- 1Department of Ecology, Evolution and Organismal Biology, Kennesaw State University, Kennesaw, GA, United States
- 2Department of Chemistry and Biochemistry, Kennesaw State University, Kennesaw, GA, United States
The accumulation of Pb deposits in soil is a growing global concern. Soil remediation options include phytoextraction that involves the use of plants and associated soil microorganism. Switchgrass (Panicum virgatum L.), a second-generation bioenergy crop was used in this study due to its ability to produce high biomass and grow in metal polluted soils. Plants were grown in Pb-contaminated soil (5,802.5 mg kg−1) in an environmentally controlled greenhouse. Plants were treated with exogenous application of the plant growth regulator (PGR) benzylaminopurine (BAP) or complete foliar nutrient solution (Triple-12®) twice a week until harvested. Plants also received the soil fungicide propiconazole (Infuse™) that was followed by the soil chelate nitrilotriacetic acid (NTA). Two concentrations of NTA were compared (5 mM and 10 mM) and combined application of NTA (10 mM) + APG (alkyl polyglucoside). Soil fungicide (propiconazole) was used to arrest arbuscular mycorrhizal fungi (AMF) activities in the roots of switchgrass in order to enhance Pb-phytoextraction. Lead (Pb) was measured in dry plant materials using an ICP-OES. Phytoextraction by switchgrass was significantly improved by dual soil applications of 10 mM NTA, APG and foliar applications of BAP which resulted in the greatestaverage Pb concentration of 5,942 mg kg−1. The average dry mass of plants and the average value for total phytoextracted Pb (mg) per pot were significantly greatestfor plants treated with 10 mM NTA, APG and BAP. Also, plants treated with NTA and BAP showed average bioconcentration factor of 1.02. The results suggested that chemically enhanced phytoextraction significantly improved biomass production of switchgrass and at the same time increased phytoextracted Pb which is important for phytoremediation and bioenergy industry.
1 Introduction
Accumulation of lead (Pb) in soil is a growing concern due to the human health implications and associated environmental damage (Anyanwu et al., 2018; Eiró et al., 2021). Lead contamination in soils is derived from various sources, including past use of leaded gasoline, smelting and mining and impurities in pesticides and chemical fertilizers (Zhuang et al., 2006; De Araújo and Do Nascimento 2010; Greipsson et al., 2013; Wu et al., 2016; González-Chávez et al., 2019; Alengebawy et al., 2021; Mufalo et al., 2021). Lead is persistent in the topsoil, but Pb exposure can occur if soil become re-suspended (Laidlaw and Taylor, 2011; Mielke et al., 2016). Levels of Pb-contamination in soil and blood lead level (BLL) in children are strongly correlated (Laidlaw and Taylor 2011). Currently there is no safe level of Pb exposure for humans (Eiró et al., 2021; McFarland et al., 2022). Neurological dysfunctions have been associated with children who had blood Pb levels of 10 mg/L or less (Canfield et al., 2003; Skerfving et al., 2015; McFarland et al., 2022). Even, long-term exposure to low-level Pb is associated with neurological damages which includes cognitive dysfunction in children (Canfield et al., 2003; Martinez-Finley et al., 2012; Paulson and Brown, 2019), along with Alzheimer’s (Bakulski et al., 2012; Fathabadi et al., 2018) and Parkinson’s diseases (Chen et al., 2017).
The removal of Pb-contaminants from soil can be accomplished through phytoextraction, an emerging cost-effective heavy metal remediation technique (Garbisu and Alkorta 2001; Greipsson 2011; Kafle et al., 2022). Phytoextraction has the potential to remove significant amounts of heavy metal contaminants from soil and restore the impacted soil for future uses (Huang et al., 1997; Greipsson et al., 2022b). The effectiveness of phytoextraction is mainly a function of a plant’s biomass production and the level of contaminants in the harvested biomass. Phytoextraction involves the use of plants and their associated soil microbes to remove heavy metals from contaminated soils (Greipsson 2011; Yan et al., 2020; Kafle et al., 2022). Phytoextraction usually requires acidification or chelation of soil which further facilitates the uptake of Pb (Aderholt et al., 2017; Chen et al., 2022). Soil chelation may be improved based on the activation efficiency of the chelating agent used, but that is widely dependent on specific heavy metal interactions within soil (Evangelou et al., 2007; Liu et al., 2020). Ethylenediaminetetraacetic acid (EDTA) was previously identified as a successful chelator in several phytoremediation studies but has raised environmental concerns related to its limited biodegradability (Elliott and Brown 1986; Oviedo and Rodríguez 2003; Greipsson 2011; Johnson et al., 2015; Aderholt et al., 2017). To limit potential environmental impacts, biodegradable chemicals other than EDTA including nitrilotriacetic acid (NTA) and natural chelates like citric acid have been tested in phytoremediation studies as well (Freitas et al., 2013; Aderholt et al., 2017; Hart et al., 2022).
The use of plant growth regulators (PGR) have been used to increase plants biomass production in phytoextraction (Chen et al., 2022). Application of the plant growth regulator benzylaminopurine (BAP) on switchgrass was found to significantly increase biomass and shoot Pb concentrations (Aderholt et al., 2017; Hart et al., 2022). The BAP is a cytokine stimulator that has been shown to encourage plant bud growth and further stimulate multiple root growth via cell division (Bouré et al., 2019). Also, application of foliar nutrients has positively impacted growth of plants used in phytoremediation (Meers and Tack 2004).
