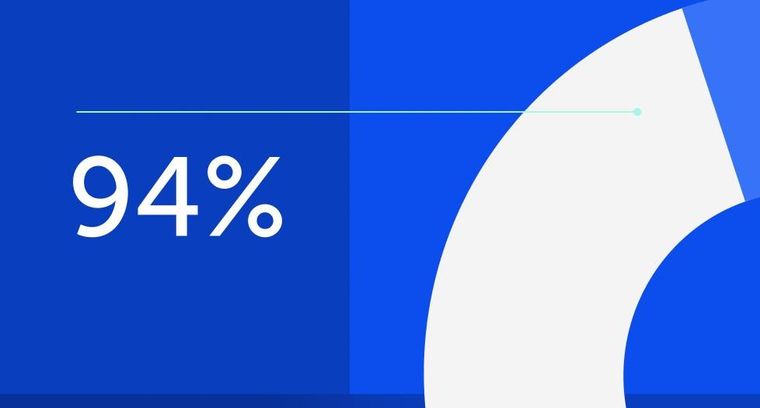
94% of researchers rate our articles as excellent or good
Learn more about the work of our research integrity team to safeguard the quality of each article we publish.
Find out more
MINI REVIEW article
Front. Energy Res., 28 October 2022
Sec. Carbon Capture, Utilization and Storage
Volume 10 - 2022 | https://doi.org/10.3389/fenrg.2022.1016406
Catalytic conversion of the greenhouse gas CO2 into value-added chemicals and fuels is highly beneficial to the environment, the economy, and the global energy supply. Metal–organic frameworks (MOFs) are promising catalysts for this purpose due to their uniquely high structural and chemical tunability. In the catalyst discovery process, computational chemistry has emerged as an essential tool as it can not only aid in the interpretation of experimental observations but also provide atomistic-level insights into the catalytic mechanism. This Mini Review summarizes recent computational studies on MOF-catalyzed CO2 conversion through different types of reactions, discusses about the usage of various computational methods in those works, and provides a brief perspective of future works in this field.
Reducing the emissions of greenhouse gases, primarily CO2, into the atmosphere to address climate change has become an urgent, global environmental issue in recent years. Numerous efforts have been made to develop efficient technologies for physically capturing CO2 from the air via adsorption into solid-state materials or absorption into liquids (Yu et al., 2012). Going one step further, CO2 can also be chemically converted into value-added chemicals and fuels, which not only reduces CO2 emissions but also produces a useful feedstock for the chemical industry and boosts the global energy supply (Huang and Tan, 2014). However, CO2 is highly stable and fairly inert, which makes such conversions energy intensive. If the energy cost would result in a net positive flow of CO2, then the process is not accomplishing the task of reducing CO2 emission. Developing efficient catalysts for CO2 conversion under mild conditions is therefore necessary for making those reactions practically useful.
Recently, a large number of metal–organic framework (MOF) materials have been designed as CO2 conversion catalysts and have shown promising performances (Beyzavi et al., 2015; Li et al., 2018; Li et al., 2020; Zhao et al., 2021a). MOFs are crystalline, nanoporous materials formed by the assembly of inorganic nodes and organic linkers in a combinatorial fashion, which enables fine-tuning of their structural and chemical properties via atomically precise design. The excellent structural integrity of MOFs also greatly facilitates computational studies, which often require well-defined atomic coordinates as starting points. Due to the rapid development in methods, algorithms and computing power, computational chemistry has become an essential tool in the investigation of a wide variety of chemical problems. Computational studies not only complement experiments by delivering atomistic-level explanation on experimental observations but also possess increasing predictive power in searching for molecules/materials with even better performance in a certain application. Indeed, many synergistic computational-experimental studies on MOFs for catalytic CO2 conversion have been published in recent years, which will be discussed in the following sections of this Mini Review.
The most studied route of catalytic CO2 conversion into value-added chemicals is the cycloaddition between CO2 and epoxides (Figure 1), which produces five-membered cyclic carbonates (CCs). CCs are versatile chemicals which can be utilized as solvents, battery electrolytes and building blocks in the synthesis of plastics and pharmaceuticals. Schaffner et al. (2010) This reaction also has a remarkable atom economy of 100%. Since the first report of MOF-catalyzed cycloaddition between CO2 and epoxides in 2009 (Song et al., 2009), a wide variety of MOFs have been tested for this reaction, in which the catalytic mechanism could vary significantly among different MOFs.
