews and Myhre, 2022). However, given the size of the proposed test system, a simple sudden expansion across the sampling area may suffice to prevent any aerosol particles from spreading within the region of concern (Williams, 2016). This would also remove the need for another continuous flow of gas. Lastly, the gas stream would then be passed through a series of treatment systems to remove any hazardous species before venting through the facility exhaust. An example schematic of the sampling setup is shown in Figure 2.
FIGURE 2. Example sensor test bed with a FO-LIBS setup being used to measure the flow across a sudden expansion. Fiber optics are used to prevent damage to sensitive equipment, such as the laser head and electronics.
The primary purpose of the conceptualized irradiation system is to provide a platform for producing conditions relevant for the development and testing of MSR off-gas sensors based on optical spectroscopy techniques. Four salt conditions will be possible using the irradiation system. These are (a) static liquid and static cover gas, (b) static liquid and flowing cover gas, (c) agitated liquid and flowing cover gas, and (d) agitated liquid and static cover gas. Testing condition (a) would be of interest to evaluate how salt species evolve from the salt passively under irradiation. The capsule would be closed during most of the test but would be vented occasionally to send the gas from the capsule headspace to the sensors. This would be representative of an MSR with a loss of flow. Testing conditions (b) and (c) seek to be more representative of an operating MSR where the cover gas is swept across the headspace to remove volatiles and when the salt is sparged with a gas to intentionally drive species into the headspace as a method of salt composition maintenance. In both these testing conditions, the capsule will constantly be sending a gas stream to the sensors. Lastly, testing condition (d) replicates a scenario where the off-gas system is static during reactor operation and all evolved species will remain in the core, or in this case capsule. Like in condition (a) the capsule headspace will be vented periodically for the sensors to test the headspace composition.
The myriad of MSR designs being pursued today offer an array of scenarios involving combinations of flowing or static molten salts as well as liquid or solid fuels. Various compositions for molten salt coolants and fuels include chlorides and fluorides. In all cases, questions arise related to what gaseous and aerosolized species will find themselves in the headspace. The development of sensors able to adequately answer those questions while salts are undergoing irradiation is critical. Note, the below experimental campaigns are aimed at being generally applicable and not specific to any single MSR design or even type. Instead, they are illustrative of the possible experiments with the conceptualized irradiation system for testing optical spectroscopy sensors. Future evolutions of the system concept would be designed to produce conditions (e.g., salt flow, cover gas flow, material interfaces, salt-surface-to-cover-gas ratio) that more closely replicate those expected in a specific reactor design.
The following subsections detail general sensing needs for MSR off-gas sensors that LIBS could address. Gaps in the research needed to progress these sensors are discussed.
Coolant and fuel salts will produce various levels vapors and aerosols that may be greatly increased based on the salt and cover gas conditions. Online sensors should be able to detect the primary constituents of fluoride- and chloride-based coolant and fuel salts. Common F salt nonfuel constituents may include F, Li, K, Na, and Be. Common chloride salt nonfuel constituents include Cl, Na, and Mg.
Figure 3 shows the expected LIBS emission profiles of these salt species, along with Ar and He as carrier gases. These spectra were generated using Saha–Boltzmann relations for a plasma in local thermodynamic equilibrium at a plasma temperature of 1 eV and an electron density of 1017 cm−3. These artificial spectra match well with our previous experimental work analyzing metal and graphite materials exposed to coolant salts with constituents (Keiser et al., 2022; Myhre et al., 2022). While the artificial spectra are useful for peak identification and experimental planning, they rely on reported transition probabilities which are lacking for some species (Andrews et al., 2022b). Thus, there are peaks not shown in the artificial spectra, which would be available for quantification in experimental spectra. As shown in Figure 3, each species has several unique emission lines across the ultra violet–visible–near infrared (UV-VIS-NIR) spectrum. LIBS has the benefit of emission peak surplus to better cope with highly complex spectra.
FIGURE 3. Fluoride- and chloride-salt species’ and carrier gas candidates’ LIBS emission profiles compared at a plasma temperature of 1 eV and electron number of 1017 cm−3. The spectral intensities have been normalized (0–1) to their maximum intensity for comparison purposes.