Soil microorganisms play a critical role in the survival of plants on metal contaminated soils (Wang 2017). Arbuscular mycorrhizal fungi (AMF) are commonly found in roots of plants growing in metal contaminated soils and their presence limits Pb uptake of plants (Leung et al., 2013; Xu et al., 2014; Cabral et al., 2015; Cabral et al., 2015; Wang 2017; Sarkar et al., 2018). During phytoextraction it is important to allow plants to grow and attain great biomass, but metal uptake should be facilitated before harvest (Greipsson 2011). Application of soil fungicide can arrest AMF activities and therefore enhance Pb-phytoextraction (Hovsepyan and Greipsson, 2004; Perry et al., 2012; Hart et al., 2022). Previous studies showed that applications of the soil-fungicide benomyl improved phytoextraction of Pb (Perry et al., 2012). Another soil-fungicide, propiconazole was found to reduce AMF colonization in roots and improve Pb phytoextraction by switchgrass (Calonne et al., 2012; Aderholt et al., 2017).
Switchgrass, a second-generation bioenergy crop was used in this phytoextraction study. Switchgrass is a potential candidate for combined phytoextraction and biomass production for biofuel production (Chen et al., 2012; Balsamo et al., 2015). Furthermore, the use of switchgrass biomass for biofuel production could potentially reduce costs associated with phytoextraction. The cultivation of biofuel crops on polluted soils may improve energy security and aid in mitigating climate change (Qin et al., 2015). In addition, the cultivation of bioenergy crops on polluted soils may reduce the need for using primary agricultural lands for biofuel production.
The main aim this study was to examine the efficacy of Pb phytoextraction by switchgrass through the combined addition of chelating agent (NTA) and APG, PGR and a complete foliar nutrient solution.
2 Materials and methods
2.1 Soil
Soil was collected from a highly contaminated former Superfund site in Cedartown, Georgia, United States. This site was used as a Pb smelting operation from 1874 to 1980. The Georgia Piedmont soils are generally clay-rich acidic ultisols with low base cation saturation (Rose 1998). The mineral compositions reflect the generally granitic or gneissic parent materials of the Georgia Piedmont that have weathered to produce soils rich in quartz, feldspar, mica, Fe-oxyhydroxides, kaolinite, and illite (Franklin et al., 2007). Soil samples were randomly selected from the site and were homogenously mixed prior to planting to ensure uniform heavy metal concentrations across experimental treatments. The soil was left unsterilized in order to maintain the indigenous soil microbiota; however, debris larger than 0.5 cm were removed by hand. Chemical analysis (by the Soil, Plant, and Water Laboratory of the College of Agricultural & Environmental Sciences, University of Georgia, 2,400 College Station Road, Athens, GA 30602) demonstrated that the soil contained high concentrations of Pb (5,802.5 mg kg−1), iron (Fe) (12,316 mg kg−1) and aluminum (Al) (5,362 mg kg−1). The soil was slightly acidic with pH of 5.7. The soil was left unsterilized in order to maintain the indigenous soil microbiota; however, debris larger than 0.5 cm were removed. In addition, eleven species of arbuscular mycorrhizal fungi (AMF) were identified in the soil from Cedartown using DNA metabarcoding a high-throughput, taxonomic identification of micro-community assemblages within a soil sample.
2.2 Plant species
Switchgrass (Panicum virgatum L.) a perennial C4 grass has several characteristics coveted for phytoremediation such as high yield biomass and tolerance to heavy metals toxicity (Chen et al., 2012; Fike at al. 2017). Also, switchgrass is one of the most extensively studied plant species for ligno-cellulosic feedstock production which can be processed in biofuel (ethanol) production (Antizar-Ladislao and Turrion-Gomez, 2008; Sanderson and Adler, 2008). Switchgrass is utilized as a second-generation biofuel grass because it consistently yields acceptable harvestable biomass, has large ethanol conversion potential, and can grow under diverse environmental conditions (Hernández-Allica et al., 2008; Gomes, 2012; Boyer et al., 2013; Min et al., 2017). The Alamo cultivar of switchgrass shows low phytotoxicity to Pb when grown in contaminated soils making it suitable for Pb phytoextraction (Johnson et al., 2015).
2.3 Plant growth conditions
Switchgrass seeds were germinated in a sand culture and seedlings were transplanted into 5 L pots (18.6 cm deep and 19 cm diameter) containing the Pb-contaminated soil. Pots were filled with 5,000 g of contaminated soil. The pots were placed on wire-topped greenhouse benches with individual plastic saucers placed under each pot to prevent soil loss and cross contamination. Plants were grown under environmentally controlled conditions in the Kennesaw State University Research Greenhouse. Plants were supplemented with white, fluorescent overhead light (10,000 Lux) for approximately 16 h per day. Temperature (average 25°C) and humidity was monitored and maintained at a near-constant level. Pots were randomized every 7 days and watered three times weekly with appropriate solutions.
2.4 Experimental setup
Four replicated pots served as the control and did not receive any chemical treatment throughout the duration of the experiment. Four replicated pots were used in each of the following treatments: 1) control, 2) NTA (NTA + APG and Infuse™), 3) NTA + Nu (NTA + APG and Infuse™ and Triple-12®), 4) NTA + BAP [NTA + APG and Infuse and Promalin® (Table 1)3.].
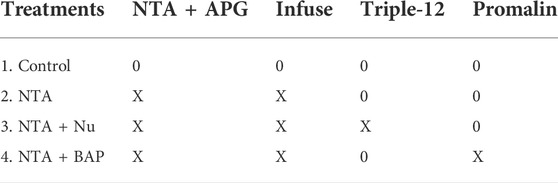
TABLE 1. Experimental design for control and treatment groups. Chemical application is indicated with X and absence of chemical application is indicated with 0.
