- 1Department of Physics, Anna University Regional Campus, Tirunelveli, India
- 2Department of Biotechnology, Central University of Tamil Nadu, Thiruvarur, Tamil Nadu, India
The growing necessity for energy worldwide has led to the hunt for an interminable solution in the form of sustainable energy generation. To accomplish sustainability, these problems can be resolved using renewable waste biomass, which is readily accessible and low priced. Moreover, the ecological issue due to the disposal of this waste biomass into the environment is also counteracted by the use of this biomass for energy generation along with the substantial solid reduction for disposal. The presence of complex biopolymers in biomass, which hasten the hydrolysis step during energy generation, was enhanced by the application of a pretreatment method. The efficiency of the pretreatment methods was enhanced by maintaining the cost and energy usage since the commercialization of this method is largely limited. The major economic drivers are based on solid concentration and, thus, lead to higher capital costs. This study reveals the wide assortment of current progression in pretreatment techniques for treating waste biomass with special focus on combined and phase-separated pretreatment. Additionally, it converses the advantages and limitations of pretreatment methods. This pivotal investigation brings about the cost- and energy-effective conversion solution that paves the way for a sustainable energy system.
1 Introduction
The expansion in population, along with the development of industrialization, affects the economic growth (Ahmed et al., 2021). Parallel to the economic point of view, the energy demand also increases with increasing population growth throughout the world, and the energy demand is expected to increase till 2040 (Ong et al., 2021). Globally, about one-third of the energy was used by buildings, transportation, and industrial sectors (G. Wang et al., 2020). In order to cope with this demand, fossil fuels, a non-renewable resource, were widely used, but due to their limited availability, they are incompetent for the growth of industrialization (W-H. Chen et al., 2021). Globally, fossil fuel usage was about 84 million barrels/day in the year 2019, and during the COVID-19 outbreak, it reduced to 16 million barrels/day due to the restriction in transport movement. From 2019–25, the demand for fossil fuels will be augmented by 5.7 million barrels/day (IEA, 2021). In the case of biofuel demand, the global increase was 28% per year during the forthcoming year 2026. Furthermore, the over usage of fossil fuels causes inconsistent prices for oils, generation of non-biodegradable waste, change in global climate, environmental degradation, and health issues (Nguyen et al., 2021; Yin et al., 2020). Thus, there is a need for an alternative source for the production of green energy, which counteracts the issues caused due to fossil fuel consumption (Rezania et al., 2020).
Biomass is one such alternative source, which is gaining more attention since it is an efficient form of energy and it is a fixed carbon reservoir (Papathoti et al., 2021). It is the organic matter which is derived from plant or animal origin and through any other natural means (Mehedintu et al., 2018). Annually, worldwide biomass production is accounted to be 130 billion tons (Sheldon, 2014). This biomass is transformed into energy, chemicals, and valued products by means of biochemical, physicochemical, and thermochemical processes (Adams et al., 2018), where the thermochemical method uses coupled exothermic energy in the form of heat and chemicals, the biochemical method uses bacteria and enzymes, and the physicochemical method uses a physical and chemical method for the conversion of biomass to biofuels (Mehrez et al., 2022). The energy generation by this biomass is considered to be an eco-friendly means with lesser environmental impacts (Shen et al., 2020). The biomass shares about 64% of lignocellulosic biomass (LCB), 24% of municipal solid waste, and 10% of agricultural and forest residues. The higher production cost for the bioenergy generated from LCB is considered to be a critical factor that hinders commercialization. Thus, technological advancement is needed to combat this issue. The energy produced from biomass accounts for 5 × 1019 kJ, and it is expected to be enhanced by 150 × 1019 kJ by 2050 based on the variety of biomass (Alper et al., 2020). The biomass raw material cost was reduced by blending the higher carbohydrate biomass with lower-cost biomass in order to accomplish the project cost by 2022 to $ 79.1 per dry ton.
Bioenergy production by biomass is a form of energy that offers a prospect for greenhouse gas reduction by exploiting biomass feedstock through effective technologies. The waste biomass can be converted to different forms of energy, such as hydrogen, methane, ethanol, methanol, and biofuels, by various processes (Bijarchiyan et al., 2020). So far, the highest hydrogen yield from waste-activated sludge (WAS) was reported to be 20.3 mg/g of volatile suspended solids (VSS) (Fu et al., 2021). There is a still a lack of idea about fermentative hydrogen production due to the lack of summarization and critical thinking. However, researchers have succeeded in improving the yield by disintegration methods and suppressing the methanogens which arrest hydrogen production (Liu et al., 2020).
The conversion technology of waste biomass to energy transforms the waste matter into different forms of biofuels that are used efficiently for energy supply. Worldwide, about 14% of bioenergy is consumed presently and is expected to be remarkable in the upcoming future (Souza et al., 2017). In addition, bioenergy production lessens the threat of energy demand and thus augments economic development (Schröder et al., 2018).
Due to the complexity of the structure of waste biomass, the efficiency of energy production is reduced. During the anaerobic digestion and fermentation method, the initial process is hydrolysis, where the complex polymers are converted to simpler monomers by means of hydrolytic enzymes, whereas due to the complexity, the hydrolytic enzymes are unable to act on it efficiently and thus result in rate-limiting step (Kavitha et al., 2017a; Kavitha et al., 2017b; Kavitha et al., 2019a; Kavitha et al., 2019b). This limitation was overcome by different pretreatment methods, which alter the structure of biomass and make it easily accessible for hydrolytic enzymes. Different pretreatment methods are physical, chemical, mechanical, and biological methods (Kannah et al., 2017a; Kavitha et al., 2017a; Kannah et al., 2017b; Kavitha et al., 2017b; Banu et al., 2019a; Banu et al., 2019b). This pretreatment method enhances the digestion efficiency by breaking down the biomass structure and thus solubilizing and discharging the internal substance to the liquid or soluble phase and converting the recalcitrant substance to the recyclable substance (Banu and Kavitha 2017). Pecorini et al. (2016) adopted autoclave and microwave methods to improve hydrolysis in municipal waste, resulting in improved yield of biogas production. Certain innovative pretreatment techniques methods such as static magnetic field and solidified carbon dioxide method also come into action, which helps in overcoming issues due to conventional pretreatment.
This review focused on understanding the structure of organic biomass that cause hindrance during the hydrolysis step in energy generation. Furthermore, different recently developed pretreatment methods and innovative methods were discussed. The energy and cost assessment during pretreatment were discussed expansively. This study further incorporated the existing research gaps in the recently developed pretreatment method and its impact on economic analysis. Lastly, future recommendations to fill these gaps were also discussed.
2 Exploration of various literature records
The article sourcing, screening, and analysis of different articles were carried out by following the method proposed by Thürer et al. (2018) through Web of Science, Google Scholar, and Scopus due to their higher content. The extensive literature survey was carried out based on a collection of books, but the relevant books for systematic review were not completely assessed. To keep the article reasonable and to confirm the source quality, further research was limited to peer-reviewed journal articles. The major aim of this literature review is to retrieve information on different pretreatment methods and their advantages, along with the cost and energy aspects of sustainable energy production. Moreover, the research gaps and future recommendations were also included. The search was limited to 20 years, ranging from 2002 to 2022. The keywords used for the search are ‘Biomass AND pretreatment AND bioenergy’; ‘Pretreatment AND bioenergy AND cost’; ‘Pretreatment AND bioenergy AND energy usage’; ‘Sustainable AND biomass AND bioenergy.’ In order to keep the search in consistent number, the search was limited with respect to title, abstract, keywords, document type, and highlights. Based on the search terms, 469 articles were retrieved overall.
The screening was carried out by reducing the sample after eliminating inappropriate and recurrent articles, and 194 articles were used in this review. In order to ensure the missing references, all the references in the articles were cross-checked. The systematic methodology of this literature review is presented in Figure 1.
While analyzing this article, the extraction and documentation of all the articles were performed based on the set of research questions, which designates the detail for this review. The research gap was described by refining the pieces of literature, and the questionnaire of research was given as follows:
• What are the different types of organic biomass?
• Is it possible to obtain energy from organic biomass?
• What are the major inhibitors that cause hydrolysis of organic biomass?
• What are the uses of different pretreatment methods?
• Do energy and cost analyses make the pretreatment feasible for the pilot scale?
• Is there any existing research gap in pretreatment methods?
• What are the different future perspectives that emerge from these papers?
By refining, this literature was used for this review and discussed.
3 Bioenergy production from waste biomass
Presently, due to the environmental and economic conditions, there is a need for energy saving and recycling. Bioenergy serves as an essential part of the energy economy and accounts for 70% of the supply of renewable energy (Reid et al., 2020). Different technologies were utilized and established for the usage of waste biomass for the production of bioenergy. This conversion aids the transformation of waste organic matter into different forms of fuels, which can have the ability to supply energy efficiently (Lee et al., 2019; Zamri et al., 2021). The selection of proper conversion technology lies in factors such as type, quantity, feedstock availability, environmental and economic conditions, and end-use applications (Mohammed et al., 2013). The International Energy Agency defines biomass as the organic matter that comes from biogenic sources and is renewable. It is divided into primary, secondary, and tertiary waste biomass. The primary biomass was generated by the food crops and forest residues, and it includes straw, corn stalks, and leaves. Secondary biomass is the residues due to food processing waste and agricultural residues, and the tertiary residues include the biomass generated due to human consumption of organic matter from a domestic source, which is further converted to sludge and wastewater (Chen et al., 2015). Apart from waste feedstock, microalgae and macroalgae were also considered to be favorable potential (Lee et al., 2019). For energy generation, biomass must be provided at a reasonable cost with minimum environmental impact. Figure 2 illustrates the different bioenergy feedstock for sustainable bioenergy production. Among different bioconversion techniques of these waste biomass for bioenergy production, the biochemical process was considered to be effective, which includes anaerobic digestion (AD) and fermentation techniques. AD is a biochemical process where the transformation of organic matter to biogas occurs by means of the presence of microorganisms in oxygen-limiting conditions (Feki et al., 2020). The produced biogas consists of the concoction of methane and carbon dioxide besides nutrient prolific effluent (Banu and Kavitha, 2017; Rajak et al., 2020). It generally consists of four stages, namely, hydrolysis, acetogenesis, acidogenesis, and methanogenesis. Four sets of bacteria act at different steps. In the first step, the hydrolytic enzymes secreted from microorganisms convert the polymer to monomers, and in the second step, the acetogenic microorganism converts these monomers to volatile fatty acids; the third step involves acidogenic bacteria, which converts the released VFA to hydrogen and acetate and finally, the methanogenic microorganisms convert the hydrogen ad acetate to CO2 and CH4 (Banu and Kavitha 2017; Bhatia et al., 2020). In fermentation techniques, the reaction ceases till the fermentation step of the acidogenic and acetogenic phase ad produces H2. The initial step of hydrolysis shows remarkable significance in eminent organic matter and thus turns out to be the rate circumscribing step in AD (Shanthi et al., 2018; Kavitha et al., 2019a; Kannah et al., 2019; Kannah et al., 2021). The utilization of raw material for AD or fermentation makes it difficult to handle due to its complexity and rate-limiting hydrolysis step. These curbs were shattered by the utilization of efficient pretreatment techniques, which disintegrate the biomass efficiently and, in turn, cause a lesser digestion period and efficient bioenergy production.
4 Necessity of a pretreatment method
Pretreatment enhances the availability of the substrates for microbes and the rate of reaction; thus, the degradation of organics also increases during the digestion process (Atelge et al., 2020). The pretreatment process enhances the surface area of the substrate for the subsequent treatment process and thereby increases the hydrolysis steps (Sharmila et al., 2020a). A biochemical reaction is enhanced by selecting efficient raw material for biological reaction and the mass transfer reaction for bioenergy yield (Cesaro and Belgiorno, 2014).
In waste-activated sludge (WAS), the presence of sludge flocs in the form of extracellular polymeric substance (EPS) around the cell wall of microbes affects the hydrolysis process (Eswari et al., 2017; Kavitha et al., 2018). It can be enhanced by the pretreatment process, where the floc structures are disrupted and cleave the inner and outer EPS layer solubilization; thus, simplified organic matter takes place (Junior et al.,2020). It promotes the particle size distribution and increases surface area and, thus, in turn, enhances the enzymatic process and degradation of organic matter. The EPS destruction leads to the release of entrapped water and thus also improves the dewaterability of sludge (Wei et al., 2018).
In LCB, the presence of lignin and hemicellulose restricts the cellulose conversion to about 20% only to sugar (Tian et al., 2018). Thus, the fragmentation of this structure is necessary for the bioconversion of this matter to the final product. The pretreatment process dissolves the hemicellulose and lignin, reduces particle size and cellulose crystallinity, and thus expands the biomass surface area (Karimi and Taherzadeh, 2016; Rezania et al., 2020). Furthermore, cellulose hydrolysis enhances the accessibility of enzymes to the cellulose surface (Kumari and Singh, 2018). Irrespective of the biomass type, the pretreatment method serves as an important factor for the conversion both in terms of economic and technical aspects (Mupondwa et al., 2017). The operating and capital cost of pretreatment must be above 40% of the total processing cost (Bhutto et al., 2017).
The biomass microalgae or macroalgae cell wall consists of lipids, carbohydrates, and protein, and it may vary based on the species and algal cultivation (Bernaerts et al., 2019). The organic compounds in the cytoplasm surrounding the cell wall of algae increase the thickness of the cell wall and thus lead to lower biodegradable characteristics (Sankaran et al., 2020). Pretreatment aims at the solubilization of cell walls and the conversion of complex cell wall components to the simpler monomer and makes it accessible for further enzymatic hydrolysis (Costa et al., 2020). It improves energy production by conquering the recalcitrant behaviour of biomass structure and reducing particle size and crystallinity index (Zabed et al., 2017).
The structural alterations of biomass were carried out through different pretreatment methods, namely, single, combined, and phase-separated pretreatment methods. Figure 3 depicts different pretreatment techniques for enhancing enzymatic hydrolysis.
4.1 Single pretreatment
Single pretreatment methods enhance the degradability of waste biomass by enhancing the surface area. These pretreatment methods are broadly classified as physical (thermal and microwave), chemical (acid and alkali), mechanical (disperser and sonication), and biological (bacteria and enzymes) (Atelge et al., 2020; Sharmila et al., 2020b). Table 1 shows the various combined and phase-separated pretreatment methods responsible for bioenergy production.