Figure 4 shows the relative intensities of the salt species’ most intense emission peaks as the plasma temperature is varied from 0 to 2 eV, while the electron density is held constant at 1017 cm−3. Typical LIBS plasmas vary between 0.5 and 1 eV, but the effective plasma temperature can be increased intentionally by raising the laser fluence and may act as a method to increase sensitivity to specific species. Further, analysis of spectra taken of plasmas with different effective temperatures could allow for enhanced deconvolution of overlapping spectral signals with nonlinear intensity dependencies on plasma temperature (see Figure 4) (Li et al., 2014).
FIGURE 4. Fluoride- and chloride-salt species’ and carrier gas candidates’ LIBS emission intensities versus plasma temperature (constant electron density = 1017 cm−3). The most intense emission peaks were selected for this comparison: F I 685.6 nm, Li I 670.7 nm, K I 766.7 nm, Na I 589.2 nm, Be II 313.2 nm, Cl I 837.8 nm, Mg II 279.6 nm, Ar I 811.8 nm, and He I 587.7 nm. The spectral intensities have been normalized (0–1) to their maximum intensity for comparison purposes.
Fuel materials may be U, Th, and Pu. The detection of these species in the off-gas could be indicative of the reactor salt conditions and would be essential for monitoring nuclear material inventory. LIBS has previously been demonstrated for the detection of these elements, and with high-resolution spectrometers, even the isotopic compositions may be determined (Cremers et al., 2012; Doucet et al., 2011; Chan et al., 2021). These fuel species have thousands of emission peaks in the 200–1,000 nm wavelength region and offer examples of the peak surplus benefit becoming a complication. Research studies to detect and model all relevant salt species in the presence of fuel species is needed. Transition probabilities for these species are either highly limited or completely unavailable, making artificial spectra impossible to produce. Future studies like those reported by Irvine et al. (2022) and Andrews et al. (2022b) will be needed to determine these fundamental properties.
Noble fission gasses are expected to have the lowest salt retention of fission products and build up in the headspace of the reactor. Xenon and krypton are of primary concern because they could significantly contribute to the overall need for off-gas processing. Their isotopes also have high neutron absorption cross sections and can significantly affect the reactivity in the reactor core. Thus, assuming that the noble gases are instantaneously released may not be conservative in reactor safety analysis. Changes in the distribution Xe and Kr between the salt and cover gas is therefore important to monitor in real time. The conceptualized system would allow for irradiation of both fueled and coolant salts. In the former Xe and Kr may be generated from fission, but in the latter, the sparge gas can be doped with Xe and Kr to investigate gas solubility in the salts. In both cases, the measurement of Xe and Kr in the cover gas would be of interest.
Figure 5 shows the expected LIBS emission profiles for Xe and Kr alongside the candidate cover gases of He and Ar, as calculated using Saha–Boltzmann relations. Most gaseous elements have transitions in the NIR region of the spectrum with more diffuse signatures, making their simultaneous detection a simpler task. Previous work has demonstrated LIBS can quantitatively measure Xe and Kr in real time and in the presence of other gases and aerosols (Andrews et al., 2022a).
FIGURE 5. Calculated emission spectra of Kr, Xe, He, and Ar at a plasma temperature of 1 eV and electron number of 1017 cm−3. The spectral intensities have been normalized (0–1) to their maximum intensity for comparison purposes.
The introduction of contaminants to coolant and fuel salts from material corrosion and mechanical wear is a major concern for MSRs. The conceptualized salt capsule may be loaded with material coupons—structural alloys, nuclear-grade graphite, and coated salt-facing materials. During corrosion, there is an exchange between salt species and the degraded material that results in salt penetration into the coupons and corrosion species entering the salt. These corrosion and salt impurity species (i.e., H, O, C, Si, Cr, Fe, Ni, Al, Mo, and the noble gases) may be generated in the salt or introduced by gas sparging. The detection of these species by spectroscopy sensors would be indicative another way the off-gas sensors can monitor the health of the reactor. If these species do not evolve, the test provides a neutron irradiated corrosion test that has only been rarely performed (Ezell et al., 2021).
The spectra of previously mentioned corrosion species calculated at a plasma temperature of 1 eV and an electron density of 1017 cm−3 are shown in Figure 6. The spectra show that metal and metalloid species have emission signatures largely in the UV-VIS region of the spectrum. These species typically have 10–20 strong emission peaks. Multivariate modeling can leverage these strong and also weaker peaks to individually quantify each species (Andrews et al., 2022a). The Balmer series Hα line at 656 nm is the largest emission peak for H. It is commonly used in LIBS research to estimate the plasma electron density by evaluating its Stark broadening (de Oliveira Borges et al., 2018). This may be used in models to better identify species’ signatures using Saha–Boltzmann calculations, such as those used to produce spectra in this paper.