A complete soil-nutrient solution containing: 332 mg L−1 nitrogen (N), 111 mg L−1 phosphorous (P) and 222 mg L−1 potassium (K) was supplied to all plants twice a week until 64 days after planting (dap). All plants were watered with 200 ml of water three times per week until 80 dap. After 80 dap, plants in treatments with foliar applications were sprayed three times per week. The Triple-12® foliar nutrient solution was used by mixing 5 ml Triple-12® into 1L H20. The Triple-12® foliar nutrient solution contained 12% N, 2% P and 12% K. The foliar BAP solution was made by using Promalin® solution. The soil fungicide Infuse™ (propiconazole) was applied after 152 dap. The soil-fungicide was given to all plants for two weeks for suppression of symbiotic arbuscular mycorrhizal fungi. After 166 dap the 10 mM NTA + APG solution was applied to treatments 2, 3, and 4 three times per week until plants were harvested at 179 dap. The NTA (10 mmol kg−1 soil) solution was made by mixing powdered NTA with an aqueous 10 M NaOH solution. The Triton X-100 (2%) was added to the NTA + APG solution in 100 ml/1 L increments (Hu et al., 2017).
2.5 Harvesting
Plants in all treatments and control plants were grown until fully mature. Plants were harvested following appearance of chlorosis and/or heavy metal toxicity and the presence of purple leaf tips. The longest leaf, most affected leaves (visible chlorosis) and remaining leaves were collected from mature plants in all treatments. Harvested plant leaves were placed in paper bags, labeled, and dried in an oven at 65°C for 48 h prior to acid digestion.
2.6 Acid digestion and chemical analysis
Dried plant samples were weighed, placed in 100 ml disposable acid digestion tubes (Environmental Express®, Inc.) following a modified EPA method 3,050 B and digested in a combined solution of 38% HCL (10.0 ml) and 70% HNO3 (10.0 ml) (U.S. EPA, 1996). Plant samples were capped and placed in the solution for 24 h until were mostly digested. Digestion tubes were then placed in the Hotblock Express System™ and refluxed for 55 min at 90°C–95°C (U.S. EPA, 1996). Digestion tubes were allowed to cool to room temperature. Cooled digestion tubes had deionized water added to them up to the 100 ml line and were vacuum filtered through 0.45-micron filters. Following filtration each sample was pipetted into 50 ml centrifuge tubes stored in a refrigerator until chemical analysis using inductively coupled plasma mass spectrometry (ICP-OES: Perkin Elmer Avio 200). Metals Al, Fe and Pb concentrations were identified in each sample collected and tested in triplicate against each standard. In addition, the bioconcentration factor (BCF) was calculated as the proportion of Pb concentration in plant’s foliage (Cplant) to the Pb concentration in the bulk soil (Csoil). The following equation was used: BCF = Cplant/Csoil.
2.7 Statistical analysis
Concentrations of Al, Pb and Fe were calculated and statistically analyzed through Analysis of Variance (ANOVA), average and standard deviation calculations, and Fisher’s least significant difference (LSD) for post-hoc analyses. Excel’s data analysis package along with appropriate R studio’s packages were used to perform the statistical tests (RStudio Team, 2021). The main statistical method used for analysis was one-way ANOVA through Excel. Data reorganization, determination of ANOVA type and data variance were verified in the R studio (RStudio Team, 2021). The ANOVA tables were calculated to identify interactions between variables. Fisher’s (LSD) was used to determine the significance of p-values. Statistically significant p-values were accepted at p < 0.05 and 5% (LSD) confidence levels, α = 0.05.
3 Results
Chlorosis of leaves was first observed following NTA applications after 170 dap. During the continued applications of NTA, the tips of plant leaves turned yellow and eventually purple as the Pb toxicity progressed. The average dry mass of the foliage was significantly greatestfor plants treated with 10 mM NTA and BAP (Figure 1). The dry mass of the foliage was doubled by the foliar BAP application compared to control plants (Figure 1). Phytoextraction of Pb was improved by treating the Pb contaminated soil with 10 mM NTA instead of 5 mM NTA. Plants treated with 5 mM NTA and BAP had an average Pb concentration of 858 mg kg−1 in the plant’s foliage. This was improved further when plants were treated with 10 mM NTA alone resulting in an average Pb concentration of 2,914 (mg kg−1) Figure 2). Plants treated with 10 mM NTA and BAP showed an average Pb concentration of 5,942 mg kg−1 the greatest one across all treatments (Figure 2). High variation in Pb concentration in plants was observed and one plant in the 10 mM NTA and BAP treatment showed the greatest Pb concentration of 10,820 mg kg−1 (Figure 2). The average value for phytoextracted Pb (mg) per pot was significantly greatestfor plants treated with 10 mM NTA and BAP (Figure 3). Whereas control plants showed the lowest average value for phytoextracted Pb per pot (Figure 3). The average value for phytoextracted Pb was more than doubled by foliar application of BAP (NTA + BAP) compared to NTA application alone (Figure 3). Other metals showed different trends in metal accumulation in plant’s foliage. Plants treated with BAP showed lower average concentration of Al in plant’s foliage than plants treated with foliar nutrients or just 10 mM NTA alone, although significant differences were not established (Figure 4). However, control plants showed significantly the lowest average concentration of Al (Figure 4). Plants treated with BAP showed greater average concentration of Fe in plant’s foliage than plants treated with foliar nutrients or just 10 mM NTA alone, although significant differences were not established (Figure 5). However, control plants showed significantly the lowest average concentration of Fe (Figure 5). The bioconcentration factor was greatest for plants treated with NTA and BAP (1.03), suggesting great phytoextraction efficiency.
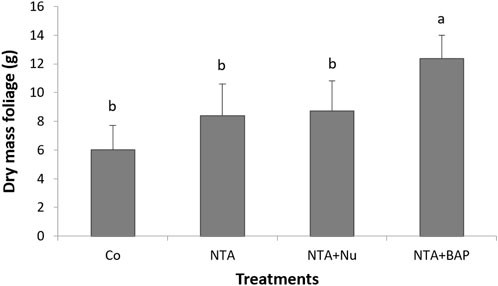
FIGURE 1. Average dry mass (g) (+SD) of Panicum virgatum per pot after final harvest. Means for columns with same letters are not significantly different (α = 0.05). (Treatments: NTA—10mM NTA, Nu—foliar nutrients application of Triple-12®, BAP- Promalin® foliar application).