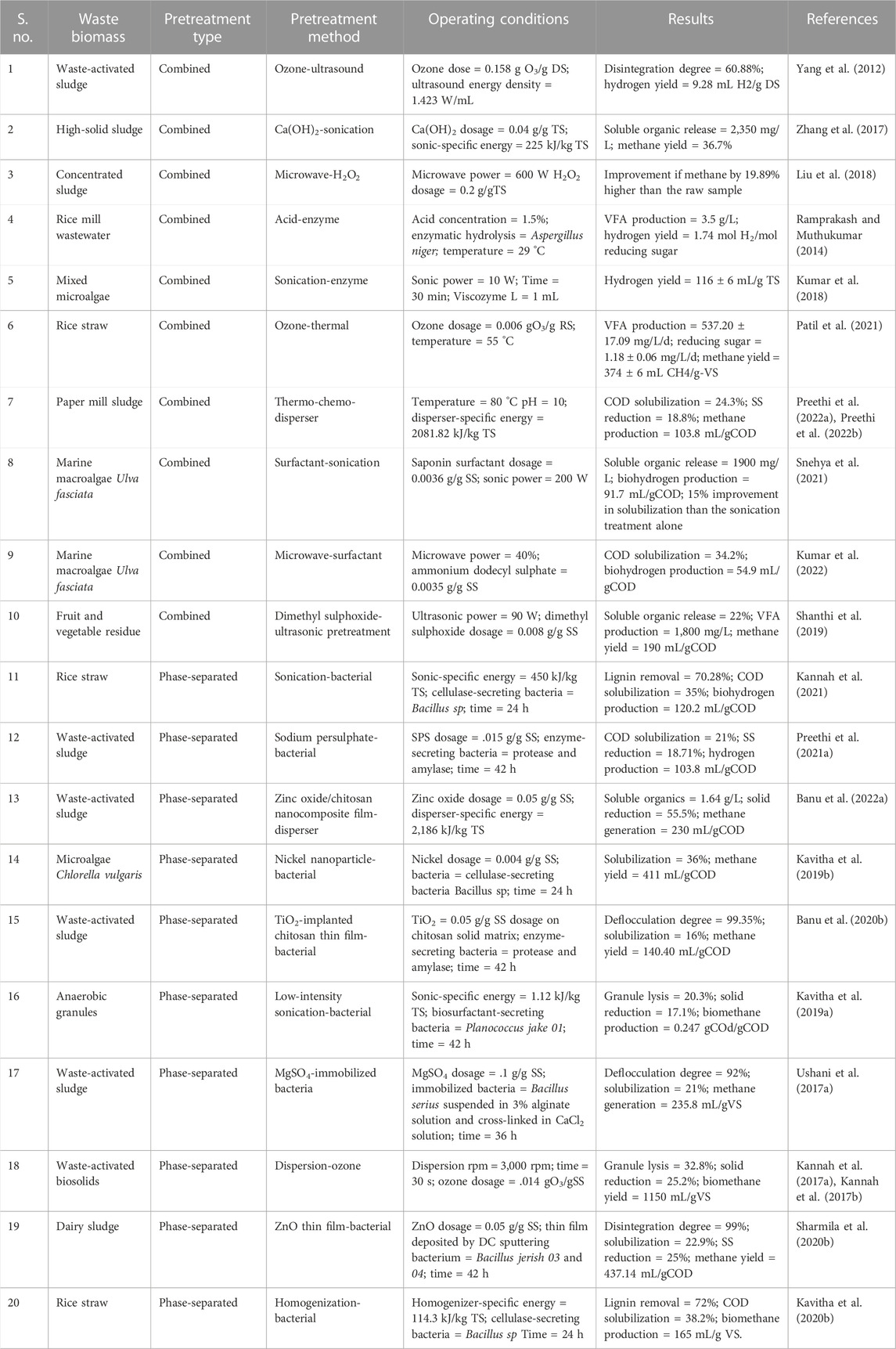
TABLE 1. Different combined and phase-separated pretreatment methods responsible for bioenergy production.
4.1.1 Physical
In physical pretreatment methods, there is no contamination due to chemicals, and it improves the energy yield. In a physical method, the variation in temperature enhances the solubilization of the substrate. It increases the surface area and augments the substrate and microorganism contact. Furthermore, the decrement in particle size declines the viscosity (Atelge et al., 2020), whereas the drawback of this method is the usage of higher energy. The physical pretreatment is classified as thermal and microwave (MW) pretreatment.
In the thermal pretreatment method, the variation in insoluble organic matter to liquefiable phase occurs due to the application of heat and thus helps in increment in substrate biodegradability (Zhou et al., 2015). The optimum temperature during this pretreatment method ranges between 150°C–180°C at a pressure of 600–2,500 kPa (Elalami et al., 2019). In this treatment, swelling in the substrate occurs due to the effect of temperature and pressure; thus, breaking the hydrogen bonds in the substrate leads to enhancement in surface area. In the thermal pretreatment method, the usage of low-temperature treatment reduces one of the drawbacks of the physical method, i.e., energy usage (Kavitha et al., 2017a). In the study by Rajput and Visvanathan (2018), a higher biogas yield of 53% was achieved at 180°C due to the alteration in chemical bonds in wheat straw.
MW pretreatment is also a type of physical pretreatment method where it operates at a wavelength of nearly 1–1 mm and with an operating frequency of 300–300 GHz (Aguilar-Reynosa et al., 2017; Liang et al., 2021). It has the advantage of a faster heating rate, higher thermal efficiency, shorter disruption time, higher efficiency, and 3D heat transfer. It was proven that the frequency of 2,450 MHz breaks the cell walls of microbes, disturbs the complex structure of biomass, and improves solubilization (Toreci et al., 2010; Chaturvedi and Verma, 2013). The irradiation causes the absorption of MW, the vibration of dielectric and polar molecules leads to the generation of heat, and pressure causes the biomass to release the intracellular components and solubilize the biopolymers (Mottet et al., 2009). The sCOD to TCOD ratio was increased to about 3.2 times when the primary and secondary sludge underwent MW pretreatment (Park and Ahn, 2011). It was reported that the specific energy input enhances the liquefaction of biomass and affects methane generation since it was considered to be one of the major factors (Passos et al., 2014c; Banu et al., 2019a). Neumann et al. (2016) showed that the MW SE input of 336,000 kJ/m3 leads to an improvement in biogas production from 16% to 50%, whereas the SE input of 20,000 kJ/kg TS shows an increase in methane production of 20%. Due to the higher energy consumption of energy in MW pretreatment, the pretreatment cost increases. Moreover, the formation of inhibitory compounds at higher temperatures occurs, and it can be minimized by circumventing higher power utilization during the treatment process.
4.1.2 Chemical
The chemical method is one of the methods that are more effective than any other single process due to the ability to enhance the complex substrate (Olatunji et al., 2021). It has gained greater attention due to its faster result and better efficiency for the degradation of complex organic matter (Hernández-Beltrán et al., 2019). The chemical pretreatment depends on the characteristics of feedstock, chemical types, and application. In LCB, this treatment method improves the availability of carbohydrates by eradicating lignin and diminishing the degree of polymerization and crystallinity of cellulose (Behera et al., 2014). In WAS, the chemical and microbial interaction promotes the solubilization of cell walls and favors enzymatic hydrolysis (Junior et al., 2020). It further reduces the HRT and enhances biogas production by improving soluble organic matter (Shrestha et al., 2020). The major disadvantage of this method is the increase in operation and maintenance costs due to the chemical purchase, and it cannot be recovered at the end of the process (Ariunbaatar et al., 2014). The major chemicals which are used for efficient pretreatment are acid, alkali, Fenton, and ozonation pretreatment.
In the alkali pretreatment method, the pH plays a crucial role which has a direct effect on the metabolic pathways of microorganisms. Some of the alkali solutions that are widely used for biomass pretreatment are sodium hydroxide, potassium hydroxide, magnesium hydroxide, lime, and ammonium hydroxide. The strong effect of alkali leads to selective lignin removal in LCB without any effect on carbohydrates and thus enhances the porosity and surface area by swelling reaction and easing the subsequent enzymatic hydrolysis (Kim et al., 2016). In sludge or microalgal biomass structure, the addition of alkali mediates different reactions, such as saponification, which results in biomass cell cleavage and thereby leads to better solubilization (Banu et al., 2018b).
Acid pretreatment does not gain as much attention as alkali pretreatment, whereas it is effective in treating LCB biomass, since it breaks lignin effectively (Neumann et al., 2016). Hydrochloric acid, sulphuric acid, nitric acid, and orthophosphoric acid were used as efficient agents for biomass pretreatment (Rorke and Kana, 2016). In LCB, the carbohydrate–lignin link breaks and recovers, reducing sugars (Gonzalez et al., 2016). The major advantage of this method is the efficient disruption and cellulose conversion, whereas the disadvantage is that the acid recovery cost is higher and has a higher chance of recalcitrant formation (Solarte-Toro et al., 2019). The hydrogen yield was enhanced by 1.24 molH2/mol glucose when the phosphoric acid yield of 0.8% was achieved during pretreating palm oil mill effluent (Mahmod et al., 2017).
In ozonation, pretreatment is an advanced oxidation process (AOP), and there is no chemical usage. It does not generate any odors, and no pathogens remain after the pretreatment of the substrate. It is a strong oxidizing agent, which is unstable and causes partial oxidation of the substrate (Atelge et al., 2020). It works by a direct and indirect reaction due to hydroxyl radicals and disrupts the cell membrane and releases the intracellular matter to a soluble medium, and increases sludge biodegradability (Gonzalez et al., 2018). In LCB, the ozone treatment aims at lignin reduction and does not have any effect on cellulose and hemicellulose structure. The methane yield was 66% when the microalgae were pretreated with ozone (Cardeña et al., 2017).
Fenton oxidation is also a type of AOP process, where the oxidation occurs due to the generation of hydroxyl radicals due to the decomposition of hydrogen peroxide and ferrous ions occurring during the acidic condition (Matavos-Aramyan and Moussavi, 2017). The hydroxyl attacks the organic matter without any recalcitrant production (Atay and Akbal, 2016). The hydrogen peroxide strength, time, pH, and temperature have a greater impact on this pretreatment. A study by Pilli et al. (2016) reported that the methane yield of 496 m3 CH4/ton VS. was achieved when the secondary sludge was exposed to the Fenton dosage of 0.07 gFe2+/gH2O2 and 60 g H2O2/kgTS at pH 3. The increase in H2O2 concentration leads to the scavenging of hydroxyl radicals, thereby lowering the concentration (M. Zhang et al., 2019). Due to the increase in acidic conditions, the neutralization of biomass is necessary before digestion, thus leading to the increased pretreatment cost (Junior et al., 2020).
4.1.3 Mechanical
This type of pretreatment intensifies the surface area, substrate porosity, and bulk density, which thereby enhances the linkage between feedstock and microbes. In LCB, the crystallinity of biomass reduces due to the particle size reduction and thus leads to the alteration in biomass structure (O’Dwyer et al., 2008). In WAS, the EPS structure and the cell membrane were broken down by the shear force created by an external force. In microalgae, the cell wall is broken by the same mechanism as that of WAS, whereas the presence of inert material in the substrate affects the microalgal disruption efficiency. The disadvantage of this method is its higher cost and energy usage. The popular mechanical pretreatment processes were ultrasonication and disperser pretreatment.
Ultrasonication accelerates the reaction for the bioactive compound extraction. Ultrasonication is considered to be an eco-friendly and cost-effective approach, as described by Menon et al. (2016). It involves the projection of sound waves through an aqueous medium and generates compression and rarefaction. It, in turn, changes pressure and produces cavitation due to the formation of bubbles in an elastic medium. The cavitation produces OH radicals that degrade the substrate efficiently (H. Wang et al., 2018). The major drawback of ultrasonic pretreatment is the higher energy cost (Yusaf and Al-Juboori, 2014). Thus, the specific energy input is one of the important parameters for the analysis of the solubilization efficiency of biomass. The study shows that the lower specific energy input enhances biogas production by 40%, whereas the moderate specific energy increases it by only 15% (Appels et al., 2008). In a study by Lizama et al. (2017), the specific energy input of 35000 kJ/kg TS shows an increase in biogas production by 31.4%. Moreover, the VFA concentration also enhances to 233.96 mg/L. In contrast, the study by Mirmasoumi et al. (2018) shows higher methane production of about 34% at the specific energy input of 14000 kJ/kg TS, and the methane generation is efficient in overcoming about 80% of sonic energy consumption.
Disperser pretreatment is a type of mechanical pretreatment where it works on the rotor–stator effect, which persuades the shear stress and thrust on sludge biomass. The disintegration occurs due to the high pressure, which results in sudden pressure change causing cavitation, turbulence, and shear force (Kannah et al., 2017b). This then interrupts the cell membrane and retrieves intracellular components. The maximum solubilization was achieved at disperser-specific energy of 8,547 kJ/kg TS, as in the study by Sethupathy and Sivashanmugam (2018).
4.1.4 Biological
It is one of the significant methods which utilize fungal, bacterial, or enzymes. The major advantage of this method is the operation stability in a shorter time and lower nutritional need (da Silva Machado and Ferraz, 2017; Saha et al., 2016). It breaks down the structures present in biomass with the action of enzymes. The major bacteria such as Clostridium sp., Cellulomonas sp., Bacillus sp., and fungi, namely, Trichoderma reesei, Trichoderma viride, and Aspergillus niger, were widely used in biomass pretreatment (Sharma et al., 2019). This type of pretreatment in LCB attack the structure of biomass by means of microbial means, thus disrupting crystalline structure, removal of lignin, and reducing carbohydrate loss. Generally, the cellulolytic and hemicellulolytic microorganisms act efficiently in LCB for the hydrolysis of carbohydrates to reduce sugar (Wagner et al., 2018). The lower rate of hydrolysis is the major obstacle to the biological pretreatment method.
A study by reported Dollhofer et al. (2018) found that biogas production was accelerated by faster degradation of dry matter and enhanced VFA concentration while utilizing fungi Neocallimastix frontalis for the pretreatment of LCB. Likewise, in another study by Yin et al. (2016), the substrate WAS is pretreated by fungal mash, which enhances the sludge hydrolysis and increases the methane yield by 53%.
The enzymes such as lipase, cellulase, protease, and amylase were used to pretreat organic biomass (Kavitha et al., 2013; Kavitha et al., 2017a; Kavitha et al., 2017b; Kavitha et al., 2017c; Preethi et al., 2022a; Preethi et al., 2022b). In a study by Odnell et al. (2016), methane production was enhanced by 37% when the hydrolytic enzyme protease was used to treat WAS. Likewise, in a study by Bonilla et al. (2018), about 26% of biogas was achieved due to the pretreatment by protease enzyme secreted by Bacillus licheniformis.
The single pretreatment method does not provide the anticipated result since it is much limited due to the functional mode as well as having its own disadvantage. Thus, it will be effective to adopt combined and phase-separated pretreatment methods for organic biomass disintegration for energy production.
4.2 Phase-separated pretreatment
Phase-separated pretreatment consists of two different phases, where the first phase removes the outer membrane and exposes the inner membrane for disintegration by the second method. This phase-separated pretreatment helps in the acceleration of solubilization and methane production.