Cluster-based density functional theory (DFT) calculations have been routinely employed to carve out the active site cluster from the periodic MOF structure, map out the reaction pathways and calculate the energy barriers to elucidate the catalytic mechanism (Table 1; Figure 2). Beyzavi et al. (2014) discovered that the MOF Hf-NU-1000 can catalyze the epoxide ring-opening step of this reaction by donating protons from the ligated aqua and OH ligands on its Hf-node. For aromatic substrates, the aromatic rings in the linker can further stabilize the transition state (TS) via π-stacking. Kathalikkattil et al. (2015) synthesized and studied an eco-friendly Zn-glutamate MOF which catalyzes CO2-propylene oxide (PO) cycloaddition through synergistic involvement of Lewis acid (Zn), Lewis base (amino groups in glutamate) and ring-opening nucleophile (bromide anion from the co-catalyst tetrabutylammonium bromide (TBAB)). Hu et al. (2018) studied the same reaction catalyzed by the Cr-MIL-101 MOF in the presence of TBAB and certified the importance of the synergistic catalytic effect between Lewis acid (Cr) and nucleophile (TBAB). The co-catalyzed reaction was found to have a much lower barrier (18 kcal/mol) than the Cr-MIL-101-alone catalyzed (47 kcal/mol) and the TBAB-alone catalyzed (27 kcal/mol) reactions. Similar synergistic effects were observed by Rachuri et al. (2019) and Wang et al. (2021) in a Zn/Cd-adenine MOF and in a Cu12 nanocage-based MOF, respectively. Parmar et al. (2019) showed that iodide can act similarly as bromide in the ring-opening step and mapped out the reaction pathway of CO2 cycloaddition onto an epoxide-bearing oxindole catalyzed by a Co-MOF and potassium iodide. Ma et al. (2022) designed a Cd-MOF with dynamic metal-iodide bonds that can release iodide anions to facilitate the ring-opening step, which was then confirmed by DFT calculations. Yang and Jiang. (2022) combined DFT calculations and wavefunction analysis to elucidate that the regioselectivity in CO2-PO cycloaddition co-catalyzed by Cu-MFU-4l/TBAB is a result of the competition between electronic and steric effects.
Without a bromide or iodide cocatalyst, efficient CO2 conversion to CC has also been achieved with MOF catalysts. Wu et al. (2019a), Wu et al. (2019b) designed a series of MOFs with format linkers and nitrogen-containing ligands which showed good catalytic performance. DFT calculations helped elucidate the role of those ligands in the reaction, which is to activate CO2 by increasing the nucleophilicity of its oxygen atom for easier nucleophilic attack on epoxide. Dhankhar et al. (2021) also observed in a Cu-MOF with nitrogen-rich linkers that the nitrogen-containing functional groups on the linker can activate CO2 to facilitate nucleophilic attack, with the help of DFT calculations.
In the aforementioned ten computational works, four density functionals have been used: M06 (Zhao and Truhlar, 2008), M06-2X (Zhao and Truhlar, 2008), M06-L (Zhao and Truhlar, 2006), and B3LYP. Vosko et al. (1980), Lee et al. (1988), Becke. (1993) M06, M06-2X, and B3LYP are among the most widely used functionals in computational chemistry due to their versatility and robustness, while M06-L has been extensively used for transition metals. Besides cluster-based DFT, periodic DFT has also been employed to investigate this catalytic reaction, which eliminates the procedure of carving out the active site cluster by including the entire periodic structure in the calculation (Table 1; Figure 2). However, due to the high computational cost associated with large unit cells of MOFs (often hundreds of atoms) and limited options on functionals (using hybrid functionals such as B3LYP often increase the computational cost significantly in periodic systems), the usage of periodic DFT in MOF catalysis is less common (albeit on the rise recently). The only reported mechanistic pathway mapped out by periodic DFT in MOF-catalyzed CO2-to-CC conversion to date is by Luo et al. (2019), who used the PBE (Perdew et al., 1996) functional with plane-wave basis sets to study this reaction catalyzed by a Zn-MOF.
To get the benefits of both cluster and periodic DFT, multi-level methods such as ONIOM (Chung et al., 2015) has been adopted (Figure 2). In ONIOM, the system is divided into multiple layers, each of which can be described using a different level of theory to balance accuracy and cost (Table 1). Xu et al. (2018a) constructed a two-layer ONIOM model to study the cycloaddition between CO2 and styrene oxide catalyzed by Co/Mg-MOF-74, in which an inner layer of 105 atoms around the active site were described using B3LYP while the rest of the system as an outer layer was described by molecular mechanics (MM). Li et al. (2019) adopted a similar strategy to study the cycloaddition between CO2 and propylene oxide catalyzed by a Cu-MOF NTU-180 and TBAB, in which M06-L was selected for the inner layer. Compared with cluster-based DFT, ONIOM can better capture the noncovalent interactions that the substrates “feel” in the MOF pores, which may be crucial in certain cases.