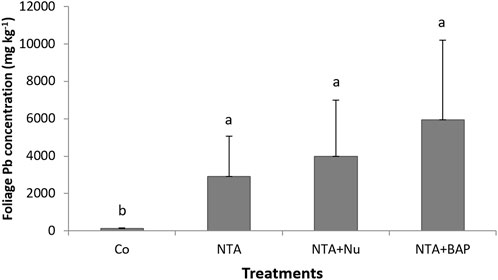
FIGURE 2. Average Pb concentration (mg kg−1) (+SD) of Panicum virgatum in different treatments. Treatments: NTA—10 mM NTA, Nu—foliar nutrients application of Triple-12®, BAP- Promalin® foliar application Means for columns with same letters are not significantly different (α = 0.05).
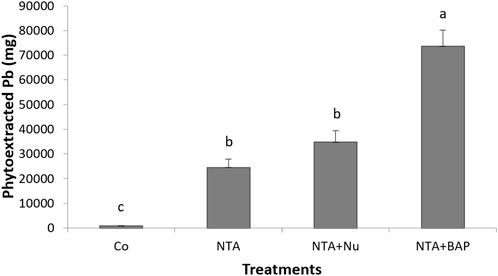
FIGURE 3. Phytoextracted Pb (g) (+SD) per pot. Treatments: NTA—10 mM NTA, Nu—foliar nutrients application of Triple-12®, BAP- Promalin® foliar application Means for columns with same letters are not significantly different (α = 0.05).
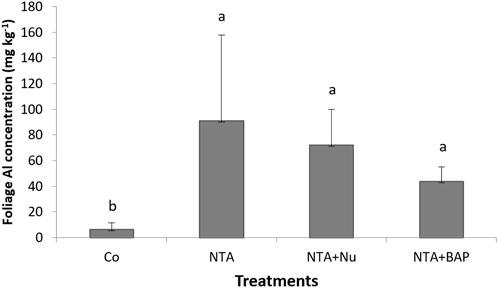
FIGURE 4. Average Al concentration (mg kg−1) (+SD) of Panicum virgatum in different treatments. Treatments: NTA—10 mM NTA, Nu—foliar nutrients application of Triple-12®, BAP- Promalin® foliar application Means for columns with same letters are not significantly different (α = 0.05).
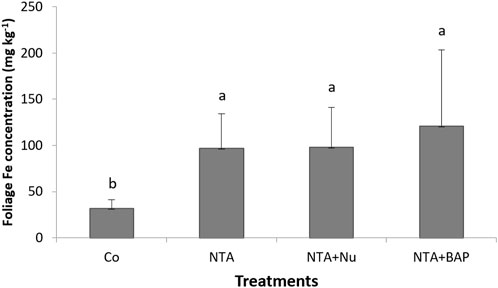
FIGURE 5. Average Fe concentration (mg kg−1) (+SD) of Panicum virgatum in different treatments. Treatments: NTA—10 mM NTA, Nu—foliar nutrients application of Triple-12®, BAP- Promalin® foliar application. Means for columns with same letters are not significantly different (α = 0.05).
4 Discussion
This study was conducted to determine if phytoextraction of Pb-contaminated soil by switchgrass can be improved by soil applications of a chelate (10 mM NTA) and APG (Triton X-100), soil-fungicide (Infuse™) and foliar applications of a PGR (BAP). The average value for phytoextracted Pb (mg) per pot was significantly highest for plants treated with 10 mM NTA, APG and BAP. Also, an average bioconcentration factor of 1.03 was greatest for plants treated with NTA and BAP. This value suggests a great phytoextraction efficiency for the NTA and BAP treatment. This study showed that the average Pb concentrations in the foliage of plants could be ranked by treatments as following: NTA (5 mM) (858 mg kg−1 Pb) < NTA (10 mM) (2,914 mg kg−1 Pb) < NTA (10 mM) and foliar application of the complete nutrient solution (3,987 mg kg−1 Pb) < NTA (10 mM) + APG and foliar application of BAP (5,942 mg kg−1). The results are consistent with previous studies that indicated that Pb-phytoextraction by switchgrass could be optimized by the use of phytohormones and varying chelating agents (Aderholt et al., 2017; Greipsson et al., 2022a). Previous studies demonstrated that application of EDTA with the addition of phytohormone BAP and citric acid showed more Pb uptake efficacy compared to EDTA alone (Johnson et al., 2015; Aderholt et al., 2017). Furthermore, exogenous applications of PGR have been associated with an increase in phytoextraction efficiency (Beavers et al., 2021). The combined applications of PGRs, chelating agents, and soil-fungicide to suppress AMF activities yielded higher levels of Pb in the phytoextraction processes (Beavers et al., 2021; Hart et al., 2022). A more effective phytoremediation process could help reclaim contaminated soils in a shorter time period and would limit heavy metal exposure to individuals in sensitive populations (Filippelli and Laidlaw 2010; Fasinu and Orisakwe 2013; Nissim and Labreccque 2021).
The effects of PGRs on plant growth and Pb translocation was previously outlined (Aderholt et al., 2017). PGRs like BAP have increased growth of switchgrass and improved phytoextraction (Hart et al., 2022). Previous studies have shown that exogenous applications of PGRs were associated with an increase in Pb-phytoextraction (Greipsson et al., 2022a). Also, previous study has shown that phytoextraction processes were improved with combined foliar applications of SNP (nitric oxide donor) and GA3 (gibberellic acid) (Beavers et al., 2021). A recent study suggested that combined applications of SA alongside the PGR DA-6 (diethyl aminoethyl hexanoate) was associated with an increase in Pb uptake (Greipsson et al., 2022a).