In WAS, the solubilization of organic matter was improved by the fragmentation of the EPS layer. After the fragmentation of this EPS layer, the disintegration of the cell membrane was boosted by endorsing improved nutrient availability (Banu and Kavitha, 2017; Guo et al., 2022). EPS is the layer formed due to microbial adherence as flocs and consists of protein, carbohydrate, and nucleic acid with bound and extractable EPS (Nouha et al., 2018; Cui et al., 2020). The EPS layer was extracted through sodium citrate at the dosage of 0.1 g/g SS, enhancing the MW pretreatment efficiency and the methane generation, as in the study by Ebenezer et al. (2015). The EPS disruption by low-temperature thermo-chemical pursued by bacterial pretreatment was demonstrated by Kavitha et al. (2017a). It was found that the LB and TB-EPS solubilize efficiently to the aqueous phase with the lesser energy usage of 0.06 kJ/L, and the antibiotic-secreting bacteria tear the peptidoglycan layer of the sludge biomass and shift the intracellular components to the soluble phase.
In microalgal biomass also, initially, the first phase weakens the cell wall exopolymers and improves the pretreatment efficiency and biodegradability of the substrate. The cell wall of Chlorella vulgaris is rigid and thus resists the degradability of biomass. This issue was conquered by the cell wall weakening by nickel nanoparticle before the disintegration by bacteria, as in the study by Kavitha et al. (2019b), and it was found that the nickel nanoparticle of 0.007 g/g SS shows an efficient weakening of cell wall without any cell lysis and enhances the surface area for tailed bacterial disintegration.
In LCB, the presence of lignin inhibits the hydrolysis process, and thus the removal of this component is necessary to expose the cellulose and hemicellulose components for further pretreatment. Kavitha et al. (2020b) demonstrated the effect of the phase-separated pretreated process on rice straw and found that the mechanical dispersion removes lignin efficiently by 72% at 114.3 kJ/kg TS and enhances the mass transfer of cellulose by cellulase secreting bacteria at 24 h The delignification of straws leads to the COD solubilization of 38.2% and the final lignin content of .5 g/L, which does not cause any effect to methanogenic microorganisms and the methane generation were 165 mL/gVS. The phase-separated pretreatment, thus, arouses the organic matter with consequent augmentation of organics for bioenergy production.
4.3 Combined pretreatment
All the single pretreatment methods have their own disadvantages, due to which there is some negative effect on methane production, such as higher energy demand and the formation of some recalcitrant substances. In order to counteract these issues, the pretreatment methods were combined to enhance the efficiency of hydrolysis. The pretreatment methods were selected concurrently and successively and were analyzed for biogas production. This combined pretreatment reduces the operational cost without jeopardizing pretreatment efficiency. Based on the method of cell disintegration and the device usage, the extracted lipid components were assessed. In microalgal biomass, the combined pretreatment works efficiently for the production of soluble components. The mixed microalgal biomass was pretreated with chemomechanical pretreatment, where 5% (w/w) HCl and 7186 J/gTS sonic energy showed a decrease in methane yields due to the inhibitory action, as in the study by Juárez et al. (2018). Tamilarasan et al. (2018) combined disperser and thermochemical pretreatment for the treatment of substrate macroalgae Chaetomorpha antenna and showed that the efficient methane production of .215 m3CH4/kg VS. was achieved at pH 11, 80°C and disperser-specific energy of 800 kJ/kg TS. Likewise, in WAS, the surfactant and ultrasonic combination shows higher solubilization of about ten times higher than raw sludge at .05 g/g SS dioctyl sodium sulphosuccinate and 160 W of ultrasonic power (Ushani et al., 2017b).
The MW pretreatment alone is not enough for the hydrolysis of organic biomass since the MW effect suppresses due to the EPS layer present in sludge flocs (Ambrose et al., 2020). The combination of calcium peroxide (CP) and MW shows higher solubilization and leads to methane generation by 80.2%, as in the study by Wang and Li (2016). Moreover, a higher acetic acid content of 55.9% was achieved at the .1 g/g VSS of CP and 480 W MW power. Several studies focus on the combination of mechanical and alkaline pretreatment for the enhancement of COD solubilization. It was proved in the study by Babu et al. (2021) that sonication at pH 12 shows enhanced solubilization due to the floc breakage, leads to a more open structure, and promotes better disintegration by OH radicals.
The combination of biological and other treatment methods also shows greater efficiency when compared to single methods alone (Ponnusamy et al., 2019). The lower rate of hydrolysis and higher process time during biological treatment can be enhanced by the combined method (Shirkavand et al., 2016). It was reported in a study by Mustafa et al. (2017) that the combined biological and physical pretreatment of rice straw increases the methane yield by 165%. In contrast to this, a study by Gruber-Brunhumer et al. (2015) showed that the physical and biological pretreatment combination on microalgae increased biogas production by 64%.
The major advantage of this method is the reduced usage of chemical concentration; mild mechanical treatment is enough to reduce particle size, and lower temperature usage yields higher efficiency (Hernández-Beltrán et al., 2019). Thus, a proper combination of pretreatment is necessary for achieving efficiency in terms of economic and energy aspects.
4.4 Innovative techniques
Some of the disadvantages of the aforementioned methods are the higher energy demand, chemical requirements, process conditions, and time. Thus, in order to overcome these issues, some of the innovative approaches developed for the production of bioenergy out of biomass feedstock are static magnetic field (SMF), low-temperature conditioning by solidified carbon dioxide, and non-thermal plasma pretreatment.
SMF is widely used in the biodegradation of organic matter and the separation of solids and liquids by altering the property of fluids due to the variation in polarization and electric charge (Dębowski and Zieliński 2020). About 30% enhancement in biodegradation was observed when the magnetic south pole strength of .45 T was applied to immobilized microbes. The improvement in metabolic activity and cell growth of microorganisms was improved at a lower frequency of magnetic field for the enhancement in bioenergy production (Zhao et al., 2020). Zieliński et al. (2021) used higher organic dairy wastewater for the production of energy by means of SMF methods and found the domination in Trichococcus sp, methanogenic bacteria, and hydrogenotrophic methanogens, which enhances the methane content to 56.8%. Furthermore, they found an abundant increase in Lactobacillales, which helps in the growth of methanogens.
Solidified carbon dioxide or dry ice pretreatment can efficiently remove the flocculated structures in WAS by forming large particles by agglomeration and thus release the bound water. The destruction of the microorganism cell wall at freezing temperature is still developing. Moreover, several factors depend on the destruction of microbes in WAS, such as chemical composition, freezing time, freezing temperature, and the rate of freezing. This method helps in dissociating floc structure and cells by forming ice crystals surrounding the cell walls, increasing the cytoplasmic water content and damaging the cell wall by osmotic shock, decreasing the strength of RNA and DNA, and thereby inducing cold death of microorganisms (Machnicka et al., 2019). A study by Kazimierowicz et al. (2021) revealed that the chemical oxygen demand enhanced to 490.6 ± 12.3 mg dm−3 when the dry ice to WAS ratio was .3.
Non-thermal plasma pretreatment is a form of advanced oxidation, which is effective in reducing the recalcitrant substance in LCB by removing lignin (Ravindran et al., 2019). It works by initiating the electrical discharge with sufficient voltage. This electrical discharge leads to an increment in electron temperature than the surrounding gas. The interface between gas molecules and electrons leads to the generation of reactive radicals and ions, thereby causing the alteration in biomass structure.
5 Energy analysis of pretreatment methods
The major input which decides the economical and eco-friendly aspects is energy, and it expresses the applicability of the pretreatment process for the pilot scale. In order to achieve profit, it is necessary to match energy utilization with energy production in terms of bioenergy. In India, fossil fuels meet the energy demand of about 70% of the population of 1.5 billion (Golembiewski et al., 2015). In the conventional incineration process, about 15% of energy was generated in the form of power, as in the study by dos Santos et al. (2020). Likewise, the pyrolysis of one tonne of municipal solid waste (MSW) for oil production shows a carbon emission of 204.33 kg CO2eq/tonne MSW (Chhabra et al., 2021). The pretreatment of the waste biomass is necessary to release the intracellular components to the aqueous phase for enhancing biodegradability, and the pretreatment method was divided into two types based on energy demand: energy input and energy output. The input energy during the pretreatment step has a major environmental effect, and thus the optimum treatment condition is necessary to barter the biogas generation enhancement and energy exhaustion of the pretreatment method (Atelge et al., 2020). The energy exploited is dependent on the method of pretreatment, operating conditions, and the device used. The major energy-consuming pretreatment methods for the solubilization of biomass in the form of electricity were sonication, disperser, thermal, MW, etc. (Cano et al., 2015; Eswari et al., 2017; Banu et al., 2019a; Banu et al., 2019b; Kumar, 2019; Kavitha et al., 2020a). A study by Pilli et al. (2011) shows the evaluation of sonic pretreatment in terms of solubilization, biopolymer assessment, particle size, and microbial action. The energy parameter considers the pretreatment alone and does not account for any interface between the wastewater treatment plant and pretreatment (Cano et al., 2015). The energy balance is an important factor in assessing the economic feasibility of the pretreatment process. In order to balance the energy, energy input and output needed to be evaluated. Table 2 demonstrates the cost and energy analysis of various pretreatment methods.
5.1 Input energy
The energy balance was calculated based on the input energy calculation required for pretreatment and bioenergy generation. The energy-demanding pretreatment process usually demands higher electricity or heat in order to fragment the microbial cells in waste biomass. In order to make the pretreatment process feasible for the pilot scale, the optimization of different pretreatment parameters and energy usage is necessary. The electricity-demanding pretreatment process is a sonication, disperser, and MW method for efficient reduction of particles present in biomass and enhanced solubilization (Kannah et al., 2017b; Banu et al., 2019b; Kannah et al., 2021). The energy consumed for this pretreatment method was calculated as
where EEp is the energy spent during the pretreatment process in kWh/kg; P is power expended during pretreatment in kW; T is the time desired for pretreatment in sec; Vis the volume of sample in m3; and TS is the total solid concentration in kg/m3.
According to Darji et al. (2014), MW is an energy-competent method where lower input of power is needed. In contrast to this, Kostas et al. (2017) stated that the power consumption is higher during MW pretreatment. The pretreatment time during MW was reduced to ten times, as in the study by Xia et al. (2013). In the combined microwave and dilute acid pretreatment of water hyacinth, the energy consumption was found to be 11.8%. It was proposed in the study that reusing the heat wasted during this pretreatment method can reduce energy consumption in full-scale operations. The energy expenditure during MW pretreatment varies with changes in solid concentration, and the energy varies between 37.5–150 k Wh/m3 for the solid concentration between 5.9–52 g/L. In order to achieve positive net energy, it was calculated that biogas production must be increased by 150% in a large-scale process (Cano et al., 2015). A study by Banu et al. (2019b) revealed that microwave-alone pretreatment shows two times greater energy for cell cleavage when compared to microwave-mediated zeolite pretreatment on WAS. Additional energy was required to cleave the cells in biomass during disperser pretreatment of macroalgal biomass, as in the study by Tamilarasan et al. (2018), when the biomass concentration increases with lower moisture content due to the disturbance in the rotor–stator arrangement. In full-scale application, ultrasonic pretreatment for sludge was made to be energetically feasible if the energy usage must be 6 kWh/m3 for the sludge concentration of 30 g/L (Ariunbaatar et al., 2014). In a study by Banu et al. (2020a), the US pretreatment alone demanded higher energy input of 25,200 kJ/kg TS for the solubilization of 15.9%. Thus it was confirmed that the scaling up of a single pretreatment method is difficult in terms of an economic point of view. In conclusion, disperser pretreatment is an energy demanding process in terms of electricity usage, whereas it is effective in terms of solid reduction and organic matter release.
The energy used up for the thermal pretreatment was calculated as per the work by Kannah et al. (2019):
where TEp is the thermal energy during pretreatment in kJ, m is the mass of substrate in kg, SH is the specific heat of the substrate in kJ/kg°C, Tap is the temperature after pretreatment in °C, and Tbp is the temperature after pretreatment °C.
The increase in energy was observed when the temperature exceeded 100°C due to evaporation, which consumed some amount of energy. A study by Passos and Ferrer (2014b)stated that the thermal effect on microalgal biomass at 75°C–95°C has an additional energy generation of 30%. It is energy efficient to use thermal pretreatment since the heat energy recovered after cooling down the pretreated substrate can be utilized for maintaining digestion temperature.
Apart from all these energy requirements, all the processes need energy for pumping and stirring during the pretreatment and digestion process. In chemical and biological pretreatment, the energy utilization is much lesser as compared to other pretreatment methods. The energy for mixing or stirring the substrate was expressed as
where MP is the power required for mixing in kW, P is the impeller power number, ρ is the density of biomass in kg/m3, n is the revolutions per second, and Di is the impeller diameter in m.
Compared to the single pretreatment, the combined pretreatment shows higher energy efficiency since the perfect combinations of pretreatment can reduce the energy to a larger level.
5.2 Output energy
The output energy is associated with the generation of bioenergy during fermentation and anaerobic digestion process and the heat recovery after the pretreatment process. The energy acquired after pretreatment in the form of hydrogen was assessed as
where biodegradability is expressed in terms of COD/COD, organic load in terms of gCOD/m3, volume of the reactor in m3, and hydrogen yield in mL/gCOD, while 3.5 is the conversion factor.
Likewise, for methane production, by considering methane yield, reactor volume and the equation were expressed as
where MY is the methane yield in L/gCOD, LHVm is the lower heating value of methane in kJ/m3 methane, and MCE is the methane conversion efficiency.
Moreover, the heat recovered after the pretreatment process was given as per the study by Banu et al. (2018a):
where Tp is the temperature incurred for pretreatment in °C, Td is the temperature for digestion in °C.
5.3 Net energy balance
Net energy balance (NE) is the difference between recovered energy (RE) and spent energy (SE). Likewise, the ratio of recovered to spent energy is termed as energy ratio (ER). Based on this, the balance in energy during pretreatment methods was evaluated. The net energy and energy ratio were expressed as
The positive NE and ER greater than one show a gain in energy, whereas the negative NE and ER less than one show a loss of energy. The decrease in overall energy exhaustion is aided by the biomass loading in the reactor. The solid loading enhances the product yield; however, the operating expenditure reduces. The suitable pretreatment conditions were obligatory due to the agitation effect connected with the higher loading of substrates. It was reported in the study by Passos et al. (2014a) that an ER greater than .7 is impossible for single pretreatment methods. Şahinkaya and Sevimli (2013) demonstrated that the combination of sonication and thermal pretreatment of WAS shows that sonication is not feasible due to its higher energy usage and thus leads to economic decline.
In a phase-separated pretreatment of rice straw, Kavitha et al. (2020b) adopted the homogenization process for delignification followed by the bacterial pretreatment for disintegration and found that the net energy for phase-separated biomass was 769.08 kWh/ton, which assured the saving in energy. Likewise, in another study by Preethi J. R. et al. (2021), a positive NE of .01 kWh/ton was obtained while pretreating 1 tonne of WAS with alkali-activated persulfate invoked bacterial pretreatment (APS-BP) as compared to bacterial pretreatment alone (BP), which gains the NE of −0.049 kWh/ton. Higher production of biomethane in APS-BP compensates for the energy utilized during the deflocculation and disintegration process.