Besides mapping out mechanistic pathways, computational methods can help better understand this catalytic reaction in other ways. Zhang et al. (2016), Sharma et al. (2018) and Kong et al. (2021) carried out DFT calculations to locate the most favorable binding sites of CO2 in different MOFs as starting points of mechanistic investigations. Parmar et al. (2019) performed grand canonical Monte Carlo (GCMC) simulations with MM force fields to obtain the sorption isotherm and binding sites of CO2 in their Co-MOF, prior to DFT study on cycloaddition reaction pathway. Liao et al. (2020) combined GCMC and DFT to get both the spatial distribution and binding energy of CO2 in a Cu-MOF named HNUST-9, which exhibited high catalytic activity in CO2-to-CC conversion. Müller et al. (2019) performed GCMC simulations to screen 12 computationally-generated MOF structures made of the same linker but different inorganic nodes and topologies for CO2 adsorption. The one with highest CO2 uptake was then synthesized and exhibited high catalytic activity in CC production.
Another important category of CO2 conversion reactions is the reduction of CO2 into fuels such as HCOOH, CO, CH3OH and CH4 (Figure 1), also known as “artificial photosynthesis.” This process allows carbon to be reused and can help achieve a carbon neutral economy. Due to the high thermodynamic stability of CO2, photochemistry is often used to facilitate its reduction. Dhakshinamoorthy et al. (2016) These reactions involve complicated processes such as excitation/emission and electron transfer, which makes computational mechanistic investigations even more useful.
MOFs with photosensitive structural units such as porphyrin/Ru (bpy)3 (and analogs) in the organic linkers and redox-active metals in the inorganic nodes can be used as photocatalysts. For example, Wang et al. (2019a) performed a thorough computational study on CO2 photoreduction catalyzed by Co-porphyrin (CoP) and a series of 2D CoP-based MOFs (Co/Zn/Zr-CoPMOF) using periodic DFT calculations. The electronic properties of the MOFs were studied by calculating the partial density of states (PDOS), which confirmed that the 3d states of Co in CoP contribute dominantly to the valence bands (VB) near Fermi level in all those MOFs. Interestingly, the 3d states of Co in Co-oxo clusters in Co-CoPMOF also has significant contribution to the same VB region and formed a low-energy conduction band (CB), which was not observed in Zn/Zr-CoPMOF or CoP itself. This results in a much narrower band gap of Co-CoPMOF (1.2 eV) compared to others (1.6–1.7 eV) and a higher light absorption ratio in Co-CoPMOF (confirmed by absorption spectra calculations), which could lead to easier electron transfer and higher photocatalytic activity. Indeed, subsequent DFT calculations showed that the CO2 to CH4 photoreduction followed the same reaction pathway on all those catalysts and had the same rate-determining step, but Co-CoPMOF had the lowest energy barrier among them. Jin. (2020) conducted a combined experimental-computational study on CO2-to-formate photoreduction catalyzed by three Zr-MOFs (PCN-222/223/224) with Zn-porphyrin linkers and showed that the catalytic activity may be correlated with the CO2 binding energy on Zn-porphyrin. Yan et al. (2018) discovered high catalytic activity of CO2 to formate photoreduction in the Eu-Ru (phen)3-MOF and showed by DFT that a water molecule coordinated to Eu can be replaced by CO2 (possibly assisted by light irradiation) to facilitate following reactions. Elcheikh Mahmoud et al. (2019) utilized time-dependent DFT (TD-DFT) to calculate the absorption spectra of a Ru (cptpy)2-based Zr-MOF AUBM-4 and showed that its photocatalytic activity in CO2 reduction should come from the Ru-to-cptpy metal-to-ligand charge transfer (MLCT) rather than the Ru-to-Zr metal-to-metal charge transfer (MMCT). Gao et al. (2020) and Stanley et al. (2021) incorporated Ru (bpy)3 and its analogs into UiO-67 and MIL-101-NH2, respectively, for photocatalytic CO2 reduction and conducted DFT calculations to study the reaction mechanism. Sun et al. (2015) doped Ti into the Zr-node in UiO-66-NH2 and observed enhanced photocatalytic activity in CO2 reduction. DFT calculations and PDOS analysis revealed that the addition of Ti creates a low-energy CB and facilitates charge transfer. The reduced Ti (Ti3+) can further reduce Zr4+ to Zr3+ through MMCT which is catalytically active, therefore acting as a mediator in the charge transfer chain. Wang et al. (2018a), Han et al. (2018), and Wang et al. (2019b) used DFT calculations to show the importance of CO2-metal binding energy on the photocatalytic activity in Co/Ni-based MOFs and provided strategies to tune this binding energy via structural modification.
MOFs have been integrated with other materials to form composite photocatalysts for CO2 reduction, in which computational methods have also been employed for mechanistic investigation. Xu et al. (2018b) integrated BIF-20, a Zn-MOF with high density of exposed B-H bonds, with graphitic carbon nitride (g-C3N4) nanosheets. DFT calculations and electron density analysis demonstrated that upon receiving photoexcited electrons from g-C3N4, B-H groups can trap the electron and strengthen CO2 binding for subsequent reduction reaction. Jiang et al. (2020) grew photosensitive TiO2 clusters inside the pores of MIL-101 and conducted periodic DFT calculations to find out possible electron transfer pathways. Benseghir et al. (2020) used DFT calculations to obtain the spatial distribution of polyoxometalate (POM) clusters in UiO-67 and elucidated the origin of enhanced photocatalytic activity of this MOF-POM composite. Olowoyo et al. (2020) utilized a semi-empirical tight-binding method to determine the orbitals in Ti-based MIL-125-NH2 to understand its photocatalytic activity in CO2 reduction. Yu et al., (2021) used DFT to elucidate the catalytic mechanism of CO2 reduction in UiO-66-MoS2 composite, in which the bridging Mo-O-Zr motif facilitates charge transfer from MoS2 to Zr4+ to generate catalytically active Zr3+.