Using second-generation bioenergy crop such as switchgrass for phytoremediation of contaminated soils has shown great potentials (Shen et al., 2002; Van Ginneken et al., 2007; Hernández-Allica et al., 2008; Chen et al., 2012; Oh et al., 2013; Balsamo et al., 2015; Min et al., 2017; Quarshie et al., 2021). This is largely due to the plants amount of harvestable biomass, ethanol conversion potential and its ability to be grown in diverse climates as a remediator (Hernández-Allica et al., 2008; Boyer et al., 2013; Min et al., 2017). Planting contaminated sites with biofuel crops and maintaining them as phytoextraction sites could potentially remediate Pb soil contamination, with the long-term goal of reclaiming the marginal land for future uses. Cultivation of bioenergy crops on marginal lands may reduce the need for using primary agricultural lands for biofuel production and might therefore improve energy security and aid in mitigating climate change (Qin et al., 2012; Qin et al., 2015).
Optimizing phytoextraction efficiency of bioenergy crops has significant implications for bioenergy production (Balsamo et al., 2015). This study showed that foliar application of BAP improved the biomass of switchgrass. Improving biomass of plants used in phytoextraction is an important task. The Alamo cultivar of switchgrass used in this study can produce 17,800 kg ha−1 of harvestable dry mass (Smith, Allen and Barney 2015). Also, biomass production of switchgrass is economical compared to the production of other high biomass crops (McLaughlin and Kszos 2005).
As demand for remediation work in brownfield sites grows, so does its association with phytoremediation as these sites have high potentials for reclamation (Nissim and Labreccque 2021). Successful removal of Pb from contaminated soils may benefit more sensitive human populations who are at risk of Pb exposure. Often contaminated farm sites lack access to additional viable soil and remediation is a clear path to continued vegetation growth or utilization of sites (Yan et al., 2020). There is a growing need for additional advances in effective phytoremediation practices to sustain the environment in affected cities (Anyanwu et al., 2018; Lee et al., 2021). Continued phytoremediation research on the reclamation of brownfield sites and contaminated farmland may benefit the environment and associated redevelopment projects (Mielke et al., 2016; Anyanwu et al., 2018). Further study is required to identify the effects of increased concentrations of additional chemical chelates for switchgrass used in phytoextraction. Future experiments can specifically explore increased NTA applications in combination with APG to determine if the additional concentrations continue to be positively associated with Pb uptake. Continued research focusing on the benefits of APGs, PGRs and chemical chelate applications within phytoremediation could broaden areas of interest with current knowledge gaps.
5 Conclusion
Phytoextraction of Pb-contaminated soil by switchgrass that was chemically enhanced with chelates, APG, soil fungicide and PGR has many implications for both phytoextraction and bioenergy industry. This study demonstrated that switchgrass treated with foliar application of a PGR (BAP) resulted in increased dry mass production of switchgrass which is important in both phytoextraction and bioenergy industry as well. Also, phytoextraction was improved using a chelate (NTA) and APG. The use of NTA has been emphasized in phytoextraction due to the long persistence time of EDTA in soil and potential mobilization of Pb into groundwater. Total phytoextraction was greatest in plants treated with combined application of soil applications of a chelate (10 mM NTA) and APG (Triton X-100), soil-fungicide (Infuse™) and foliar applications of a PGR (BAP). These findings strongly suggest that phytoextraction can be improved through judicious chemical application and at the same time enhancing switchgrass biomass production.
Data availability statement
The raw data supporting the conclusion of this article will be made available by the authors, without undue reservation.
Author contributions
Conceptualization, SG; methodology, SG, TM, and MK; formal analysis, GH and AG; investigation, SG, GH, AG, MK, and TM; resources, SG, and MK; data curation, SG and MK; writing—original draft preparation, SG, GH, and AG, writing—review and editing, SG, MK, GH, AG, and TM; supervision, SG, TM, and MK; project administration, SG; funding acquisition, SG, MK, and TM. All authors have read and agreed to the published version of the manuscript.
Funding
This study was financially supported by a grant from the National Science Foundation, Environmental Engineering Program (award # 1705924).
Acknowledgments
We would like to thank Nick Sbravati for his help.
Conflict of interest
The authors declare that the research was conducted in the absence of any commercial or financial relationships that could be construed as a potential conflict of interest.
Publisher’s note
All claims expressed in this article are solely those of the authors and do not necessarily represent those of their affiliated organizations, or those of the publisher, the editors and the reviewers. Any product that may be evaluated in this article, or claim that may be made by its manufacturer, is not guaranteed or endorsed by the publisher.