In combined pretreatment by Kannah et al. (2017b), using disperser invoked ozone pretreatment of WAS yielded lesser net energy due to the EPS fragmentation, which aided in the solubilization enhancement for efficient biomethane generation. Likewise, the study by Shanthi et al. (2019) showed that an energy ratio of .8 was achieved for 70% of delignification, followed by 22% solubilization during fruit and vegetable residues treatment by combining Dimethyl sulphoxide and ultrasonic pretreatment. The lower concentration of lignin does not cause any inhibition of anaerobic digestion. Sethupathy et al. (2020) achieved positive net energy of 900.64 kWh while achieving 27% solubilization from paper mill waste biosolids through the disperser-induced rhamnolipid disintegration process.
6 Cost analysis
Cost analysis is the crucial factor that assesses the economic viability of the pretreatment method. Except for WAS, all other cost analyses are still not implemented at full scale. The cost mainly comprises capital disbursement, anaerobic digester, and maintenance costs. The energy expended cost is one of the factors which is necessary to determine the beneficialness of the pretreatment process, and it almost accounts for about 62% of the capital cost (Sharmila et al., 2020a). In the wastewater treatment plant, biosolids management accounts for 50% of the operating cost, and the economic feasibility of the pretreatment method is marginally associated with bioenergy generation and solids reduction (Uthirakrishnan et al., 2022). The addition of the pretreatment process to the traditional anaerobic digester adds on operational expenditures, whereas in microalgal biomass, the effective bioenergy generation hinges on the cost incurred for cultivation, harvesting, conversion to energy operation of the digester and product purification, product price, and overhead charges. (Chia et al., 2018). The optimal pretreatment method selection is not only dependent on biodegradability and energy conversion efficiency but also on economical and energetic profits. In order to achieve economic feasibility, it is essential to maintain profits in operation, maintenance, and energy consumption costs (Gonzalez et al., 2018; Shrestha et al., 2020). Thus, a proper pretreatment pattern is necessary to assess the economic viability.
6.1 Operating cost
Operational cost (OPC) comprises labor cost, energy cost, chemicals, maintenance, and indirect cost. Indirect cost includes overhead charges during manufacturing and includes repair and maintenance, electricity for production, and equipment. The improvement in methane or biogas production through pretreatment technologies leads to higher OPC and maintenance costs (Junior et al., 2020). The generation of energy and process integration were closely interrelated to OPC.
Generally, pretreatment such as ultrasonication, high-temperature thermal, homogenization, and ozonation have higher OPC. Apart from this, the higher energy requirement in physical pretreatment is another barrier and thus has a higher capital cost (Oladejo et al., 2018). The mechanical pretreatment, namely, ultrasonication, has higher OPC with a higher cost for energy yield.
While using homogenization, the major features are easier operation and higher energy efficiency, thus leading to lesser investment and OPC. In chemical pretreatment, ozone consumes higher energy, and a higher range of equipment is needed; thus, it increases the operational cost. Moreover, the alkali pretreatment favors lower OPC (Ismail et al., 2017). Moreover, lime usage reduces the operational cost for an easier recovery, making this pretreatment method feasible (Kumar and Sharma 2017). In the biological pretreatment method, the energy cost is lower, but due to the usage of enzymes, the OPC increases (Atelge et al., 2020; Shrestha et al., 2020). Rittmann et al. (2008) assessed the OPC of full-scale anaerobic digestion of 3,300 m3 for the treatment of 380 m3 sludge on a daily basis by adopting pulse field pretreatment and accomplished net profit in this system. The cost obligatory for 1 kWh of energy was .23 dollars, and for solid reduction, the cost expended per kg of solids was .28 dollars (Kavitha et al., 2015). In third-generation biofuels, the substrate microalgae need a higher operational cost for harvesting biomass and thus are considered to be a major drawback (Ullah et al., 2014). For a closed cultivation system, the operational cost for harvesting was in the range of .1–.6 €/kg for the energy expenditure between .1–.7 kWh/kg of biomass (Muhammad et al., 2021). Based on OPC, the cost for chemicals and enzymes was higher, and thus the biological and chemical OPC is higher, and it is necessary to assess its performance during commercialization.
6.2 Capital cost
Capital cost (CAC) is a one-time investment, whereas the OPC reoccurs every year (Bhatia et al., 2021). It includes the cost incurred for the equipment, engineering, construction, and contingency. Quantitative economic analysis for different biomass pretreatment was performed by Eggeman and Elander (2005). They demonstrated that the CAC for dilute acid, ammonia fiber explosion, and hot water pretreatment was found to be 25, 25.7, and 4.5 million dollars. After achieving the product yield, they concluded that the acid-based pretreatment shows higher efficiency in economic aspects. The combination of single pretreatment enhances the limitation of individual methods and is linked with enhanced energy expenditure and higher capital and operation cost (Atelge et al., 2020). Physical pretreatment, such as microwave heating, has a higher capital revenue ratio regardless of higher investment (Vani et al., 2012). The efficient pretreatment and hydrolysis of cellulosic biomass account for about 20% of CAC and OPC in the biorefining industry (Klein-Marcuschamer et al., 2011), whereas Bhutto et al. (2017) stated that the CAC and OPC of pretreatment method account 40% of total processing cost. The two-step acid pretreatment was proposed by Humbird et al. (2011), who assessed that the CAC for corn stover pretreatment was around 13% of the total CAC. Likewise, in cellulosic crops, the presence of inhibitory products and their processing enhances the fixed CAC and production cost of feedstock, ranging from .02–1.10 dollars per kg of feedstock (Cheng et al., 2019). Integrated biorefinery is one of the cost-effective practices. It helps in the reduction of CAC and energy usage and provides efficient clarification for energy retrieval and waste discarding (Morero et al., 2020; Mabalane et al., 2021). The CAC related to the size of the plant in full scale and was expressed as
The approximate cost linked with the pretreatment process were70–150 US dollars per ton sludge of capital, operational, and maintenance costs (Pilli et al., 2020). In alkali pretreatment, the pH alteration is obligatory to restrict the methanogenic microorganism inhibition, and thus the additional cost is predictable. The acid pretreatment leads to equipment corrosion, and there is a need for a reactor that can be able to withstand acidic conditions and thus increase CAC (Gonzalez et al., 2018). The lower revenue and higher CAC are the major hurdles, and it is not economically viable in the present market.
6.3 Net profit
The profitability is closely interrelated with the selling price of biofuels produced and the cost incurred for feedstock. The decrease in feedstock and increase in selling price directly have a greater increase in profitability (Preethi et al., 2021). In the case of bioenergy production, the solid reduction cost and the energy cost were considered as the gain, whereas the consumables and the solid remaining disposal cost were considered to be the loss. In the biorefinery of microalgal biomass, the increase in profit of two times higher than the overall process cost was noted as compared to the profit due to energy generation alone. Moreover, in the case of MSW processing, a profit of 42 € per ton was achieved in managing waste by instigating a circular economy (Fan et al., 2020). Chemicals such as acid, alkali, and surfactant solubilize the biomass effectively, whereas it shows a loss in profit. Tamilarasan et al. (2018) reported that the combination of physical, mechanical, and chemical treatment for macroalgae enhances the net profit of 90 $ per ton of biomass for the enhanced methane production by 551.5%. Pretreatment increases the solubilization whereas it adds on the operational cost, and thus, there is a need for the assessment of bioenergy production. The net profit of the pretreatment process was given by Hay et al. (2015):
where sCODc is the reduction cost, ESC is the sale of energy, and PC is the pretreatment cost expressed in $. The ultrasonic pretreatment used up .0026 $ for the hydrogen generation of .077 mLH2/mLh and achieved .2132 $ as net profit (Hay et al., 2015). Likewise, the phase-separated ultrasonic mediated microwave pretreatment demonstrated by Kavitha et al. (2018) shows a net profit of 2.67 US$/ton for the ER of 1.08, and furthermore, it indicates an energy saving of 50%. The homogenization-mediated bacterial pretreatment on rice straw shows the biomethane production of 165 mL/gVS, with a net profit of 134.89 US$/ton and a cost-benefit ratio of 1.52 as in the study by Kavitha et al. (2020b). The higher cost of chemical retrieval can be counterbalanced with low-cost reactors for the pretreatment process and thus helps in the profitability of the entire process. Moreover, the process integration is also profitable, which drastically reduces energy utilization. Thus, for full-scale implementation, a quantitative cost analysis is required for pretreatment and energy production.
7 Existing research gap in the pretreatment sector
In the pretreatment method, microalgae pretreatment involves a limited time for the achievement of the desired result and diminishes the energy input (Onumaegbu et al., 2018). The combination of two or more pretreatment method counterpart the limitations of a single pretreatment method, and thus much consideration is necessary to develop the pretreatment combination by trial-and-error methods. Due to the ever-lasting research gaps among researchers, scientists, and engineers, the commercialization of the pretreatment process is unattainable for bioenergy production (Sharmila et al., 2020b). It is necessary to acquire the application field outlook concerning economic output that is instantly practicable in industries. There is a lack of novel results and application of the pretreatment process, and thus, there is also a need for a detailed assessment of biomass structure and pretreatment efficiency (Rezania et al., 2020). In order to assess the relationship between the research gap and commercialization, it is necessary to understand the effect of technical, economic, and ecological obstacles. It is necessary to identify the steps in bioenergy generation at which the economic effect is higher and thus provides an idea to assess research priorities for development (Russo and Ladisch, 2008). A wide range of microorganisms is used for organic substance degradation in order to maintain the stability, thus leading to increased production cost (Weber et al., 2010). Thus, research is needed to focus on biocatalyst development with efficient characteristics and lower production cost for better performance.
The combined pretreatment is unfavorable from an economic point of view, which affects the spore-producing microbes which are responsible for hydrogen production, andthus, the development of lesser influence treatment was necessary for microbial growth (Prabakar et al., 2018). In sludge pretreatment, the management and handling of pretreated sludge is also a major barrier due to the presence of solids (Sridhar et al., 2021). In order to avert the hydrolysis process, the pretreatment techniques were allowed, whereas the drastic energy usage and incomplete degradation led to the amalgamation of the deflocculation process. The sustainable deflocculation process is necessary in order to resist this issue.
The major obstacle in chemical pretreatment is liquid waste management and disposal due to the presence of a higher concentration of toxic materials, which needs additional costs for the extraction of toxic matters (Bensah and Mensah, 2013). In physical pretreatment, the major barrier is its higher energy and time requirement whereas in biological pretreatment, the augmentation in methane production during anaerobic digestion is still indefinite due to the biomass complex structure, thus needs very longer time and more space for pretreatment (Paudel et al., 2017). Further investigations are needed in the pretreatment for achieving higher efficiency by incorporating genetic engineering methods. While utilizing low-intensity SMF, the positive effect on methane generation was observed, whereas high-intensity SMF has not been investigated widely. In a study by Di Costanzo et al. (2022), high-intensity SMF showed a negative effect on methane generation, whereas the chemical precipitation of valuable compounds was promoted. A major limitation in mechanical pretreatment is its incompetence for lignin exclusion, which acts as a hurdle for carbohydrate availability for bioenergy production. Mechanical pretreatment reduces about 1–2 mm of LCB for the eradication of inhibitors during hydrolysis, as was found in the study by Neumann et al. (2016). The complete process consumed about 33% of total electricity and made it unsustainable. Consequently, energy reduction enhances economic improvement in the complete process. Apart from the generation of toxic substances and disposal of waste, the pretreatment method needs supplementary equipment and higher energy necessities. Additionally, it should be considered in cost and overall process viability (Den et al., 2018). It is compulsory to manage the waste generation after pretreatment by proper policies and government agencies by creating newer technologies for eradicating pollutants. The major economic conflict in the pretreatment method is due to knowledge deficiency, and there is no implementation of a new pretreatment method on a large scale yet (Rezania et al., 2020). Eventually, there is insufficient research on the application of different biomass on a large scale. Thus, there is a need for proper exploration before execution of the process from laboratory to commercial scale. Moreover, the pretreatment process is in the development stage, and effective optimization of the process is necessary prior to the energy generation.
8 Future recommendation
Amidst different pretreatment methods for enhanced bioenergy production, the biological method is the most optimistic method. Each pretreatment method has its own shortcomings based on the biomass type, the process adopted, and the end product. However, these studies have been performed on a small scale and have significant differences in lab scale findings and pilot scale output and causing disparity in industrial scale results. Chemical pretreatment has a better ability for solids to biogas production without any scum formation. The disadvantage is that the addition of chemicals affects AD efficiency. The development of a research area based on an immobilized system could be better in the future for the hydrolysis of biomass. The disperser or sonication pretreatment method achieves more attention for the pretreatment of solids, and linking the mechanical with other pretreatment methods has a synergic effect. Moreover, the studies on a laboratory scale are insufficient to estimate the cost at a larger scale. Thus, the scaling effect is of chief significance for obtaining a pragmatic assessment of energy and cost. Future studies must focus on the mechanism and reaction kinetics of inorganic matter retrieved from biomass and its impact on biomass molecular arrangements. Several studies have been made to cover up the research gaps for efficient bioenergy generation, whereas further research is needed to evaluate the synergistic effect of combined pretreatment with respect to cost. Apart from the economic and energy aspects, the environmental impact assessment during pretreatment is also needed to be studied based on the laboratory and full scale. Progression has been made in the field of biorefineries in order to eradicate the gaps between the production and commercialization of a product. Thus, collectively, there is a need to address all of these issues, and a viable pretreatment approach is necessary for large-scale commercialization.
9 Policies and regulation
Apart from the advancement in technologies, policies also play a major role in the commercialization of bioenergy produced from waste feedstock by providing subsidies to producers. Renewable energy directive commands that renewable energy contributes to 14% of the total energy usage in the transport segment by 2030. The Renewable Energy Sources Act was enforced in Germany, with the major aim being the development of AD. This policy was further divided into renewable energy feed-in regulation and the Recycling and Waste Management Act, which came into force in the years 1991 and 1996, respectively. Renewable energy feed-in regulation incorporates grid connection for renewable energy plants, feed-in preferences, and 20 years tariff, whereas Recycling and Waste Management Act gives importance to waste reuse by converting the organic-rich waste to energy or valued products. The Energy Independence and Security Act (EISA), signed in the United States in 2007, promotes clean renewable fuel production. An annual increment of 36 billion gallons of renewable fuel by 2022 was set as the target. The indirect emission must be accounted for since the present policies promoted the shift towards the practice of second-generation biofuels over first-generation biofuels. For instance, the application of fertilizer has to be considered while removing crop residues, thus altering the chemical properties of soil. Thus, the excess cost must be accounted for fertilizers used. These policies can have a wide effect on biomass and energy generation, thus promoting the commercialization of bioenergy produced.