Electro catalysis is another solution to facilitate difficult redox reactions such as CO2 reduction (Figure 1). MOFs, despite having the aforementioned advantages as catalysts, have not been extensively used as electro catalysts until recently due to their often poor conductivity. Downes and Marinescu. (2017) With the discovery of more conductive MOFs as well as MOF-based composites, computational methods have been employed to study the mechanism of catalytic electroreduction of CO2.
Wang et al. (2018b) designed a POM-metalloporphyrin MOF in which the electron-rich low-valent metals (Mo(V)) in POMs and the macrocycle conjugated π-electron system in metalloporphyrin can boost the electrocatalytic activity together. Periodic DFT calculations showed that the energy barrier of CO2-to-CO reduction in this MOF is much lower than in POM or metalloporphyrin alone, supporting the hypothesis of synergistic involvement of POM and metalloporphyrin in electron mobility. Huang et al. (2020) used DFT calculations to show that coordinated halogen anions on the Cd metal sites in Cd-metalloporphyrin MOFs NNU-17/18 can help activate bound CO2 molecules for subsequent electroreduction. Zhong et al. (2020) developed 2D Cu-Zn bimetallic MOFs for CO2-to-CO electroreduction and revealed different roles of Cu and Zn centers in the catalysis using periodic DFT. Zhu et al. (2021) discovered from DFT calculations that a non-traditional C−H···O hydrogen bond played an important role in stabilizing the adsorbed *CHO intermediate during CO2 electroreduction catalyzed by the MOF Cu-MFU-4l. Majidi et al. (2021) observed high activity towards CO2 electroreduction at low overpotentials in a conductive 2D Cu-tetrahydroxyquinone MOF and used periodic DFT to map out the free energy profile. Zhao et al. (2021b) and Kang et al. (2020) combined Cu-based MOFs with Cu nanoparticles and Cu-foam electrodes, respectively, for CO2 electroreduction and conducted DFT calculations to map out the reaction pathways.
Despite many challenges, pure chemical (without photo/electro catalysis) reduction of CO2 in MOFs has still been made possible, which is mostly achieved by using H2 as the reductant to form HCOOH (Figure 1). This requires the heterolytic dissociation of H2 to a proton and a hydride, which can then attack the oxygen and carbon on CO2, respectively. Frustrated Lewis pairs (FLPs), in which active Lewis acid and Lewis base groups are separated by steric hinderance, can both bind CO2 (Ashley and O'Hare, 2013) and heterolytically dissociate H2 (Stephan and Erker, 2010). The porosity and tunability of MOFs make them excellent platforms for incorporating FLPs. Ye and Johnson (Ye and Johnson, 2015a; Ye and Johnson, 2015b; Ye and Johnson, 2016; Ye et al., 2018) have conducted a series of computational work on exploring MOF-based FLPs for CO2 hydrogenation. They first studied (Ye and Johnson, 2015a) the mechanism of CO2 hydrogenation catalyzed by boryl-pyrazole-based FLPs incorporated in UiO-66 using periodic DFT, in which they obtained a low energy barrier but also found that H2 needs to interact with FLP before CO2. Otherwise, the barrier increases significantly due to the strong CO2-FLP binding. They then conducted (Ye and Johnson, 2015b) a computational screening by varying the functional groups on FLP and observed a nearly linear correlation between H2 binding energy and CO2 hydrogenation barrier—stronger H2 binding leads to higher CO2 hydrogenation barrier. A Sabatier analysis was then carried out to determine the FLP with optimal trade-off between those two quantities. They also extended the scope of their work by studying further hydrogenation to CH3OH (Ye and Johnson, 2016) and exploring other MOFs (Ye et al., 2018). In the latter work (Ye et al., 2018), GCMC simulations were also performed to identify MOFs with high CO2 adsorption capacity. Yang and Jiang. (2020) created a FLP in UiO-66 by simply removing one bidentate carboxylate linker from the node and putting a hydroxide ligand on one of the two newly-created open Zr sites to balance the charge. DFT calculations confirmed that the other open Zr site and the hydroxide can act as (frustrated) Lewis acid and base, respectively, to catalyze CO2 hydrogenation. Heshmat. (2020) revisited the reaction investigated by Ye and Johnson (2015a) using periodic DFT-based ab initio molecular dynamics (AIMD) simulations with meta dynamics for enhanced sampling. A stepwise pathway was found to be more favorable in AIMD in contrast to the concerted pathway observed from static DFT, suggesting more mechanistic possibilities for these reactions.