References
Aderholt, M., Vogelien, D., Koether, M., and Greipsson, S. (2017). Phytoextraction of contaminated urban soils by Panicum virgatum L. enhanced with application of a plant growth regulator (BAP) and citric acid. Chemosphere 15-16, 20–74. doi:10.1016/j.chemosphere.2017.02.022
Alengebawy, A., Abdelkhalek, S., Qureshi, S., and Wang, M. (2021). Heavy metals and pesticides toxicity in agricultural soil and plants: Ecological risks and human health implications. Toxics 9 (3), 42. doi:10.3390/toxics9030042
Antizar-Ladislao, B., and Turrion-Gomez, J. L. (2008). Second-generation biofuels and local bioenergy systems. Biofuel. Bioprod. Biorefin. 2, 455–469. doi:10.1002/bbb.97
Anyanwu, B., Ezejiofor, A., Igweze, Z., and Orisakwe, O. (2018). Heavy metal mixture exposure and effects in developing nations: An update. Toxics 6 (6), 1–32. doi:10.3390/toxics6040065
Bakulski, K., Rozek, L., Dolinoy, D., Paulson, H., and Hu, H. (2012). Alzheimer’s disease and environmental exposure to lead: The epidemiologic evidence and potential role of epigenetics. Curr. Alzheimer Res. 9, 563–573. doi:10.2174/156720512800617991
Balsamo, R., Kelly, W., Satrio, J., Ruiz-Felix, M., Fetterman, M., Wynn, R., et al. (2015). Utilization of grasses for potential biofuel production and phytoremediation of heavy metal contaminated soils. Int. J. Phytoremediation 17 (1-6), 448–455. doi:10.1080/15226514.2014.922918
Beavers, A., Koether, M., McElroy, T., and Greipsson, S. (2021). Effects of exogenous application of plant growth regulators (SNP and GA3) on phytoextraction by switchgrass (Panicum virgatum L.) grown in lead (Pb) contaminated soil. Sustainability 13 (19), 10866. doi:10.3390/su131910866
Bouré, N., Kumar, V., and Arnaud, N. (2019). The BAP module: A multisignal integrator orchestrating growth. Trends Plant Sci. 24 (7), 602–610. doi:10.1016/j.tplants.2019.04.002
Boyer, C., Roberts, R., English, B., Tyler, D., Larson, J., and Mooney, D. (2013). Effects of soil type and landscape on yield and profit maximizing nitrogen rates for switchgrass production. Biomass Bioenergy 48, 33–42. doi:10.1016/j.biombioe.2012.11.004
Cabral, L., Soares, C., Giachini, A., and Siqueira, J. (2015). Arbuscular mycorrhizal fungi in phytoremediation of contaminated areas by trace elements: Mechanisms and major benefits of their applications. World J. Microbiol. Biotechnol. 31 (11), 1655–1664. doi:10.1007/s11274-015-1918-y
Calonne, M., Sahraoui, A. L. H., Campagnac, E., Debiane, D., Laruelle, F., Grandmougin-Ferjani, A., et al. (2012). Propiconazole inhibits the sterol 14α-demethylase in Glomus irregulare like in phytopathogenic fungi. Chemosphere 87 (4), 376–383. doi:10.1016/j.chemosphere.2011.12.027
Canfield, R., Henderson, R., Cory-Slechta, D., Cox, C., Jusko, T., and Lanphear, B. (2003). Intellectual Impairment in children with blood lead concentrations below 10 μg per Deciliter. N. Engl. J. Med. Overseas. Ed. 348, 1517–1526. doi:10.1056/nejmoa022848
Chen, B., Lai, H., and Juang, K. (2012). Model evaluation of plant metal content and biomass yield for the phytoextraction of heavy metals by switchgrass. Ecotoxicol. Environ. Saf. 80, 393–400. doi:10.1016/j.ecoenv.2012.04.011
Chen, H., Kwong, J., Copes, R., Tu, K., Villeneuve, P., van Donkelaar, A., et al. (2017). Living near major roads and the incidence of Dementia, Parkinson’s disease, and multiple sclerosis: A population-based cohort study. Lancet 389, 718–726. doi:10.1016/S0140-6736(16)32399-6
Chen, L., Beiyuan, J., Hu, W., Zhang, Z., Duan, C., Cui, Q., et al. (2022). Phytoremediation of potentially toxic elements (PTEs) contaminated soils using alfalfa (Medicago sativa L.): A comprehensive review. Chemosphere 293, 133577. doi:10.1016/j.chemosphere.2022.133577
De Araújo, J., and Do Nascimento, C. (2010). Phytoextraction of lead from soil from a battery recycling site: The use of citric acid and NTA. Water, Air, & Soil Pollut. 211, 113–120. doi:10.1007/s11270-009-0285-4
Eiró, L., Ferreira, M., Frazão, D., Aragão, W., Souza-Rodrigues, R., Fagundes, N., et al. (2021). Lead exposure and its association with neurological damage: Systematic review and meta-analysis. Environ. Sci. Pollut. Res. 28, 37001–37015. doi:10.1007/s11356-021-13536-y
Elliott, H., and Brown, G. (1986). Comparative evaluation of NTA and EDTA for extractive decontamination of Pb-polluted soils. Water Air Soil Pollut. 45 (45), 3–4. doi:10.1007/BF00283464
Evangelou, M., Ebel, M., and Schaeffer, A. (2007). Chelate assisted phytoextraction of heavy metals from soil. Effect, mechanism, toxicity, and fate of chelating agents. Chemosphere 68, 989–1003. doi:10.1016/j.chemosphere.2007.01.062
Fasinu, P., and Orisakwe, O. (2013). Heavy metal pollution in sub-Saharan Africa and possible implications in cancer epidemiology. Asian Pac. J. Cancer Prev. 14, 3393–3402. doi:10.7314/apjcp.2013.14.6.3393
Fathabadi, B., Dehghanifiroozabadi, M., Aaseth, J., Sharifzadeh, G., Nakhaee, S., Rajabpour-Sanati, A., et al. (2018). Comparison of blood lead levels in patients with Alzheimer’s disease and healthy people. Am. J. Alzheimers Dis. Other Demen. 33, 541–547. doi:10.1177/1533317518794032
Fike, J., Pease, J., Owens, V., Farris, R., Hansen, J., Heaton, E., et al. (2017). Switchgrass nitrogen response and estimated production costs on diverse sites. GCB Bioenergy 9, 1526–1542. doi:10.1111/gcbb.12444