10 Conclusion
This study reviews the wide-ranging aspects of the enhancement of energy production aids with the use of pretreatment methods, whereas the usage of energy and cost makes it less desirable. The bioenergy production from waste biomass was efficiently obtained by various combinations of pretreatment and possesses greater potential to meet future energy security. The inhibitory components in biomass after pretreatment must be identified in order to reduce the pretreatment expense and reactor configuration. Grounded on these, the pretreatment methods, the solid reduction, energy generation, and disposal of leftovers must be carefully investigated for scaling up the process since it needs in-depth investigations. Furthermore, novel pretreatment methods are needed to be explored for improving waste biomass conversion. A single pretreatment method is not possible for all types of biomass since the biomass property, environment, and source vary. Thus, it is necessary to investigate computational technologies for the optimization of the pretreatment method since it is an effective tool for the analysis of chemical processes automatically and recognize the optimum methodologies. Moreover, it is efficient in constructing process modelling and simulation for optimizing economic efficiency.
For upscaling the pretreatment method to the industrial level, the sustainability, energy consumption, capital cost, and environmental effects are still a greater challenge. Consequently, the forthcoming work should evaluate the large-scale amalgamation and integrated strategy for waste management. Broadly, it is concluded that based on economic, environmental, and energy aspects, pretreatment selection is problematic. In order to utilize the resource for full potential for bioenergy generation, the policymaker must consider a standardized process, which paves the way for energy generation. Furthermore, the government should support and consider the unused resource for bioenergy generation, which thereby decreases GHG emissions and manages the waste in an effective manner.
Author contributions
PM, GM, and RB contributed to the conception and design. PM wrote the original draft of the manuscript. GM and RB periodically supervised the written part. All authors contributed to manuscript revision and read and approved the submitted version.
Funding
This work was supported by the Department of Biotechnology, India, under its initiative Mission Innovation Challenge Scheme (IC4). The grant from the project entitled “A novel integrated biorefinery for conversion of lignocellulosic agro waste into value-added products and bioenergy (BT/PR31054/PBD/26/763/2019)” was utilized for this study.
Conflict of interest
The authors declare that the research was conducted in the absence of any commercial or financial relationships that could be construed as a potential conflict of interest.
Publisher’s note
All claims expressed in this article are solely those of the authors and do not necessarily represent those of their affiliated organizations, or those of the publisher, the editors, and the reviewers. Any product that may be evaluated in this article, or claim that may be made by its manufacturer, is not guaranteed or endorsed by the publisher.
References
Adams, P., Bridgwater, T., Lea-Langton, A., Ross, A., and Watson, I. (2018). “Biomass conversion technologies,” in Greenhouse gas balances of bioenergy systems (United states: Academic Press), 107–139.
Aguilar-Reynosa, A., Romaní, A., Rodríguez-Jasso, R. M., Aguilar, C. N., Garrote, G., and Ruiz, H. A. (2017). Microwave heating processing as alternative of pretreatment in second-generation biorefinery: An overview. Energy Convers. Manag. 136, 50–65. doi:10.1016/j.enconman.2017.01.004
Ahmed, S. F., Liu, G., Mofijur, M., Azad, A. K., Hazrat, M. A., and Chu, Y. M. (2021). Physical and hybrid modelling techniques for earth-air heat exchangers in reducing building energy consumption: Performance, applications, progress, and challenges. Sol. Energy 216, 274–294. doi:10.1016/j.solener.2021.01.022
Alper, K., Tekin, K., Karagöz, S., and Ragauskas, A. J. (2020). Sustainable energy and fuels from biomass: A review focusing on hydrothermal biomass processing. Sustain. Energy and Fuels 4 (9), 4390–4414. doi:10.1039/d0se00784f
Ambrose, H. W., Chin, C. T. L., Hong, E., Philip, L., Suraishkumar, G. K., Sen, T. K., et al. (2020). Effect of hybrid (microwave-H2O2) feed sludge pretreatment on single and two-stage anaerobic digestion efficiency of real mixed sewage sludge. Process Saf. Environ. Prot. 136, 194–202. doi:10.1016/j.psep.2020.01.032
Appels, L., Dewil, R., Baeyens, J., and Degrève, J. (2008). Ultrasonically enhanced anaerobic digestion of waste activated sludge. Int. J. Sustain. Eng. 1 (2), 94–104. doi:10.1080/19397030802243319
Ariunbaatar, J., Panico, A., Esposito, G., Pirozzi, F., and Lens, P. N. (2014). Pretreatment methods to enhance anaerobic digestion of organic solid waste. Appl. energy 123, 143–156. doi:10.1016/j.apenergy.2014.02.035
Atay, Ş., and Akbal, F. (2016). Classification and effects of sludge disintegration technologies integrated into sludge handling units: An overview. CLEAN–Soil, Air, Water 44 (9), 1198–1213. doi:10.1002/clen.201400084
Atelge, M. R., Atabani, A. E., Banu, J. R., Krisa, D., Kaya, M., Eskicioglu, C., et al. (2020). A critical review of pretreatment technologies to enhance anaerobic digestion and energy recovery. Fuel 270, 117494. doi:10.1016/j.fuel.2020.117494
Babu, R., Capannelli, G., and Comite, A. (2021). Effect of different pretreatments on sludge solubilization and estimation of bioenergy potential. Processes 9 (8), 1382. doi:10.3390/pr9081382
Banu, J. R., and Kavitha, S. (2017). “Various sludge pretreatments: Their impact on biogas generation BT - waste biomass management – a holistic approach,” in Lakhveer Singh and vipin chandra kalia. (Cham: Springer International Publishing), 39–71.
Banu, J. R., Eswari, A. P., Kavitha, S., Kannah, R. Y., Kumar, G., Jamal, M. T., et al. (2019b). Energetically efficient microwave disintegration of waste activated sludge for biofuel production by zeolite: Quantification of energy and biodegradability modelling. Int. J. Hydrogen Energy 44 (4), 2274–2288. doi:10.1016/j.ijhydene.2018.06.040
Banu, J. R., Kannah, R. Y., Kavitha, S., Ashikvivek, A., Bhosale, R. R., and Kumar, G. (2020a). Cost effective biomethanation via surfactant coupled ultrasonic liquefaction of mixed microalgal biomass harvested from open raceway pond. Bioresour. Technol. 304, 123021. doi:10.1016/j.biortech.2020.123021
Banu, J. R., Kannah, R. Y., Kavitha, S., Gunasekaran, M., and Kumar, G. (2018b). Novel insights into scalability of biosurfactant combined microwave disintegration of sludge at alkali pH for achieving profitable bioenergy recovery and net profit. Bioresour. Technol. 267, 281–290. doi:10.1016/j.biortech.2018.07.046
Banu, J. R., Sharmila, V. G., Kannah, R. Y., Kanimozhi, R., Elfasakhany, A., Gunasekaran, M., et al. (2022a). Impact of novel deflocculant ZnO/Chitosan nanocomposite film in disperser pretreatment enhancing energy efficient anaerobic digestion: Parameter assessment and cost exploration. Chemosphere 286, 131835. doi:10.1016/j.chemosphere.2021.131835
Banu, J. R., Sharmila, V. G., Kavitha, S., Rajajothi, R., Gunasekaran, M., Angappane, S., et al. (2020b). TiO2-chitosan thin film induced solar photocatalytic deflocculation of sludge for profitable bacterial pretreatment and biofuel production. Fuel 273, 117741. doi:10.1016/j.fuel.2020.117741
Banu, J. R., Sharmila, V. G., Kumar, S. A., Kumar, G., Nguyen, D. D., Saratale, G. D., et al. (2019a). Cost effective sludge reduction using synergetic effect of dark Fenton and disperser treatment. J. Clean. Prod. 207, 261–270. doi:10.1016/j.jclepro.2018.09.252
Banu, J. R., Sugitha, S., Kannah, R. Y., Kavitha, S., and Yeom, I. T. (2018a). Marsilea spp.—a novel source of lignocellulosic biomass: Effect of solubilized lignin on anaerobic biodegradability and cost of energy products. Bioresour. Technol. 255, 220–228. doi:10.1016/j.biortech.2018.01.103
Banu, J. R., Sugitha, S., Kavitha, S., Kannah, Y., Kim, S. H., Zhen, G., et al. (2022b). Profitable disperser coupled surfactant pretreatment of aquatic phytomass for energy efficient solubilization and biomethanation: A study on lignin inhibition and its possible solutions. Sustain. Energy Fuels 6, 3195–3207. doi:10.1039/D2SE00208F
Behera, S., Arora, R., Nandhagopal, N., and Kumar, S. (2014). Importance of chemical pretreatment for bioconversion of lignocellulosic biomass. Renew. Sustain. Energy Rev. 36, 91–106. https://www.sciencedirect.com/science/article/pii/S1364032114002743. doi:10.1016/j.rser.2014.04.047
Bensah, E. C., and Mensah, M. (2013). Chemical pretreatment methods for the production of cellulosic ethanol: Technologies and innovations. Int. J. Chem. Eng. 2013, 1–21. doi:10.1155/2013/719607
Bernaerts, T. M., Gheysen, L., Foubert, I., Hendrickx, M. E., and Van Loey, A. M. (2019). The potential of microalgae and their biopolymers as structuring ingredients in food: A review. Biotechnol. Adv. 37 (8), 107419. doi:10.1016/j.biotechadv.2019.107419
Bhatia, S. K., Jagtap, S. S., Bedekar, A. A., Bhatia, R. K., Patel, A. K., Pant, D., et al. (2020). Recent developments in pretreatment technologies on lignocellulosic biomass: Effect of key parameters, technological improvements, and challenges. Bioresour. Technol. 300, 122724. doi:10.1016/j.biortech.2019.122724
Bhatia, S. K., Jagtap, S. S., Bedekar, A. A., Bhatia, R. K., Rajendran, K., Pugazhendhi, A., et al. (2021). Renewable biohydrogen production from lignocellulosic biomass using fermentation and integration of systems with other energy generation technologies. Sci. Total Environ. 765, 144429. doi:10.1016/j.scitotenv.2020.144429
Bhutto, A. W., Qureshi, K., Harijan, K., Abro, R., Abbas, T., Bazmi, A. A., et al. (2017). Insight into progress in pre-treatment of lignocellulosic biomass. Energy 122, 724–745. doi:10.1016/j.energy.2017.01.005
Bijarchiyan, M., Sahebi, H., and Mirzamohammadi, S. (2020). A sustainable biomass network design model for bioenergy production by anaerobic digestion technology: Using agricultural residues and livestock manure. Energy, Sustain. Soc. 10 (1), 19. doi:10.1186/s13705-020-00252-7
Bonilla, S., Choolaei, Z., Meyer, T., Edwards, E. A., Yakunin, A. F., and Allen, D. G. (2018). Evaluating the effect of enzymatic pretreatment on the anaerobic digestibility of pulp and paper biosludge. Biotechnol. Rep. 17, 77–85. doi:10.1016/j.btre.2017.12.009
Cano, R., Pérez-Elvira, S. I., and Fdz-Polanco, F. (2015). Energy feasibility study of sludge pretreatments: A review. Appl. Energy 149, 176–185. doi:10.1016/j.apenergy.2015.03.132
Cardeña, R., Moreno, G., Bakonyi, P., and Buitrón, G. (2017). Enhancement of methane production from various microalgae cultures via novel ozonation pretreatment. Chem. Eng. J. 307, 948–954. doi:10.1016/j.cej.2016.09.016
Cesaro, A., and Belgiorno, V. (2014). Pretreatment methods to improve anaerobic biodegradability of organic municipal solid waste fractions. Chem. Eng. J. 240, 24–37. doi:10.1016/j.cej.2013.11.055
Chaturvedi, V., and Verma, P. (2013). An overview of key pretreatment processes employed for bioconversion of lignocellulosic biomass into biofuels and value added products. 3 Biotech. 3 (5), 415–431. doi:10.1007/s13205-013-0167-8
Chen, D., Yin, L., Wang, H., and He, P. (2015). Reprint of: Pyrolysis technologies for municipal solid waste: A review. Waste Manag. 37, 116–136. doi:10.1016/j.wasman.2015.01.022
Chen, W. H., Lo, H. J., Yu, K. L., Ong, H. C., and Sheen, H. K. (2021). Valorization of sorghum distillery residue to produce bioethanol for pollution mitigation and circular economy. Environ. Pollut. 285, 117196. doi:10.1016/j.envpol.2021.117196
Cheng, M. H., Huang, H., Bruce, D., and Singh, V. (2019). The costs of sugar production from different feedstocks and processing technologies: Review of industrial sugar production costs. Biofuels, Bioprod. Biorefining 13, 723–739. doi:10.1002/bbb.1976
Chhabra, V., Parashar, A., Shastri, Y., and Bhattacharya, S. (2021). Techno-economic and life cycle assessment of pyrolysis of unsegregated urban municipal solid waste in India. Industrial Eng. Chem. Res. 60 (3), 1473–1482. doi:10.1021/acs.iecr.0c04746
Chia, S. R., Chew, K. W., Show, P. L., Yap, Y. J., Ong, H. C., Ling, T. C., et al. (2018). Analysis of economic and environmental aspects of microalgae biorefinery for biofuels production: A review. Biotechnol. J. 13 (6), 1700618. doi:10.1002/biot.201700618
Costa, J. A. V., Freitas, B. C. B., Moraes, L., Zaparoli, M., and Morais, M. G. (2020). Progress in the physicochemical treatment of microalgae biomass for value-added product recovery. Bioresour. Technol. 301, 122727. doi:10.1016/j.biortech.2019.122727
Cui, D., Tan, C., Deng, H., Gu, X., Pi, S., Chen, T., et al. (2020). Biosorption mechanism of aqueous Pb2+, Cd2+, and Ni2+ ions on extracellular polymeric substances (EPS). Archaea 2020, 8891543. doi:10.1155/2020/8891543
da Silva Machado, A., and Ferraz, A. (2017). Biological pretreatment of sugarcane bagasse with basidiomycetes producing varied patterns of biodegradation. Bioresour. Technol. 225, 17–22. doi:10.1016/j.biortech.2016.11.053