Chemical hydrogenation of CO2 without FLP has also been achieved using other MOF-based catalysts, most with open metal sites. Maihom et al. (2013) conducted DFT calculations to show that Cu-alkoxide-functionalized MOF-5 can largely reduce the barrier in CO2-to-HCOOH hydrogenation. An et al. (2019) and Zhang et al. (2021) incorporated Cu/Zn on the nodes in Zr-MOFs and observed synergistic catalytic effect between Cu/Zn and Zr in CO2 hydrogenation. Qi et al. (2021) combined experiments and DFT calculations to show that in metalloporphyrin-based PCN-224, the Zr-node and the Cu-porphyrin catalyzed different segments of the CO2-to-ethanol reaction. Krishnan et al. (2022) computationally screened metalloporphyrin-based MOF-525 (similar to PCN-224) with different metal centers for CO2-to-HCOOH hydrogenation and identified Rh and Ir as best candidates. Yang and Jiang. (2021) computationally explored MOF-supported N-heterocyclic carbene-stabilized metal hydrides for catalytic CO2 hydrogenation to produce methanol. Gutterod et al. (2020a), Gutterod et al. (2020b), Zhu et al. (2020) and Mori et al. (2020) incorporated Pt/Cu/PdAg nanoparticles in MOFs for CO2 hydrogenation and conducted synergistic experimental-computational works to study the nanoparticle-MOF interactions and the catalytic mechanism.
In conclusion, computational chemistry has emerged as an essential tool in the study and design of MOF-based catalysts for CO2 conversion, particularly for providing atomistic-level insights and mapping out mechanistic pictures. DFT-based structural optimizations, energy calculations, transition state searches and wavefunction analyses have been routinely employed in the workflow. With the rapid development of new theoretical and computational methods, we believe that more state-of-the-art tools beyond regular DFT calculations will be applied to this problem in the near future, including but not limited to advanced wavefunction theory-based methods, machine learning (ML)-based potentials and AIMD. Nevertheless, rigorous testing and comprehensive benchmark calculations should be carried out to establish more robust and transferrable computational protocols. Moreover, with the recent development of robust high-throughput DFT/ML workflows for MOFs (Rosen et al., 2019; Rosen et al., 2021; Rosen et al., 2022a; Rosen et al., 2022b) and the establishment of large MOF structure databases (Wilmer et al., 2011; Chung et al., 2014; Colón et al., 2017; Moghadam et al., 2017; Chung et al., 2019; Li et al., 2021; Rosen et al., 2021; Rosen et al., 2022b), high-throughput computational screening can be adopted to exponentially accelerate the discovery of new MOF catalysts for CO2 conversion. We believe that computational chemistry study will become an integral part in the massively collaborative effort to solve the omnipresent global climate crisis via carbon capture and utilization.
HC contributed to the conceptualization, literature survey, and manuscript writing.
HC acknowledges the start-up funding from The University of Texas Rio Grande Valley and the Welch Foundation departmental Grant No. #BX-0048.
The author declares that the research was conducted in the absence of any commercial or financial relationships that could be construed as a potential conflict of interest.
All claims expressed in this article are solely those of the authors and do not necessarily represent those of their affiliated organizations, or those of the publisher, the editors and the reviewers. Any product that may be evaluated in this article, or claim that may be made by its manufacturer, is not guaranteed or endorsed by the publisher.