Filippelli, G., and Laidlaw, M. (2010). The elephant in the playground: Confronting lead-contaminated soils as an important source of lead burdens to urban populations. Perspect. Biol. Med. 53 (1), 31–45. doi:10.1353/pbm.0.0136
Franklin, D. H., West, L. T., Radcliffe, D. E., and Hendrix, P. F. (2007). Characteristics and Genesis of preferential flow paths in a piedmont ultisol. Soil Sci. Soc. Am. J. 71, 752–758. doi:10.2136/sssaj2003.0166
Freitas, E. V., Nascimento, C. W., Souza, A., and Silva, F. B. (2013). Citric acid-assisted phytoextraction of lead: A field experiment. Chemosphere 92 (2), 213–217. doi:10.1016/j.chemosphere.2013.01.103
Garbisu, C., and Alkorta, I. (2001). Phytoextraction: A cost-effective plant-based technology for the removal of metals from the environment. Bioresour. Technol. 77 (3), 229–236. doi:10.1016/S0960-8524(00)00108-5
Gomes, H. (2012). Phytoremediation for bioenergy: Challenges and opportunities. Environ. Technol. Rev. 1 (1), 59–66. doi:10.1080/09593330.2012.696715
González-Chávez, M., Carrillo-González, R., Cuellar-Sánchez, A., Delgado-Alvarado, A., Suárez-Espinosa, J., Ríos-Leal, E., et al. (2019). Phytoremediation assisted by mycorrhizal fungi of a Mexican defunct lead-acid battery recycling site. Sci. Total Environ. 650 (2), 3134–3144. doi:10.1016/j.scitotenv.2018.10.031
Greipsson, S., Koether, M., and McElroy, T. (2022a). Effects of supplementary nutrients (soil-nitrogen or foliar-iron) on switchgrass (Panicum virgatum L.) grown in Pb-contaminated soil. J. Plant Nutr. 45 (19), 2919–2930. doi:10.1080/01904167.2022.2068433
Greipsson, S., McElroy, T., and Koether, M. (2022b). Foliar application of Salicylic acid and DA-6 on Swithgrass (Panicum virgatum L.) grown in Pb-contaminated soil; implications for phytoextraction. Commun. Soil Sci. Plant Analysis 53 (16), 2045–2053. doi:10.1080/00103624.2022.2070193
Greipsson, S., Tay, C. F., Whatley, A., and Deocampo, D. (2013). Sharp decline in lead contamination in topsoil away from a smelter and lead migration in Ultisol. World Environ. 3 (3), 102–107. doi:10.5923/j.env.20130303.05
Hart, G., Koether, M., McElroy, T., and Greipsson, S. (2022). Evaluation of chelating agents used in phytoextraction by switchgrass of lead contaminated soil. Plants 11, 1012. doi:10.3390/plants11081012
Hernández-Allica, J., Becerril, J., and Garbisu, C. (2008). Assessment of the phytoextraction potential of high biomass crop plants. Environ. Pollut. 152 (1), 32–40. doi:10.1016/j.envpol.2007.06.002
Hovsepyan, A., and Greipsson, S. (2004). Effect of arbuscular mycorrhizal fungi on phytoextraction by corn (Zea mays) of lead-contaminated soil. Int. J. Phytoremediation 6 (4), 305–321. doi:10.1080/16226510490888820
Hu, X., Liu, X., Zhang, X., Cao, L., Chen, J., and Yu, H. (2017). Increased accumulation of Pb and Cd from contaminated soil with Scirpus triqueter by the combined application of NTA and APG. Chemosphere 188, 397–402. doi:10.1016/j.chemosphere.2017.08.173
Johnson, D., Deocampo, D., El-Mayas, H., and Greipsson, S. (2015). Induced phytoextraction of lead through chemical manipulation of switchgrass and corn; role of iron supplement. Int. J. Phytoremediation 17 (12), 1192–1203. doi:10.1080/15226514.2015.1045134
Kafle, A., Timilsina, A., Gautam, A., Adhikari, K., Bhattarai, A., and Aryal, N. (2022). Phytoremediation: Mechanisms, plant selection and enhancement by natural and synthetic agents. Environ. Adv. 8, 100203. doi:10.1016/j.envadv.2022.100203
Laidlaw, M. A., and Taylor, M. P. (2011). Potential for childhood lead poisoning in the inner cities of Australia due to exposure to lead in soil dust. Environ. Pollut. 159, 1–9. doi:10.1016/j.envpol.2010.08.020
Lee, J., Kaunda, R., Sinkala, T., Workman, C., Bazilian, M., and Clough, G. (2021). Phytoremediation and phytoextraction in sub-Saharan Africa: Addressing economic and social challenges. Ecotoxicol. Environ. Saf. 226, 112864. doi:10.1016/j.ecoenv.2021.112864
Leung, H., Wang, Z., Ye, Z., Yung, K., Peng, X., and Cheung, K. (2013). Interactions between arbuscular mycorrhizae and plants in phytoremediation of metal-contaminated soils: A review. Pedosphere 23, 549–563. doi:10.1016/s1002-0160(13)60049-1
Liu, L., Luo, D., Yao, G., Huang, X., Wei, L., Liu, Y., et al. (2020). Comparative activation process of Pb, Cd and Tl Using chelating agents from contaminated red soils. Int. J. Environ. Res. Public Health 17 (2), 497. doi:10.3390/ijerph17020497
Martinez-Finley, E., Chakraborty, S., Fretham, S., and Aschner, M. (2012). Cellular transport and homeostasis of essential and nonessential metals. Metallomics 4, 593–605. doi:10.1039/c2mt00185c
McFarland, M., Hauer, M., and Reuben, A. (2022). Half of US population exposed to adverse lead levels in early childhood. Proc. Natl. Acad. Sci. U. S. A. 119 (11), e2118631119. doi:10.1073/pnas.2118631119
McLaughlin, S., and Kszos, L. (2005). Development of switchgrass (Panicum virgatum) as a bioenergy feedstock in the United States. Biomass Bioenergy 28 (6), 515–535. doi:10.1016/j.biombioe.2004.05.006
Meers, E., and Tack, F. (2004). The potential of foliar treatments for enhanced phytoextraction of heavy metals from contaminated soil. Remediation 14, 111–123. doi:10.1002/rem.20025