Darji, D., Alias, Y., Som, F., and Razak, N. (2014). Microwave heating and hydrolysis of rubber wood biomass in ionic liquids. J. Chem. Technol. Biotechnol. 90, 2050–2056. doi:10.1002/jctb.4516
Dębowski, M., and Zieliński, M. (2020). Technological effectiveness of sugar-industry effluent methane fermentation in a fluidized active filling reactor (FAF-R). Energies 13 (24), 6626. doi:10.3390/en13246626
Den, W., Sharma, V. K., Lee, M., Nadadur, G., and Varma, R. S. (2018). Lignocellulosic biomass transformations via greener oxidative pretreatment processes: Access to energy and value-added chemicals. Front. Chem. 6, 141. doi:10.3389/fchem.2018.00141
Di Costanzo, N., Di Capua, F., Cesaro, A., Mascolo, M. C., Pirozzi, F., and Esposito, G. (2022). Impact of high-intensity static magnetic field on chemical properties and anaerobic digestion of sewage sludge. Waste Biomass Valorization, 1–11. doi:10.1007/s12649-022-01891-x
Dollhofer, V., Dandikas, V., Dorn-In, S., Bauer, C., Lebuhn, M., and Bauer, J. (2018). Accelerated biogas production from lignocellulosic biomass after pre-treatment with Neocallimastix frontalis. Bioresour. Technol. 264, 219–227. doi:10.1016/j.biortech.2018.05.068
dos Santos, I. F. S., Mensah, J. H. R., Gonçalves, A. T. T., and Barros, R. M. (2020). Incineration of municipal solid waste in Brazil: An analysis of the economically viable energy potential. Renew. Energy 149, 1386–1394. doi:10.1016/j.renene.2019.10.134
Ebenezer, A. V., Kaliappan, S., Kumar, S. A., Yeom, I. T., and Banu, J. R. (2015). Influence of deflocculation on microwave disintegration and anaerobic biodegradability of waste activated sludge. Bioresour. Technol. 185, 194–201. doi:10.1016/j.biortech.2015.02.102
Eggeman, T., and Elander, R. T. (2005). Process and economic analysis of pretreatment technologies. Bioresour. Technol. 96 (18), 2019–2025. doi:10.1016/j.biortech.2005.01.017
Elalami, D., Carrere, H., Monlau, F., Abdelouahdi, K., Oukarroum, A., and Barakat, A. (2019). Pretreatment and co-digestion of wastewater sludge for biogas production: Recent research advances and trends. Renew. Sustain. Energy Rev. 114, 109287. doi:10.1016/j.rser.2019.109287
Eswari, A. P., Kavitha, S., Banu, J. R., Karthikeyan, O. P., and Yeom, I. T. (2017). H2O2 induced cost effective microwave disintegration of dairy waste activated sludge in acidic environment for efficient biomethane generation. Bioresour. Technol. 244, 688–697. doi:10.1016/j.biortech.2017.07.078
Eswari, P., Kavitha, S., Kaliappan, S., Yeom, I. T., and Banu, J. R. (2016). Enhancement of sludge anaerobic biodegradability by combined microwave-H2O2 pretreatment in acidic conditions. Environ. Sci. Pollut. Res. 23 (13), 13467–13479. doi:10.1007/s11356-016-6543-2
Fan, Y. V., Klemeš, J. J., Gordon Walmsley, T., and Bertók, B. (2020). Implementing circular economy in municipal solid waste treatment system using P-graph. Sci. Total Environ. 701, 134652. doi:10.1016/j.scitotenv.2019.134652
Feki, E., Battimelli, A., Sayadi, S., Dhouib, A., and Khoufi, S. (2020). High-rate anaerobic digestion of waste activated sludge by integration of electro-Fenton process. Molecules 25 (3), 626. doi:10.3390/molecules25030626
Fu, Q., Wang, D., Li, X., Yang, Q., Xu, Q., Ni, B. J., et al. (2021). Towards hydrogen production from waste activated sludge: Principles, challenges and perspectives. Renew. Sustain. Energy Rev. 135, 110283. doi:10.1016/j.rser.2020.110283
Golembiewski, B., Sick, N., and Bröring, S. (2015). The emerging research landscape on bioeconomy: What has been done so far and what is essential from a technology and innovation management perspective? Innovative Food Sci. Emerg. Technol. 29, 308–317. doi:10.1016/j.ifset.2015.03.006
Gonzalez, A., Hendriks, A. T. W. M., van Lier, J. B., and de Kreuk, M. (2018). Pre-treatments to enhance the biodegradability of waste activated sludge: Elucidating the rate limiting step. Biotechnol. Adv. 36 (5), 1434–1469. doi:10.1016/j.biotechadv.2018.06.001
Gonzalez, R. R., Sivagurunathan, P., and Kim, S. H. (2016). Effect of severity on dilute acid pretreatment of lignocellulosic biomass and the following hydrogen fermentation. Int. J. Hydrogen Energy 41 (46), 21678–21684. doi:10.1016/j.ijhydene.2016.06.198
Gruber-Brunhumer, M. R., Jerney, J., Zohar, E., Nussbaumer, M., Hieger, C., Bochmann, G., et al. (2015). Acutodesmus obliquus as a benchmark strain for evaluating methane production from microalgae: Influence of different storage and pretreatment methods on biogas yield. Algal Res. 12, 230–238. doi:10.1016/j.algal.2015.08.022
Guo, G., Li, Y., Zhou, S., Chen, Y., Qin, Y., and Li, Y. Y. (2022). Enhanced degradation and biogas production of waste activated sludge by a high-solid anaerobic membrane bioreactor together with in pipe thermal pretreatment process. Bioresour. Technol. 346, 126583. doi:10.1016/j.biortech.2021.126583
Hay, J., Wen, X., Wu, T. Y., Juan, J. C., and Jamaliah, M. J. (2015). Improved biohydrogen production and treatment of pulp and paper mill effluent through ultrasonication pretreatment of wastewater. Energy Convers. Manag. 106, 576–583. doi:10.1016/j.enconman.2015.08.040
Hernández-Beltrán, J. U., Hernández-De Lira, I. O., Cruz-Santos, M. M., Saucedo-Luevanos, A., Hernández-Terán, F., and Balagurusamy, N. (2019). Insight into pretreatment methods of lignocellulosic biomass to increase biogas yield: Current state, challenges, and opportunities. Appl. Sci. 9 (18), 3721. doi:10.3390/app9183721
Humbird, D., Davis, R., Tao, L., Kinchin, C., Hsu, D., Aden, A., et al. (2011). Process design and economics for biochemical conversion of lignocellulosic biomass to ethanol: Dilute-acid pretreatment and enzymatic hydrolysis of corn stover (No. NREL/TP-5100-47764). Golden, CO (United States): National Renewable Energy Lab.
IEA (2021). IEA, IRENA, WMO, WBG, WHO,tracking SDG7: The energy progress report 2021. Washington, DC: The World Bank.
Ismail, S., Saharuddin, M. Q., and Mohamed Zahari, M. S. (2017). Upgraded seawater-alkaline pre-treatment of lignocellulosic biomass for bio-methane production. Energy Procedia 138, 372–379. doi:10.1016/j.egypro.2017.10.390
Juárez, J. M., Pastor, E. R., Sevilla, J. M. F., Torre, R. M., García-Encina, P. A., and Rodríguez, S. B. (2018). Effect of pretreatments on biogas production from microalgae biomass grown in pig manure treatment plants. Bioresour. Technol. 257, 30–38. doi:10.1016/j.biortech.2018.02.063
Junior, I. V., de Almeida, R., and Cammarota, M. C. (2020). A review of sludge pretreatment methods and Co-digestion to boost biogas production and energy self-sufficiency in wastewater treatment plants. J. water process Eng. 40, 101857. doi:10.1016/j.jwpe.2020.101857
Kannah, R. Y., Kavitha, S., Banu, J. R., Karthikeyan, O. P., and Sivashanmugham, P. (2017b). Dispersion induced ozone pretreatment of waste activated biosolids: Arriving biomethanation modelling parameters, energetic and cost assessment. Bioresour. Technol. 244, 679–687. doi:10.1016/j.biortech.2017.08.001
Kannah, R. Y., Kavitha, S., Banu, J. R., Yeom, I. T., and Johnson, M. J. B. T. (2017a). Synergetic effect of combined pretreatment for energy efficient biogas generation. Bioresour. Technol. 232, 235–246. doi:10.1016/j.biortech.2017.02.042
Kannah, R. Y., Kavitha, S., Sivashanmugham, P., Kumar, G., Nguyen, D. D., Chang, S. W., et al. (2019). Biohydrogen production from rice straw: Effect of combinative pretreatment, modelling assessment and energy balance consideration. Int. J. Hydrogen Energy 44 (4), 2203–2215. doi:10.1016/j.ijhydene.2018.07.201
Kannah, Y., Kavitha, S., Sivashanmugam, P., Kumar, G., and Banu J., R. (2021). Ultrasonic induced mechanoacoustic effect on delignification of rice straw for cost effective biopretreatment and biomethane recovery. Sustain. Energy and Fuels 5 (6), 1832–1844. doi:10.1039/d0se01814g
Karimi, K., and Taherzadeh, M. J. (2016). A critical review on analysis in pretreatment of lignocelluloses: Degree of polymerization, adsorption/desorption, and accessibility. Bioresour. Technol. 203, 348–356. doi:10.1016/j.biortech.2015.12.035
Kavitha, S., Banu, J. R., Kumar, G., Kaliappan, S., and Yeom, I. T. (2018). Profitable ultrasonic assisted microwave disintegration of sludge biomass: Modelling of biomethanation and energy parameter analysis. Bioresour. Technol. 254, 203–213. doi:10.1016/j.biortech.2018.01.072
Kavitha, S., Kannah, R. Y., Banu, J. R., Kaliappan, S., and Johnson, M. (2017c). Biological disintegration of microalgae for biomethane recovery-prediction of biodegradability and computation of energy balance. Bioresour. Technol. 244, 1367–1375. doi:10.1016/j.biortech.2017.05.007
Kavitha, S., Kannah, R. Y., Gunasekaran, M., Nguyen, D. D., Ala'a, H., Park, J. H., et al. (2019a). Effect of low intensity sonic mediated fragmentation of anaerobic granules on biosurfactant secreting bacterial pretreatment: Energy and mass balance analysis. Bioresour. Technol. 279, 156–165. doi:10.1016/j.biortech.2019.01.118
Kavitha, S., Kannah, R. Y., Kasthuri, S., Gunasekaran, M., Pugazhendi, A., Rene, E. R., et al. (2020b). Profitable biomethane production from delignified rice straw biomass: The effect of lignin, energy and economic analysis. Green Chem. 22 (22), 8024–8035. doi:10.1039/d0gc02738c
Kavitha, S., Kannah, Y., Gunasekaran, M., and Kumar, G. (2020a). Rhamnolipid induced deagglomeration of anaerobic granular biosolids for energetically feasible ultrasonic homogenization and profitable biohydrogen. Int. J. Hydrogen Energy 45 (10), 5890–5899. doi:10.1016/j.ijhydene.2019.04.063
Kavitha, S., Kumar, S. A., Yogalakshmi, K. N., Kaliappan, S., and Banu, J. R. (2013). Effect of enzyme secreting bacterial pretreatment on enhancement of aerobic digestion potential of waste activated sludge interceded through EDTA. Bioresour. Technol. 150, 210–219. doi:10.1016/j.biortech.2013.10.021
Kavitha, S., Preethi, J., Rajesh Banu, J., and Yeom, I. T. (2017a). Low temperature thermochemical mediated energy and economically efficient biological disintegration of sludge: Simulation and prediction studies for anaerobic biodegradation. Chem. Eng. J. 317, 481–492. doi:10.1016/j.cej.2017.02.092
Kavitha, S., Saranya, T., Kaliappan, S., Kumar, S. A., Yeom, I. T., and Banu, J. R. (2015). Accelerating the sludge disintegration potential of a novel bacterial strain Planococcus jake 01 by CaCl2 induced deflocculation. Bioresour. Technol. 175, 396–405. doi:10.1016/j.biortech.2014.10.122
Kavitha, S., Schikaran, M., Kannah, R. Y., Gunasekaran, M., Kumar, G., and Banu, J. R. (2019b). Nanoparticle induced biological disintegration: A new phase separated pretreatment strategy on microalgal biomass for profitable biomethane recovery. Bioresour. Technol. 289, 121624. doi:10.1016/j.biortech.2019.121624
Kavitha, S., Subbulakshmi, P., Banu, J. R., Gobi, M., and Yeom, I. T. (2017b). Enhancement of biogas production from microalgal biomass through cellulolytic bacterial pretreatment. Bioresour. Technol. 233, 34–43. doi:10.1016/j.biortech.2017.02.081
Kazimierowicz, J., Bartkowska, I., and Walery, M. (2021). Effect of low-temperature conditioning of excess dairy sewage sludge with the use of solidified carbon dioxide on the efficiency of methane fermentation. Energies 14 (1), 150. doi:10.3390/en14010150
Kim, J., Sunagawa, M., Kobayashi, S., Shin, T., and Takayama, C. (2016). Developmental localization of calcitonin gene-related peptide in dorsal sensory axons and ventral motor neurons of mouse cervical spinal cord. Neurosci. Res. 105, 42–48. doi:10.1016/j.neures.2015.09.003
Klein-Marcuschamer, D., Simmons, B. A., and Blanch, H. W. (2011). Techno-economic analysis of a lignocellulosic ethanol biorefinery with ionic liquid pre-treatment. Biofuels, Bioprod. Biorefining 5 (5), 562–569. doi:10.1002/bbb.303
Kostas, E. T., Beneroso, D., and Robinson, J. P. (2017). The application of microwave heating in bioenergy: A review on the microwave pre-treatment and upgrading technologies for biomass. Renew. Sustain. Energy Rev. 77, 12–27. doi:10.1016/j.rser.2017.03.135
Kumar, A. K., and Sharma, S. (2017). Recent updates on different methods of pretreatment of lignocellulosic feedstocks: A review. Bioresour. Bioprocess. 4 (1), 7–19. doi:10.1186/s40643-017-0137-9
Kumar, G., Nguyen, D. D., Sivagurunathan, P., Kobayashi, T., Xu, K., and Chang, S. W. (2018). Cultivation of microalgal biomass using swine manure for biohydrogen production: Impact of dilution ratio and pretreatment. Bioresour. Technol. 260, 16–22. doi:10.1016/j.biortech.2018.03.029
Kumar, M. D., Sharmila, V. G., Kumar, G., Park, J. H., Al-Qaradawi, S. Y., and Banu, J. R. (2022). Surfactant induced microwave disintegration for enhanced biohydrogen production from macroalgae biomass: Thermodynamics and energetics. Bioresour. Technol. 350, 126904. doi:10.1016/j.biortech.2022.126904
Kumar, M. (2019). Global environmental policies with innovation spillovers. Manag. Environ. Qual. Int. J. 30 (4), 833–850. doi:10.1108/meq-05-2018-0098
Kumari, D., and Singh, R. (2018). Pretreatment of lignocellulosic wastes for biofuel production: A critical review. Renew. Sustain. Energy Rev. 90, 877–891. doi:10.1016/j.rser.2018.03.111