An, B., Li, Z., Song, Y., Zhang, J., Zeng, L., Wang, C., et al. (2019). Cooperative copper centres in a metal–organic framework for selective conversion of CO2 to ethanol. Nat. Catal. 2, 709–717. doi:10.1038/s41929-019-0308-5
Ashley, A. E., and O'Hare, D. (2013). FLP-mediated activations and reductions of CO2 and CO. Top. Curr. Chem. 334, 191–217. doi:10.1007/128_2012_377
Becke, A. D. (1993). Density-functional thermochemistry. III. The role of exact exchange. J. Chem. Phys. 98, 5648–5652. doi:10.1063/1.464913
Benseghir, Y., Lemarchand, A., Duguet, M., Mialane, P., Gomez-Mingot, M., Roch-Marchal, C., et al. (2020). Co-Immobilization of a Rh catalyst and a keggin polyoxometalate in the UiO-67 Zr-based metal–organic framework: In depth structural characterization and photocatalytic properties for CO2 reduction. J. Am. Chem. Soc. 142, 9428–9438. doi:10.1021/jacs.0c02425
Beyzavi, M. H., Klet, R. C., Tussupbayev, S., Borycz, J., Vermeulen, N. A., Cramer, C. J., et al. (2014). A hafnium-based metal–organic framework as an efficient and multifunctional catalyst for facile CO2 fixation and regioselective and enantioretentive epoxide activation. J. Am. Chem. Soc. 136, 15861–15864. doi:10.1021/ja508626n
Beyzavi, M. H., Stephenson, C. J., Liu, Y., Karagiaridi, O., Hupp, J. T., and Farha, O. K. (2015). Metal–Organic framework-based catalysts: Chemical fixation of CO2 with epoxides leading to cyclic organic carbonates. Front. Energy Res. 2, 63. doi:10.3389/fenrg.2014.00063
Chung, L. W., Sameera, W. M. C., Ramozzi, R., Page, A. J., Hatanaka, M., Petrova, G. P., et al. (2015). The ONIOM method and its applications. Chem. Rev. 115, 5678–5796. doi:10.1021/cr5004419
Chung, Y. G., Camp, J., Haranczyk, M., Sikora, B. J., Bury, W., Krungleviciute, V., et al. (2014). Computation-ready, experimental metal–organic frameworks: A tool to enable high-throughput screening of nanoporous crystals. Chem. Mat. 26, 6185–6192. doi:10.1021/cm502594j
Chung, Y. G., Haldoupis, E., Bucior, B. J., Haranczyk, M., Lee, S., Zhang, H., et al. (2019). Advances, updates, and analytics for the computation-ready, experimental metal–organic framework database: CoRE MOF 2019. J. Chem. Eng. Data 64, 5985–5998. doi:10.1021/acs.jced.9b00835
Colón, Y. J., Gómez-Gualdrón, D. A., and Snurr, R. Q. (2017). Topologically guided, automated construction of metal–organic frameworks and their evaluation for energy-related applications. Cryst. Growth Des. 17, 5801–5810. doi:10.1021/acs.cgd.7b00848
Dhakshinamoorthy, A., Asiri, A. M., and Garcia, H. (2016). Metal–organic framework (MOF) compounds: Photocatalysts for redox reactions and solar fuel production. Angew. Chem. Int. Ed. 55, 5414–5445. doi:10.1002/anie.201505581
Dhankhar, S. S., Das, R., Ugale, B., Pillai, R. S., and Nagaraja, C. M. (2021). Chemical fixation of CO2 under solvent and Co-Catalyst-free conditions using a highly porous two-fold interpenetrated Cu(II)-Metal–Organic framework. Cryst. Growth & Des. 21, 1233–1241. doi:10.1021/acs.cgd.0c01530
Downes, C. A., and Marinescu, S. C. (2017). Electrocatalytic metal–organic frameworks for energy applications. Chemsuschem 10, 4374–4392. doi:10.1002/cssc.201701420
Elcheikh Mahmoud, M., Audi, H., Assoud, A., Ghaddar, T. H., and Hmadeh, M. (2019). Metal–organic framework photocatalyst incorporating bis(4'-(4-carboxyphenyl)-terpyridine)ruthenium(II) for visible-light-driven carbon dioxide reduction. J. Am. Chem. Soc. 141, 7115–7121. doi:10.1021/jacs.9b01920
Gao, X., Guo, B., Guo, C., Meng, Q., Liang, J., and Liu, J. (2020). Zirconium-based metal–organic framework for efficient photocatalytic reduction of CO2 to CO: The influence of doped metal ions. ACS Appl. Mat. Interfaces 12, 24059–24065. doi:10.1021/acsami.0c05631
Gutterod, E. S., Lazzarini, A., Fjermestad, T., Kaur, G., Manzoli, M., Bordiga, S., et al. (2020). Hydrogenation of CO2 to methanol by Pt nanoparticles encapsulated in UiO-67: Deciphering the role of the metal–organic framework. J. Am. Chem. Soc. 142, 999–1009. doi:10.1021/jacs.9b10873
Gutterod, E. S., Pulumati, S. H., Kaur, G., Lazzarini, A., Solemsli, B. G., Gunnaes, A. E., et al. (2020). Influence of defects and H2O on the hydrogenation of CO2 to methanol over Pt nanoparticles in UiO-67 metal–organic framework. J. Am. Chem. Soc. 142, 17105–17118. doi:10.1021/jacs.0c07153