FIGURE 6. Selected corrosion species’ LIBS emission profiles compared at a plasma temperature of 1 eV and electron number of 1017 cm−3. The spectral intensities have been normalized (0–1) to their maximum intensity for comparison purposes.
This paper presents an early-stage concept for an irradiation experiment capability with the primary purpose of developing optical spectroscopy sensors for analyzing cover gases relevant to MSRs. LIBS has been focused on as the sensor to be developed with such a system, though other optical spectroscopy sensors could also be developed. The concept has been designed to accommodate a range of reactor conditions with varying combinations of static or dynamic salt and cover gases. Approximate signals expected from LIBS measurements of aerosolized salts, fission products, and common impurities have been calculated to illustrate detectability for elements of major interest to the MSR community. These elements include coolant and fuel salt constituents, impurities, and gaseous fission products. Aerosolized coolant and fuel salts generated by turbulent flow through the reactor primary circuit could provide the opportunity to monitor the composition of the salts. An in-depth understanding of how representative the aerosolized salts are of the bulk liquid salt and the effect of constituent volatility on the aerosol stoichiometry would be necessary to unlock this potential. This study serves as a starting point for future experimental work. The potential challenges of convoluted emissions, lack of reported transition probabilities, and LIBS signal stability over time will need to be explored as these experiments are performed.
The raw data supporting the conclusion of this article will be made available by the authors, without undue reservation.
HA—Conceptualization, Writing—Original Draft, Writing—Review and Editing, Visualization, Supervision. KM—Conceptualization, Writing—Original Draft, Writing—Review and Editing. JM—Conceptualization, Writing—Review and Editing.
This work was funded by the US Department of Energy, Office of Nuclear Energy’s Advanced Reactor Development Program.
The authors declare that the research was conducted in the absence of any commercial or financial relationships that could be construed as a potential conflict of interest.
This manuscript has been authored by UT-Battelle, LLC, under contract DE-AC05-00OR22725 with the US Department of Energy (DOE). The US government retains and the publisher, by accepting the article for publication, acknowledges that the US government retains a nonexclusive, paid-up, irrevocable, world- wide license to publish or reproduce the published form of this manuscript, or allow others to do so, for US government purposes. DOE will provide public access to these results of federally sponsored research in accordance with the DOE Public Access Plan (http://energy.gov/downloads/doe-public-access-plan).
All claims expressed in this article are solely those of the authors and do not necessarily represent those of their affiliated organizations, or those of the publisher, the editors and the reviewers. Any product that may be evaluated in this article, or claim that may be made by its manufacturer, is not guaranteed or endorsed by the publisher.
Andrews, H. B., McFarlane, J., Chapel, A. S., Ezell, N. D. B., Holcomb, D. E., De Wet, D., et al. (2021a). Review of molten salt reactor off-gas management considerations. Nuclear Engineering and Design. 385, 111529. doi:10.1016/j.nucengdes.2021.111529
Andrews, H. B., and McFarlane, J. (2021). Characterization of surrogate molten salt reactor aerosol streams. Oak Ridge, Tennessee: Oak Ridge National Laboratory. ORNL/TM-2021/2205.
Andrews, H. B., McFarlane, J., Holcomb, D., Ezell, N. D. B., Myhre, K., Lines, A., et al. (2021b). “Sensor technology for molten salt reactor off-gas systems,” in Proceedings of the 12th NPIC&HMIT, Rhode Island, United States, June-2021, 723.