Lee, S. Y., Sankaran, R., Chew, K. W., Tan, C. H., Krishnamoorthy, R., Chu, D. T., et al. (2019). Waste to bioenergy: A review on the recent conversion technologies. Bmc Energy 1 (1), 4–22. doi:10.1186/s42500-019-0004-7
Liang, T., Elmaadawy, K., Liu, B., Hu, J., Hou, H., and Yang, J. (2021). Anaerobic fermentation of waste activated sludge for volatile fatty acid production: Recent updates of pretreatment methods and the potential effect of humic and nutrients substances. Process Saf. Environ. Prot. 145, 321–339. doi:10.1016/j.psep.2020.08.010
Liu, J., Yang, M., Zhang, J., Zheng, J., Xu, H., Wang, Y., et al. (2018). A comprehensive insight into the effects of microwave-H2O2 pretreatment on concentrated sewage sludge anaerobic digestion based on semi-continuous operation. Bioresour. Technol. 256, 118–127. doi:10.1016/j.biortech.2018.01.126
Liu, X., He, D., Wu, Y., Xu, Q., Wang, D., Yang, Q., et al. (2020). Freezing in the presence of nitrite pretreatment enhances hydrogen production from dark fermentation of waste activated sludge. J. Clean. Prod. 248, 119305. doi:10.1016/j.jclepro.2019.119305
Lizama, A. C., Figueiras, C. C., Herrera, R. R., Pedreguera, A. Z., and Espinoza, J. E. R. (2017). Effects of ultrasonic pretreatment on the solubilization and kinetic study of biogas production from anaerobic digestion of waste activated sludge. Int. Biodeterior. Biodegrad. 123, 1–9. doi:10.1016/j.ibiod.2017.05.020
Mabalane, P. N., Oboirien, B. O., Sadiku, E. R., and Masukume, M. (2021). A techno-economic analysis of anaerobic digestion and gasification hybrid system: Energy recovery from municipal solid waste in south Africa. Waste Biomass Valorization 12 (3), 1167–1184. doi:10.1007/s12649-020-01043-z
Machnicka, A., Grübel, K., Wacławek, S., and Sikora, K. (2019). Waste-activated sludge disruption by dry ice: Bench scale study and evaluation of heat phase transformations. Environ. Sci. Pollut. Res. 26 (26), 26488–26499. doi:10.1007/s11356-019-05889-2
Mahmod, S. S., Jahim, J. M., and Abdul, P. M. (2017). Pretreatment conditions of palm oil mill effluent (POME) for thermophilic biohydrogen production by mixed culture. Int. J. hydrogen energy 42 (45), 27512–27522. doi:10.1016/j.ijhydene.2017.07.178
Matavos-Aramyan, S., and Moussavi, M. (2017). Advances in Fenton and Fenton based oxidation processes for industrial effluent contaminants control-a review. Int. J. Environ. Sci. Nat. Resour. 2 (4), 1–18. doi:10.19080/ijesnr.2017.02.555594
Mehedintu, A., Sterpu, M., and Soava, G. (2018). Estimation and forecasts for the share of renewable energy consumption in final energy consumption by 2020 in the European Union. Sustainability 10 (5), 1515. doi:10.3390/su10051515
Mehrez, I., Shobana, S., Semaan, G., Kim, S. H., and Kumar, G. (2022). Biomass based bioenergy: Technologies and impact on environmental sustainability. J. Korean Soc. Environ. Eng. 44 (1), 1–12. doi:10.4491/ksee.2022.44.1.1
Menon, A., Ren, F., Wang, J. Y., and Giannis, A. (2016). Effect of pretreatment techniques on food waste solubilization and biogas production during thermophilic batch anaerobic digestion. J. Material Cycles Waste Manag. 18 (2), 222–230. doi:10.1007/s10163-015-0395-6
Mirmasoumi, S., Ebrahimi, S., and Saray, R. K. (2018). Enhancement of biogas production from sewage sludge in a wastewater treatment plant: Evaluation of pretreatment techniques and co-digestion under mesophilic and thermophilic conditions. Energy 157, 707–717. doi:10.1016/j.energy.2018.06.003
Mohammed, Y. S., Mokhtar, A. S., Bashir, N., and Saidur, R. (2013). An overview of agricultural biomass for decentralized rural energy in Ghana. Renew. Sustain. Energy Rev. 20, 15–25. doi:10.1016/j.rser.2012.11.047
Morero, B., Montagna, A. F., Campanella, E. A., and Cafaro, D. C. (2020). Optimal process design for integrated municipal waste management with energy recovery in Argentina. Renew. Energy 146, 2626–2636. doi:10.1016/j.renene.2019.08.085
Mottet, A., Steyer, J. P., Déléris, S., Vedrenne, F., Chauzy, J., and Carrère, H. (2009). Kinetics of thermophilic batch anaerobic digestion of thermal hydrolysed waste activated sludge. Biochem. Eng. J. 46 (2), 169–175. doi:10.1016/j.bej.2009.05.003
Muhammad, G., Alam, M. A., Mofijur, M., Jahirul, M. I., Lv, Y., Xiong, W., et al. (2021). Modern developmental aspects in the field of economical harvesting and biodiesel production from microalgae biomass. Renew. Sustain. Energy Rev. 135, 110209. doi:10.1016/j.rser.2020.110209
Mupondwa, E., Li, X., Tabil, L., Sokhansanj, S., and Adapa, P. (2017). Status of Canada's lignocellulosic ethanol: Part I: Pretreatment technologies. Renew. Sustain. Energy Rev. 72, 178–190. doi:10.1016/j.rser.2017.01.039
Mustafa, A. M., Poulsen, T. G., Xia, Y., and Sheng, K. (2017). Combinations of fungal and milling pretreatments for enhancing rice straw biogas production during solid-state anaerobic digestion. Bioresour. Technol. 224, 174–182. doi:10.1016/j.biortech.2016.11.028
Neumann, P., Pesante, S., Venegas, M., and Vidal, G. (2016). Developments in pre-treatment methods to improve anaerobic digestion of sewage sludge. Rev. Environ. Sci. Bio/Technology 15 (2), 173–211. doi:10.1007/s11157-016-9396-8
Nguyen, X. P., Hoang, A. T., Ölçer, A. I., and Huynh, T. T. (2021). Record decline in global CO2 emissions prompted by COVID-19 pandemic and its implications on future climate change policies. Energy Sources, Part A 43, 1–4. doi:10.1080/15567036.2021.1879969
Nouha, K., Kumar, R. S., Balasubramanian, S., and Tyagi, R. D. (2018). Critical review of EPS production, synthesis and composition for sludge flocculation. J. Environ. Sci. 66, 225–245. doi:10.1016/j.jes.2017.05.020
O'Dwyer, J., Zhu, L., Granda, C. B., Chang, V. S., and Holtzapple, M. T. (2008). Neural network prediction of biomass digestibility based on structural features. Biotechnol. Prog. 24 (2), 283–292. doi:10.1021/bp070193v
Odnell, A., Recktenwald, M., Stensén, K., Jonsson, B. H., and Karlsson, M. (2016). Activity, life time and effect of hydrolytic enzymes for enhanced biogas production from sludge anaerobic digestion. Water Res. 103, 462–471. doi:10.1016/j.watres.2016.07.064
Oladejo, J., Shi, K., Luo, X., Yang, G., and Wu, T. (2018). A review of sludge-to-energy recovery methods. Energies 12 (1), 60. doi:10.3390/en12010060
Olatunji, K. O., Ahmed, N. A., and Ogunkunle, O. (2021). Optimization of biogas yield from lignocellulosic materials with different pretreatment methods: A review. Biotechnol. Biofuels 14 (1), 1–34. doi:10.1186/s13068-021-02012-x
Ong, H. C., Tiong, Y. W., Goh, B. H. H., Gan, Y. Y., Mofijur, M., Fattah, I. R., et al. (2021). Recent advances in biodiesel production from agricultural products and microalgae using ionic liquids: Opportunities and challenges. Energy Convers. Manag. 228, 113647. doi:10.1016/j.enconman.2020.113647
Onumaegbu, C., Mooney, J., Alaswad, A., and Olabi, A. G. (2018). Pre-treatment methods for production of biofuel from microalgae biomass. Renew. Sustain. Energy Rev. 93, 16–26. doi:10.1016/j.rser.2018.04.015
Papathoti, N. K., Laemchiab, K., Megavath, V. S., Keshav, P. K., Numparditsub, P., Le Thanh, T., et al. (2021). Augmented ethanol production from alkali-assisted hydrothermal pretreated cassava peel waste. Energy Sources, Part A 43, 1–11. doi:10.1080/15567036.2021.1928338
Park, W. J., and Ahn, J. H. (2011). Effects of microwave pretreatment on mesophilic anaerobic digestion for mixture of primary and secondary sludges compared with thermal pretreatment. Environ. Eng. Res. 16 (2), 103–109. doi:10.4491/eer.2011.16.2.103
Passos, F., and Ferrer, I. (2014b). Microalgae conversion to biogas: Thermal pretreatment contribution on net energy production. Environ. Sci. Technol. 48 (12), 7171–7178. doi:10.1021/es500982v
Passos, F., Hernandez-Marine, M., García, J., and Ferrer, I. (2014a). Long-term anaerobic digestion of microalgae grown in HRAP for wastewater treatment. Effect of microwave pretreatment. Water Res. 49, 351–359. doi:10.1016/j.watres.2013.10.013
Passos, F., Uggetti, E., Carrère, H., and Ferrer, I. (2014c). Pretreatment of microalgae to improve biogas production: A review. Bioresour. Technol. 172, 403–412. doi:10.1016/j.biortech.2014.08.114
Patil, R., Cimon, C., Eskicioglu, C., and Goud, V. (2021). Effect of ozonolysis and thermal pre-treatment on rice straw hydrolysis for the enhancement of biomethane production. Renew. Energy 179, 467–474. doi:10.1016/j.renene.2021.07.048
Paudel, S. R., Banjara, S. P., Choi, O. K., Park, K. Y., Kim, Y. M., and Lee, J. W. (2017). Pretreatment of agricultural biomass for anaerobic digestion: Current state and challenges. Bioresour. Technol. 245, 1194–1205. doi:10.1016/j.biortech.2017.08.182
Pecorini, I., Baldi, F., Carnevale, E. A., and Corti, A. (2016). Biochemical methane potential tests of different autoclaved and microwaved lignocellulosic organic fractions of municipal solid waste. Waste Manag. 56, 143–150. doi:10.1016/j.wasman.2016.07.006
Pilli, S., Bhunia, P., Yan, S., LeBlanc, R. J., Tyagi, R. D., and Surampalli, R. Y. (2011). Ultrasonic pretreatment of sludge: A review. Ultrason. sonochemistry 18 (1), 1–18. doi:10.1016/j.ultsonch.2010.02.014
Pilli, S., More, T. T., Yan, S., Tyagi, R. D., and Surampalli, R. Y. (2016). Fenton pre-treatment of secondary sludge to enhance anaerobic digestion: Energy balance and greenhouse gas emissions. Chem. Eng. J. 283, 285–292. doi:10.1016/j.cej.2015.07.056
Pilli, S., Pandey, A. K., Katiyar, A., Pandey, K., and Tyagi, R. D. (2020). “Pre-treatment technologies to enhance anaerobic digestion,” in Sustainable sewage sludge management and resource efficiency (London, UK: IntechOpen).
Ponnusamy, V. K., Nguyen, D. D., Dharmaraja, J., Shobana, S., Banu, J. R., Saratale, R. G., et al. (2019). A review on lignin structure, pretreatments, fermentation reactions and biorefinery potential. Bioresour. Technol. 271, 462–472. doi:10.1016/j.biortech.2018.09.070
Prabakar, D., Manimudi, V. T., Sampath, S., Mahapatra, D. M., Rajendran, K., Pugazhendhi, A., et al. (2018). Advanced biohydrogen production using pretreated industrial waste: Outlook and prospects. Renew. Sustain. Energy Rev. 96, 306–324. doi:10.1016/j.rser.2018.08.006
Preethi, M., Banu, J. R., Kavitha, S., Kannah, R. Y., Varjani, S., and Gunasekaran, M. (2022b). Mild hydrogen peroxide interceded bacterial disintegration of waste activated sludge for efficient biomethane production. Sci. Total Environ. 817, 152873. doi:10.1016/j.scitotenv.2021.152873
Preethi, M., Banu, J. R., Kumar, G., Tyagi, V. K., Bajhaiya, A. K., Gugulothu, P., et al. (2022a). Biohydrogen production from waste activated sludge through thermochemical mechanical pretreatment. Bioresour. Technol. 358, 127301. doi:10.1016/j.biortech.2022.127301
Preethi, M., Banu, J. R., Sharmila, V. G., Kavitha, S., Varjani, S., Kumar, G., et al. (2021a). Alkali activated persulfate mediated extracellular organic release on enzyme secreting bacterial pretreatment for efficient hydrogen production. Bioresour. Technol. 341, 125810. doi:10.1016/j.biortech.2021.125810
Preethi, M., Gunasekaran, M., Kumar, G., Karthikeyan, O. P., and Varjani, S. (2021b). Lignocellulosic biomass as an optimistic feedstock for the production of biofuels as valuable energy source: Techno-economic analysis, Environmental Impact Analysis, Breakthrough and Perspectives. Environ. Technol. Innovation 24, 102080. doi:10.1016/j.eti.2021.102080
Rajak, R. C., Jacob, S., and Kim, B. S. (2020). A holistic zero waste biorefinery approach for macroalgal biomass utilization: A review. Sci. Total Environ. 716, 137067. doi:10.1016/j.scitotenv.2020.137067
Rajput, A. A., and Visvanathan, C. (2018). Effect of thermal pretreatment on chemical composition, physical structure and biogas production kinetics of wheat straw. J. Environ. Manag. 221, 45–52. doi:10.1016/j.jenvman.2018.05.011
Ramprakash, B., and Muthukumar, K. (2014). Comparative study on the production of biohydrogen from rice mill wastewater. Int. J. Hydrogen Energy 39 (27), 14613–14621. doi:10.1016/j.ijhydene.2014.06.029
Ravindran, R., Sarangapani, C., Jaiswal, S., Lu, P., Cullen, P. J., Bourke, P., et al. (2019). Improving enzymatic hydrolysis of brewer spent grain with nonthermal plasma. Bioresour. Technol. 282, 520–524. doi:10.1016/j.biortech.2019.03.071
Reid, W. V., Ali, M. K., and Field, C. B. (2020). The future of bioenergy. Glob. change Biol. 26 (1), 274–286. doi:10.1111/gcb.14883
Rezania, S., Oryani, B., Cho, J., Talaiekhozani, A., Sabbagh, F., Hashemi, B., et al. (2020). Different pretreatment technologies of lignocellulosic biomass for bioethanol production: An overview. Energy 199, 117457. doi:10.1016/j.energy.2020.117457
Rittmann, B. E., Lee, H. S., Zhang, H., Alder, J., Banaszak, J. E., and Lopez, R. (2008). Full-scale application of focused-pulsed pre-treatment for improving biosolids digestion and conversion to methane. Water Sci. Technol. 58 (10), 1895–1901. doi:10.2166/wst.2008.547
Rorke, D., and Kana, E. G. (2016). Biohydrogen process development on waste sorghum (Sorghum bicolor) leaves: Optimization of saccharification, hydrogen production and preliminary scale up. Int. J. hydrogen energy 41 (30), 12941–12952. doi:10.1016/j.ijhydene.2016.06.112
Russo, L., and Ladisch, M. (2008). “Gaps in the research of 2nd generation transportation biofuels,” in Final report (Paris: IEA Bioenergy).