Han, B., Ou, X., Deng, Z., Song, Y., Tian, C., Deng, H., et al. (2018). Nickel metal-organic framework monolayers for photoreduction of diluted CO2 : Metal-Node-Dependent activity and selectivity. Angew. Chem. Int. Ed. Engl. 57, 17053–17057. doi:10.1002/ange.201811545
Heshmat, M. (2020). Alternative pathway of CO2 hydrogenation by lewis-pair-functionalized UiO-66 MOF revealed by metadynamics simulations. J. Phys. Chem. C 124, 10951–10960. doi:10.1021/acs.jpcc.0c01088
Hu, T.-D., Sun, Y.-W., and Ding, Y.-H. (2018). A quantum-chemical insight on chemical fixation carbon dioxide with epoxides co-catalyzed by MIL-101 and tetrabutylammonium bromide. J. CO2 Util. 28, 200–206. doi:10.1016/j.jcou.2018.09.027
Huang, C. H., and Tan, C. S. (2014). A Review: CO2 utilization. Aerosol Air Qual. Res. 14, 480–499. doi:10.4209/aaqr.2013.10.0326
Huang, Q., Niu, Q., Ma, N. N., Dong, L. Z., Li, S. L., Li, D. S., et al. (2020). Axial Cl/Br atom-mediated CO2 electroreduction performance in a stable porphyrin-based metal–organic framework. Chem. Commun. 56, 14817–14820. doi:10.1039/d0cc06092e
Jiang, Z., Xu, X., Ma, Y., Cho, H. S., Ding, D., Wang, C., et al. (2020). Filling metal-organic framework mesopores with TiO2 for CO2 photoreduction. Nature 586, 549–554. doi:10.1038/s41586-020-2738-2
Jin, J. (2020). Porphyrin-based metal–organic framework catalysts for photoreduction of CO2: Understanding the effect of node connectivity and linker metalation on activity. New J. Chem. 44, 15362–15368. doi:10.1039/d0nj03507f
Kang, X., Li, L., Sheveleva, A., Han, X., Li, J., Liu, L., et al. (2020). Electro-reduction of carbon dioxide at low over-potential at a metal–organic framework decorated cathode. Nat. Commun. 11, 5464. doi:10.1038/s41467-020-19236-4
Kathalikkattil, A. C., Babu, R., Roshan, R. K., Lee, H., Kim, H., Tharun, J., et al. (2015). An lcy-topology amino acid MOF as eco-friendly catalyst for cyclic carbonate synthesis from CO2: Structure-DFT corroborated study. J. Mat. Chem. A Mat. 3, 22636–22647. doi:10.1039/c5ta05688h
Kong, X. H., Hu, K. Q., Mei, L., Li, A., Liu, K., Zeng, L. W., et al. (2021). Double-layer nitrogen-rich two-dimensional anionic uranyl-organic framework for cation dye capture and catalytic fixation of carbon dioxide. Inorg. Chem. 60, 11485–11495. doi:10.1021/acs.inorgchem.1c01492
Krishnan, R., Yang, K., Hashem, K., and Jiang, J. (2022). Metallated porphyrinic metal−organic frameworks for CO2 conversion to HCOOH: A computational screening and mechanistic study. Mol. Catal. 527, 112407. doi:10.1016/j.mcat.2022.112407
Lee, C., Yang, W., and Parr, R. G. (1988). Development of the Colle-Salvetti correlation-energy formula into a functional of the electron density. Phys. Rev. B 37, 785–789. doi:10.1103/physrevb.37.785
Li, A., Perez, R. B., Wiggin, S., Ward, S. C., Wood, P. A., and Fairen-Jimenez, D. (2021). The launch of a freely accessible MOF CIF collection from the CSD. Matter 4, 1105–1106. doi:10.1016/j.matt.2021.03.006
Li, D., Kassymova, M., Cai, X., Zang, S.-Q., and Jiang, H.-L. (2020). Photocatalytic CO2 reduction over metal–organic framework-based materials. Coord. Chem. Rev. 412, 213262. doi:10.1016/j.ccr.2020.213262
Li, R., Zhang, W., and Zhou, K. (2018). Metal–organic-framework-based catalysts for photoreduction of CO2. Adv. Mat. 30, 1705512. doi:10.1002/adma.201705512
Li, X., Cheetham, A. K., and Jiang, J. (2019). CO2 cycloaddition with propylene oxide to form propylene carbonate on a copper metal–organic framework: A density functional theory study. Mol. Catal. 463, 37–44. doi:10.1016/j.mcat.2018.11.015
Liao, J., Zeng, W., Zheng, B., Cao, X., Wang, Z., Wang, G., et al. (2020). Highly efficient CO2 capture and conversion of a microporous acylamide functionalized rht-type metal–organic framework. Inorg. Chem. Front. 7, 1939–1948. doi:10.1039/d0qi00231c
Luo, Z., Wang, J., He, Y., Ao, Q., Deng, Q., Zeng, Z., et al. (2019). A stable Zn-based metal–organic framework as an efficient catalyst for carbon dioxide cycloaddition and alcoholysis at mild conditions. Catal. Lett. 150, 1408–1417. doi:10.1007/s10562-019-03053-6