Mielke, H. W., Conzales, C. R., Powell, E. T., and Mielke, P. W. (2016). Spatiotemporal dynamic transformations of soil lead and children's blood lead ten years after Hurricane Katrina: New grounds for primary prevention. Environ. Int. 94, 567–575. doi:10.1016/j.envint.2016.06.017
Min, D., Guragain, Y., Prasad, V., Vadlani, P., and Lee, J. (2017). Effects of different genotypes of switchgrass as a bioenergy crop on yield components and bioconversion potential. J. Sustain. Bioenergy Syst. 7, 27–35. doi:10.4236/jsbs.2017.71003
Nissim, W., and Labreccque, M. (2021). Reclamation of urban brownfields through phytoremediation: Implications for building sustainable and resilient towns. Urban For. Urban Green. 65, 127364. doi:10.1016/j.ufug.2021.127364
Oviedo, C., and Rodríguez, J. (2003). EDTA: The chelating agent under environmental scrutiny. Quim. Nova 26 (6), 901–905. doi:10.1590/S0100-40422003000600020
Paulson, J. A., and Brown, M. (2019). The CDC blood lead reference value for children: Time for a change. Environ. Health 18, 16. doi:10.1186/s12940-019-0457-7
Perry, R., El-Mayas, H., Krogstadt, E., and Greipsson, S. (2012). Chemically enhanced phytoextraction of lead-contaminated soils. Int. J. Phytoremediation 14, 703–713. doi:10.1080/15226514.2011.619236
Qin, Z., Zhaung, Q., and Cai, X. (2015). Bioenergy crop productivity and potential climate change mitigation from marginal lands in the United States: An ecosystem modeling perspective. GCB Bioenergy 7, 1211–1221. doi:10.1111/gcbb.12212
Quarshie, S., Xiao, X., and Zhang, L. (2021). Enhanced phytoremediation of soil heavy metal pollution and commercial utilization of harvested plant biomass: A review. Water Air Soil Pollut. 232, 475. doi:10.1007/s11270-021-05430-7
Rose, S. (1998). Anion adsorption and desorption characteristics of a piedmont ultisol: Some implications for the fate of sulfate deposition. Water Air Soil Pollut. 101, 333–347. doi:10.1023/a:1004916724390
RStudio Team (2021). RStudio: Integrated development environment for R. Boston, MA: RStudio, PBC. http://www.rstudio.com/.
Sanderson, M. A., and Adler, P. R. (2008). Perennial forages as second generation bioenergy crops. Int. J. Mol. Sci. 9 (9), 768–788. doi:10.3390/ijms9050768
Sarkar, A., Wang, Q., Asaeda, T., Kaneko, Y., and Rashid, M. H. (2018). Arbuscular mycorrhiza confers lead tolerance and uptake in Miscanthus sacchariflorus. Chem. Ecol. 34 (5), 454–469. doi:10.1080/02757540.2018.1437150
Shen, Z. G., Li, X. D., Wang, C. C., Chen, H. M., and Chua, H. (2002). Lead phytoextraction from contaminated soil with high-biomass plant species. J. Environ. Qual. 31 (6), 1893–1900. doi:10.2134/jeq2002.1893
Skerfving, S., Löfmark, L., Lundh, T., Mikoczy, Z., and Strömberg, U. (2015). Late effects of low blood lead concentrations in children on school performance and cognitive functions. Neurotoxicology 49, 114–120. doi:10.1016/j.neuro.2015.05.009
U.S. EPA (1996). Method 3050B: Acid digestion of sediments, sludges, and soils, revision 2. Washington, DC, USA: U.S. EPA.
Wang, F. (2017). Occurrence of arbuscular mycorrhizal fungi in mining-impacted sites and their contribution to ecological restoration: Mechanisms and applications. Crit. Rev. Environ. Sci. Technol. 47 (20), 1901–1957. doi:10.1080/10643389.2017.1400853
Wu, X., Cobbina, S., Mao, G., Xu, H., Zhang, Z., and Yang, L. (2016). A review of toxicity and mechanisms of individual and mixtures of heavy metals in the environment. Environ. Sci. Pollut. Res. 23 (9), 8244–8259. doi:10.1007/s11356-016-6333-x
Xu, Z., Ban, Y., Li, Z., Hui, C., Yang, R., and Tang, M. (2014). Arbuscular mycorrhizal fungi play a role in protecting roots of Sophora viciifolia Hance. from Pb damage associated with increased phytochelatin synthase gene expression. Environ. Sci. Pollut. Res. 21, 12671–12683. doi:10.1007/s11356-014-3209-9
Yan, A., Wang, Y., Tan, S. N., Yusof, M., Ghosh, S., and Chen, Z. (2020). Phytoremediation: A promising approach for revegetation of heavy metal-polluted land. Front. Plant Sci. 11, 359. doi:10.3389/fpls.2020.00359
Keywords: APG, phytoextraction, second-generation bioenergy crop, phytoremediation, plant growth regulators, remediation, soil restoration
Citation: Hart G, Gilly A, Koether M, McElroy T and Greipsson S (2022) Phytoextraction of lead (Pb) contaminated soil by switchgrass (Panicum virgatum L): Impact of BAP and NTA applications. Front. Energy Res. 10:1032404. doi: 10.3389/fenrg.2022.1032404
Received: 30 August 2022; Accepted: 24 October 2022;
Published: 07 November 2022.
Edited by:
Liandong Zhu, Wuhan University, ChinaReviewed by:
Dan Wang, School of Metallurgy and Environment, Central South University, ChinaLijun Liu, Huazhong Agricultural University, China
Copyright © 2022 Hart, Gilly, Koether, McElroy and Greipsson. This is an open-access article distributed under the terms of the Creative Commons Attribution License (CC BY). The use, distribution or reproduction in other forums is permitted, provided the original author(s) and the copyright owner(s) are credited and that the original publication in this journal is cited, in accordance with accepted academic practice. No use, distribution or reproduction is permitted which does not comply with these terms.
*Correspondence: Sigurdur Greipsson, c2dyZWlwc3NAa2VubmVzYXcuZWR1