Saha, B. C., Qureshi, N., Kennedy, G. J., and Cotta, M. A. (2016). Biological pretreatment of corn stover with white-rot fungus for improved enzymatic hydrolysis. Int. Biodeterior. Biodegrad. 109, 29–35. doi:10.1016/j.ibiod.2015.12.020
Şahinkaya, S., and Sevimli, M. F. (2013). Sono-thermal pre-treatment of waste activated sludge before anaerobic digestion. Ultrason. sonochemistry 20 (1), 587–594. doi:10.1016/j.ultsonch.2012.07.006
Sankaran, R., Cruz, R. A. P., Pakalapati, H., Show, P. L., Ling, T. C., Chen, W. H., et al. (2020). Recent advances in the pretreatment of microalgal and lignocellulosic biomass: A comprehensive review. Bioresour. Technol. 298, 122476. doi:10.1016/j.biortech.2019.122476
Schröder, P., Beckers, B., Daniels, S., Gnädinger, F., Maestri, E., Marmiroli, N., et al. (2018). Intensify production, transform biomass to energy and novel goods and protect soils in Europe—a vision how to mobilize marginal lands. Sci. Total Environ. 616, 1101–1123. doi:10.1016/j.scitotenv.2017.10.209
Sethupathy, A., Arunagiri, A., Sivashanmugam, P., Banu, J. R., and Ashokkumar, M. (2020). Disperser coupled rhamnolipid disintegration of pulp and paper mill waste biosolid: Characterisation, methane production, energy assessment and cost analysis. Bioresour. Technol. 297, 122545. doi:10.1016/j.biortech.2019.122545
Sethupathy, A., and Sivashanmugam, P. (2018). Enhancing biomethane potential of pulp and paper sludge through disperser mediated polyhydroxyalkanoates. Energy Convers. Manag. 173, 179–186. doi:10.1016/j.enconman.2018.07.076
Shanthi, M., Banu, J. R., and Sivashanmugam, P. (2018). Effect of surfactant assisted sonic pretreatment on liquefaction of fruits and vegetable residue: Characterization, acidogenesis, biomethane yield and energy ratio. Bioresour. Technol. 264, 35–41. doi:10.1016/j.biortech.2018.05.054
Shanthi, M., Banu, J. R., and Sivashanmugam, P. (2019). Synergistic effect of combined pretreatment in solubilizing fruits and vegetable residue for biogas production: Hydrolysis, energy assessment. Fuel 250, 194–202. doi:10.1016/j.fuel.2019.04.001
Sharma, H. K., Xu, C., and Qin, W. (2019). Biological pretreatment of lignocellulosic biomass for biofuels and bioproducts: An overview. Waste Biomass Valorization 10 (2), 235–251. doi:10.1007/s12649-017-0059-y
Sharmila, V. G., Angappane, S., Gunasekaran, M., Kumar, G., and Banu, J. R. (2020b). Immobilized ZnO nano film impelled bacterial disintegration of dairy sludge to enrich anaerobic digestion for profitable bioenergy production: Energetic and economic analysis. Bioresour. Technol. 308, 123276. doi:10.1016/j.biortech.2020.123276
Sharmila, V. G., Banu, J. R., Kim, S. H., and Kumar, G. (2020a). A review on evaluation of applied pretreatment methods of wastewater towards sustainable H2 generation: Energy efficiency analysis. Int. J. Hydrogen Energy 45 (15), 8329–8345. doi:10.1016/j.ijhydene.2020.01.081
Sheldon, R. A. (2014). Green and sustainable manufacture of chemicals from biomass: State of the art. Green Chem. 16 (3), 950–963. doi:10.1039/c3gc41935e
Shen, F., Xiong, X., Fu, J., Yang, J., Qiu, M., Qi, X., et al. (2020). Recent advances in mechanochemical production of chemicals and carbon materials from sustainable biomass resources. Renew. Sustain. Energy Rev. 130, 109944. doi:10.1016/j.rser.2020.109944
Shirkavand, E., Baroutian, S., Gapes, D. J., and Young, B. R. (2016). Combination of fungal and physicochemical processes for lignocellulosic biomass pretreatment–A review. Renew. Sustain. Energy Rev. 54, 217–234. doi:10.1016/j.rser.2015.10.003
Shrestha, B., Hernandez, R., Fortela, D. L. B., Sharp, W., Chistoserdov, A., Gang, D., et al. (2020). A review of pretreatment methods to enhance solids reduction during anaerobic digestion of municipal wastewater sludges and the resulting digester performance: Implications to future urban biorefineries. Appl. Sci. 10 (24), 9141. doi:10.3390/app10249141
Snehya, A. V., Sundaramahalingam, M. A., Rajeshbanu, J., Anandan, S., and Sivashanmugam, P. (2021). Studies on evaluation of surfactant coupled sonication pretreatment on Ulva fasciata (marine macroalgae) for enhanced biohydrogen production. Ultrason. Sonochemistry 81, 105853. doi:10.1016/j.ultsonch.2021.105853
Solarte-Toro, J. C., Romero-García, J. M., Martínez-Patiño, J. C., Ruiz-Ramos, E., Castro-Galiano, E., and Cardona-Alzate, C. A. (2019). Acid pretreatment of lignocellulosic biomass for energy vectors production: A review focused on operational conditions and techno-economic assessment for bioethanol production. Renew. Sustain. Energy Rev. 107, 587–601. doi:10.1016/j.rser.2019.02.024
Souza, G. M., Ballester, M. V. R., de Brito Cruz, C. H., Chum, H., Dale, B., Dale, V. H., et al. (2017). The role of bioenergy in a climate-changing world. Environ. Dev. 23, 57–64. doi:10.1016/j.envdev.2017.02.008
Sridhar, P., Tyagi, R. D., Bhunia, P., Rout, P. R., Zhang, T. C., and Surampalli, R. Y. (2021). Greenhouse gas emissions in sludge ultrasonication followed by anaerobic digestion processes. Bioresour. Technol. 341, 125754. doi:10.1016/j.biortech.2021.125754
Tamilarasan, K., Banu, J. R., Kumar, M. D., Sakthinathan, G., and Park, J. H. (2019). Influence of mild-ozone assisted disperser pretreatment on the enhanced biogas generation and biodegradability of green marine macroalgae. Front. Energy Res. 7, 89. doi:10.3389/fenrg.2019.00089
Tamilarasan, K., Kavitha, S., Selvam, A., Banu, J. R., Yeom, I. T., Nguyen, D. D., et al. (2018). Cost-effective, low thermo-chemo disperser pretreatment for biogas production potential of marine macroalgae Chaetomorpha antennina. Energy 163, 533–545. doi:10.1016/j.energy.2018.08.147
Thürer, M., Tomašević, I., Stevenson, M., Qu, T., and Huisingh, D. (2018). A systematic review of the literature on integrating sustainability into engineering curricula. J. Clean. Prod. 181, 608–617. doi:10.1016/j.jclepro.2017.12.130
Tian, S. Q., Zhao, R. Y., and Chen, Z. C. (2018). Review of the pretreatment and bioconversion of lignocellulosic biomass from wheat straw materials. Renew. Sustain. Energy Rev. 91, 483–489. doi:10.1016/j.rser.2018.03.113
Toreci, I., Kennedy, K. J., and Droste, R. L. (2010). Effect of high-temperature microwave irradiation on municipal thickened waste activated sludge solubilization. Heat. Transf. Eng. 31 (9), 766–773. doi:10.1080/01457630903501039
Ullah, K., Ahmad, M., Sharma, V. K., Lu, P., Harvey, A., Zafar, M., et al. (2014). Algal biomass as a global source of transport fuels: Overview and development perspectives. Prog. Nat. Sci. Mater. Int. 24 (4), 329–339. doi:10.1016/j.pnsc.2014.06.008
Ushani, U., Banu, J. R., Kavitha, S., Kaliappan, S., and Yeom, I. T. (2017a). Immobilized and MgSO4 induced cost effective bacterial disintegration of waste activated sludge for effective anaerobic digestion. Chemosphere 175, 66–75. doi:10.1016/j.chemosphere.2017.02.046
Ushani, U., Banu, J. R., Tamilarasan, K., Kavitha, S., and Yeom, I. T. (2017b). Surfactant coupled sonic pretreatment of waste activated sludge for energetically positive biogas generation. Bioresour. Technol. 241, 710–719. doi:10.1016/j.biortech.2017.05.201
Ushani, U., Kavitha, S., Kannah, R. Y., Gunasekaran, M., Kumar, G., Nguyen, D. D., et al. (2018). Sodium thiosulphate induced immobilized bacterial disintegration of sludge: An energy efficient and cost effective platform for sludge management and biomethanation. Bioresour. Technol. 260, 273–282. doi:10.1016/j.biortech.2018.03.118
Uthirakrishnan, U., Sharmila, V. G., Merrylin, J., Kumar, S. A., Dharmadhas, J. S., Varjani, S., et al. (2022). Current advances and future outlook on pretreatment techniques to enhance biosolids disintegration and anaerobic digestion: A critical review. Chemosphere 288, 132553. doi:10.1016/j.chemosphere.2021.132553
Vani, S., Binod, P., Kuttiraja, M., Sindhu, R., Sandhya, S. V., Preeti, V. E., et al. (2012). Energy requirement for alkali assisted microwave and high pressure reactor pretreatments of cotton plant residue and its hydrolysis for fermentable sugar production for biofuel application. Bioresour. Technol. 112, 300–307. doi:10.1016/j.biortech.2012.02.076
Wagner, A. O., Lackner, N., Mutschlechner, M., Prem, E. M., Markt, R., and Illmer, P. (2018). Biological pretreatment strategies for second-generation lignocellulosic resources to enhance biogas production. Energies 11 (7), 1797. doi:10.3390/en11071797
Wang, G., Zhao, L., Liang, R., and Liu, Z. (2020). Design of remote monitoring system for sewage source heat pump based on PLC and GPRS. IOP Conf. Ser. Earth Environ. Sci. 480, 012008. IOP Publishing. doi:10.1088/1755-1315/480/1/012008
Wang, H., Cai, W. W., Liu, W. Z., Li, J. Q., Wang, B., Yang, S. C., et al. (2018). Application of sulfate radicals from ultrasonic activation: Disintegration of extracellular polymeric substances for enhanced anaerobic fermentation of sulfate-containing waste-activated sludge. Chem. Eng. J. 352, 380–388. doi:10.1016/j.cej.2018.07.029
Wang, J., and Li, Y. (2016). Synergistic pretreatment of waste activated sludge using CaO2 in combination with microwave irradiation to enhance methane production during anaerobic digestion. Appl. Energy 183, 1123–1132. doi:10.1016/j.apenergy.2016.09.042
Weber, C., Farwick, A., Benisch, F., Brat, D., Dietz, H., Subtil, T., et al. (2010). Trends and challenges in the microbial production of lignocellulosic bioalcohol fuels. Appl. Microbiol. Biotechnol. 87 (4), 1303–1315. doi:10.1007/s00253-010-2707-z
Wei, H., Gao, B., Ren, J., Li, A., and Yang, H. (2018). Coagulation/flocculation in dewatering of sludge: A review. Water Res. 143, 608–631. doi:10.1016/j.watres.2018.07.029
Xia, A., Cheng, J., Song, W., Yu, C., Zhou, J., and Cen, K. (2013). Enhancing enzymatic saccharification of water hyacinth through microwave heating with dilute acid pretreatment for biomass energy utilization. Energy 61, 158–166. doi:10.1016/j.energy.2013.09.019
Yang, S. S., Guo, W. Q., Cao, G. L., Zheng, H. S., and Ren, N. Q. (2012). Simultaneous waste activated sludge disintegration and biological hydrogen production using an ozone/ultrasound pretreatment. Bioresour. Technol. 124, 347–354. doi:10.1016/j.biortech.2012.08.007
Yin, Y., Liu, Y. J., Meng, S. J., Kiran, E. U., and Liu, Y. (2016). Enzymatic pretreatment of activated sludge, food waste and their mixture for enhanced bioenergy recovery and waste volume reduction via anaerobic digestion. Appl. Energy 179, 1131–1137. doi:10.1016/j.apenergy.2016.07.083
Yin, Z., Zhu, L., Li, S., Hu, T., Chu, R., Mo, F., et al. (2020). A comprehensive review on cultivation and harvesting of microalgae for biodiesel production: Environmental pollution control and future directions. Bioresour. Technol. 301, 122804. doi:10.1016/j.biortech.2020.122804
Yusaf, T., and Al-Juboori, R. A. (2014). Alternative methods of microorganism disruption for agricultural applications. Appl. Energy 114, 909–923. doi:10.1016/j.apenergy.2013.08.085
Zabed, H., Sahu, J. N., Suely, A., Boyce, A. N., and Faruq, G. (2017). Bioethanol production from renewable sources: Current perspectives and technological progress. Renew. Sustain. Energy Rev. 71, 475–501. doi:10.1016/j.rser.2016.12.076
Zamri, M. F. M. A., Hasmady, S., Akhiar, A., Ideris, F., Shamsuddin, A. H., Mofijur, M., et al. (2021). A comprehensive review on anaerobic digestion of organic fraction of municipal solid waste. Renew. Sustain. Energy Rev. 137, 110637. doi:10.1016/j.rser.2020.110637
Zhang, B., Ji, M., Wang, F., Li, R., Zhang, K., Yin, X., et al. (2017). Damage of EPS and cell structures and improvement of high-solid anaerobic digestion of sewage sludge by combined (Ca (OH) 2+ multiple-transducer ultrasonic) pretreatment. RSC Adv. 7 (37), 22706–22714. doi:10.1039/c7ra01060e
Zhang, M. H., Dong, H., Zhao, L., Wang, D. X., and Meng, D. (2019). A review on Fenton process for organic wastewater treatment based on optimization perspective. Sci. Total Environ. 670, 110–121. doi:10.1016/j.scitotenv.2019.03.180
Zhao, B., Sha, H., Li, J., Cao, S., Wang, G., and Yang, Y. (2020). Static magnetic field enhanced methane production via stimulating the growth and composition of microbial community. J. Clean. Prod. 271, 122664. doi:10.1016/j.jclepro.2020.122664
Zhou, J., Xu, W., Wong, J. W., Yong, X., Yan, B., Zhang, X., et al. (2015). Ultrasonic and thermal pretreatments on anaerobic digestion of petrochemical sludge: Dewaterability and degradation of PAHs. PloS one 10 (9), e0136162. doi:10.1371/journal.pone.0136162
Keywords: waste biomass, bioenergy, pretreatment, sustainable, solubilization
Citation: Preethi , M G and Banu. J R (2023) Indexing energy and cost of the pretreatment for economically efficient bioenergy generation. Front. Energy Res. 10:1060599. doi: 10.3389/fenrg.2022.1060599
Received: 03 October 2022; Accepted: 30 December 2022;
Published: 13 January 2023.
Edited by:
Marcin Debowski, University of Warmia and Mazury in Olsztyn, PolandReviewed by:
Joanna Kazimierowicz, Bialystok University of Technology, PolandArulazhagan Pugazhendi, King Abdulaziz university, Saudi Arabia
Copyright © 2023 Preethi, M and Banu. J. This is an open-access article distributed under the terms of the Creative Commons Attribution License (CC BY). The use, distribution or reproduction in other forums is permitted, provided the original author(s) and the copyright owner(s) are credited and that the original publication in this journal is cited, in accordance with accepted academic practice. No use, distribution or reproduction is permitted which does not comply with these terms.
*Correspondence: Rajesh Banu. J, cmFqZXNoY2VzQGdtYWlsLmNvbQ==