Andrews, H. B., McFarlane, J., and Myhre, K. G. (2022a). Monitoring noble gases (Xe and Kr) and aerosols (Cs and Rb) in a molten salt reactor surrogate off-gas stream using laser-induced breakdown spectroscopy (LIBS). Applied Spectroscopy. 76 (8), 988–997. doi:10.1177/00037028221088625
Andrews, H. B., and Myhre, K. G. (2022). Quantification of lanthanides in a molten salt reactor surrogate off-gas stream using laser-induced breakdown spectroscopy. Applied Spectroscopy. 76 (8), 877–886. doi:10.1177/00037028211070323
Andrews, H. B., Sadergaski, L. R., and Myhre, K. G. (2022b). Neptunium transition probabilities estimated through laser induced breakdown spectroscopy (LIBS) measurements. Journal of Analytical Atomic Spectrometry. 37 (4), 768–774. doi:10.1039/d1ja00423a
Chan, G. C. Y., Martin, L. R., Trowbridge, L. D., Zhu, Z., Mao, X., and Russo, R. E. (2021). Analytical characterization of laser induced plasmas towards uranium isotopic analysis in gaseous uranium hexafluoride. Spectrochimica Acta Part B: Atomic Spectroscopy. 176, 106036. doi:10.1016/j.sab.2020.106036
Cremers, D. A., Beddingfield, A., Smithwick, R., Chinni, R. C., Jones, C. R., Beardsley, B., et al. (2012). Monitoring uranium, hydrogen, and lithium and their isotopes using a compact laser-induced breakdown spectroscopy (LIBS) probe and high-resolution spectrometer. Applied Spectroscopy. 66 (3), 250–261. doi:10.1366/11-06314
Cremers, D. A., and Radziemski, L. J. (2013). Handbook of laser-induced breakdown spectroscopy. New Jersey, United States: John Wiley & Sons.
De Oliveira Borges, F., Ospina, J. U., De Holanda Cavalcanti, G., Farias, E. E., Rocha, A. A., Ferreira, P. I., et al. (2018). CF-LIBS analysis of frozen aqueous solution samples by using a standard internal reference and correcting the self-absorption effect. Journal of Analytical Atomic Spectrometry. 33 (4), 629–641. doi:10.1039/c7ja00299h
Doucet, F. R., Lithgow, G., Kosierb, R., Bouchard, P., and Sabsabi, M. (2011). Determination of isotope ratios using laser-induced breakdown spectroscopy in ambient air at atmospheric pressure for nuclear forensics. Journal of Analytical Atomic Spectrometry. 26 (3), 536–541. doi:10.1039/c0ja00199f
Ezell, N. D. B., Raiman, S. S., Kurley, J. M., and McDuffee, J. (2021). Neutron irradiation of alloy N and 316L stainless steel in contact with a molten chloride salt. Nuclear Engineering and Technology. 53 (3), 920–926. doi:10.1016/j.net.2020.07.042
Felmy, H. M., Clifford, A. J., Medina, A. S., Cox, R. M., Wilson, J. M., Lines, A. M., et al. (2021). On-line monitoring of gas-phase molecular iodine using Raman and fluorescence spectroscopy paired with chemometric analysis. Environmental Science & Technology. 55 (6), 3898–3908. doi:10.1021/acs.est.0c06137
Fobar, D. G., Xiao, X., Burger, M., Le Berre, S., Motta, A. T., and Jovanovic, I. (2018). Robotic delivery of laser-induced breakdown spectroscopy for sensitive chlorine measurement in dry cask storage systems. Progress in Nuclear Energy. 109, 188–194. doi:10.1016/j.pnucene.2018.08.001
Forsberg, C. (2020). Market basis for salt-cooled reactors: Dispatchable heat, hydrogen, and electricity with assured peak power capacity. Nuclear Technology. 206 (11), 1659–1685. doi:10.1080/00295450.2020.1743628
Hughey, K. D., Bradley, A. M., Tonkyn, R. G., Felmy, H. M., Blake, T. A., Bryan, S. A., et al. (2020). Absolute band intensity of the iodine monochloride fundamental mode for infrared sensing and quantitative analysis. The Journal of Physical Chemistry. 124 (46), 9578–9588. doi:10.1021/acs.jpca.0c07353
Irvine, S., Andrews, H., Myhre, K., Goldstein, K., and Coble, J. (2022). Radiative transition probabilities of neutral and singly ionized Europium estimated by laser-induced breakdown spectroscopy (LIBS). Journal of Quantitative Spectroscopy and Radiative Transfer. 286, 108184. doi:10.1016/j.jqsrt.2022.108184
Keiser, J. R., Singh, P. M., Lance, M. J., Meyer, H. M., Myhre, K. G., Lowe, T. M., et al. (2022). Interaction of beryllium with 316H stainless steel in molten Li2BeF4 (FLiBe). Journal of Nuclear Materials. 565, 153698. doi:10.1016/j.jnucmat.2022.153698
Li, C. M., Zou, Z. M., Yang, X. Y., Hao, Z. Q., Guo, L. B., Li, X. Y., et al. (2014). Quantitative analysis of phosphorus in steel using laser-induced breakdown spectroscopy in air atmosphere. Journal of Analytical Atomic Spectrometry. 29 (8), 1432–1437. doi:10.1039/c4ja00036f
Moon, J., McFarlane, J., Robb, K., McAlister, A., Braatz, A., Taylor, Z., et al. (2022). Measuring the solubility of xenon in molten chloride salt. Oak Ridge, Tennessee: Oak Ridge National Laboratory. ORNL/TM-2022/1.
Myhre, K. G., Andrews, H. B., Sulejmanovic, D., Contescu, C. I., Keiser, J. R., and Gallego, N. C. (2022). Approach to using 3D laser-induced breakdown spectroscopy (LIBS) data to explore the interaction of FLiNaK and FLiBe molten salts with nuclear-grade graphite. Journal of Analytical Atomic Spectrometry. 37 (8), 1629–1641. doi:10.1039/d2ja00168c
Nakanishi, R., Saeki, M., Wakaida, I., and Ohba, H. (2020). Detection of gadolinium in surrogate nuclear fuel debris using fiber-optic laser-induced breakdown spectroscopy under gamma irradiation. Applied Sciences. 10 (24), 8985. doi:10.3390/app10248985
Rajavelu, H., Vasa, N. J., and Seshadri, S. (2022). Hollow-core optical fiber-based laser-induced breakdown spectroscopy technique for the elemental analysis of pulverized coal. Applied Physics A. 128 (10), 868. doi:10.1007/s00339-022-06007-9
Riley, B. J., McFarlane, J., DelCul, G. D., Vienna, J. D., Contescu, C. I., and Forsberg, C. W. (2019). Molten salt reactor waste and effluent management strategies: A review. Nuclear Engineering and Design. 345, 94–109. doi:10.1016/j.nucengdes.2019.02.002
Serp, J., Allibert, M., Beneš, O., Delpech, S., Feynberg, O., Ghetta, V., et al. (2014). The molten salt reactor (MSR) in Generation IV: Overview and perspectives. Progress in Nuclear Energy. 77, 308–319. doi:10.1016/j.pnucene.2014.02.014
Williams, A. N. (2016). Measurement of rare earth and uranium elements using laser-induced breakdown spectroscopy (LIBS) in an aerosol system for nuclear safeguards applications. Richmond, United States: Virginia Commonwealth University.
Xiao, X., Le Berre, S., Fobar, D. G., Burger, M., Skrodzki, P. J., Hartig, K. C., et al. (2018). Measurement of chlorine concentration on steel surfaces via fiber-optic laser-induced breakdown spectroscopy in double-pulse configuration. Spectrochimica Acta Part B: Atomic Spectroscopy. 141, 44–52. doi:10.1016/j.sab.2018.01.003
Keywords: molten salts, optical spectroscopy, laser-induced breakdown spectroscopy (LIBS), radioactive, radiolysis, fission gasses, online monitoring, molten salt reactor (MSR)
Citation: Andrews HB, Myhre KG and McFarlane J (2023) Concept for an irradiation experiment to test a laser-induced breakdown spectroscopy off-gas sensor for molten salt systems. Front. Energy Res. 10:1100536. doi: 10.3389/fenrg.2022.1100536
Received: 16 November 2022; Accepted: 21 December 2022;
Published: 09 January 2023.
Edited by:
Palash Kumar Bhowmik, Idaho National Laboratory (DOE), United StatesReviewed by:
Md Shafiqul Islam, Dhaka University, BangladeshCopyright © 2023 Andrews, Myhre and McFarlane. This is an open-access article distributed under the terms of the Creative Commons Attribution License (CC BY). The use, distribution or reproduction in other forums is permitted, provided the original author(s) and the copyright owner(s) are credited and that the original publication in this journal is cited, in accordance with accepted academic practice. No use, distribution or reproduction is permitted which does not comply with these terms.
*Correspondence: Hunter B. Andrews, YW5kcmV3c2hiQG9ybmwuZ292
Disclaimer: All claims expressed in this article are solely those of the authors and do not necessarily represent those of their affiliated organizations, or those of the publisher, the editors and the reviewers. Any product that may be evaluated in this article or claim that may be made by its manufacturer is not guaranteed or endorsed by the publisher.
Research integrity at Frontiers
Learn more about the work of our research integrity team to safeguard the quality of each article we publish.