Ma, R., Qiao, C., Xia, L., Xia, Z., Yang, Q., Xu, Y., et al. (2022). Dynamic metal-iodide bonds in a tetracoordinated cadmium-based metal–organic framework boosting efficient CO2 cycloaddition under solvent- and cocatalyst-free conditions. Inorg. Chem. 61, 7484–7496. doi:10.1021/acs.inorgchem.2c00569
Maihom, T., Wannakao, S., Boekfa, B., and Limtrakul, J. (2013). Production of formic acid via hydrogenation of CO2 over a copper-alkoxide-functionalized MOF: A mechanistic study. J. Phys. Chem. C 117, 17650–17658. doi:10.1021/jp405178p
Majidi, L., Ahmadiparidari, A., Shan, N., Misal, S. N., Kumar, K., Huang, Z., et al. (2021). 2D copper tetrahydroxyquinone conductive metal–organic framework for selective CO2 electrocatalysis at low overpotentials. Adv. Mat. 33, e2004393. doi:10.1002/adma.202004393
Moghadam, P. Z., Li, A., Wiggin, S. B., Tao, A., Maloney, A. G. P., Wood, P. A., et al. (2017). Development of a cambridge structural database subset: A collection of metal–organic frameworks for past, present, and future. Chem. Mat. 29, 2618–2625. doi:10.1021/acs.chemmater.7b00441
Mori, K., Konishi, A., and Yamashita, H. (2020). Interfacial engineering of PdAg/TiO2 with a metal–organic framework to promote the hydrogenation of CO2 to formic acid. J. Phys. Chem. C 124, 11499–11505. doi:10.1021/acs.jpcc.0c02457
Müller, P., Bucior, B., Tuci, G., Luconi, L., Getzschmann, J., Kaskel, S., et al. (2019). Computational screening, synthesis and testing of metal–organic frameworks with a bithiazole linker for carbon dioxide capture and its green conversion into cyclic carbonates. Mol. Syst. Des. Eng. 4, 1000–1013. doi:10.1039/c9me00062c
Olowoyo, J. O., Saini, U., Kumar, M., Valdés, H., Singh, H., Omorogie, M. O., et al. (2020). Reduced graphene oxide/NH2-MIL-125(Ti) composite: Selective CO2 photoreduction to methanol under visible light and computational insights into charge separation. J. CO2 Util. 42, 101300. doi:10.1016/j.jcou.2020.101300
Parmar, B., Patel, P., Pillai, R. S., Tak, R. K., Kureshy, R. I., Khan, N. H., et al. (2019). Cycloaddition of CO2 with an epoxide-bearing oxindole scaffold by a metal-organic framework-based heterogeneous catalyst under ambient conditions. Inorg. Chem. 58, 10084–10096. doi:10.1021/acs.inorgchem.9b01234
Perdew, J. P., Burke, K., and Ernzerhof, M. (1996). Generalized gradient approximation made simple. Phys. Rev. Lett. 77, 3865–3868. doi:10.1103/physrevlett.77.3865
Qi, S.-C., Yang, Z.-H., Zhu, R.-R., Lu, X.-J., Xue, D.-M., Liu, X.-Q., et al. (2021). The cascade catalysis of the porphyrinic zirconium metal–organic framework PCN-224-Cu for CO2 conversion to alcohols. J. Mat. Chem. A Mat. 9, 24510–24516. doi:10.1039/d1ta06950k
Rachuri, Y., Kurisingal, J. F., Chitumalla, R. K., Vuppala, S., Gu, Y., Jang, J., et al. (2019). Adenine-based Zn(II)/Cd(II) metal–organic frameworks as efficient heterogeneous catalysts for facile CO2 fixation into cyclic carbonates: A DFT-supported study of the reaction mechanism. Inorg. Chem. 58, 11389–11403. doi:10.1021/acs.inorgchem.9b00814
Rosen, A. S., Fung, V., Huck, P., O’Donnell, C. T., Horton, M. K., Truhlar, D. G., et al. (2022). High-throughput predictions of metal–organic framework electronic properties: Theoretical challenges, graph neural networks, and data exploration. npj Comput. Mat. 8, 112. doi:10.1038/s41524-022-00796-6
Rosen, A. S., Iyer, S. M., Ray, D., Yao, Z., Aspuru-Guzik, A., Gagliardi, L., et al. (2021). Machine learning the quantum-chemical properties of metal–organic frameworks for accelerated materials discovery. Matter 4, 1578–1597. doi:10.1016/j.matt.2021.02.015
Rosen, A. S., Notestein, J. M., and Snurr, R. Q. (2019). Identifying promising metal–organic frameworks for heterogeneous catalysis via high-throughput periodic density functional theory. J. Comput. Chem. 40, 1305–1318. doi:10.1002/jcc.25787
Rosen, A. S., Notestein, J. M., and Snurr, R. Q. (2022). Realizing the data-driven, computational discovery of metal–organic framework catalysts. Curr. Opin. Chem. Eng. 35, 100760. doi:10.1016/j.coche.2021.100760
Schaffner, B., Schaffner, F., Verevkin, S. P., and Borner, A. (2010). Organic carbonates as solvents in synthesis and catalysis. Chem. Rev. 110, 4554–4581. doi:10.1021/cr900393d
Sharma, N., Dhankhar, S. S., Kumar, S., Kumar, T. J. D., and Nagaraja, C. M. (2018). Rational design of a 3D Mn(II) -Metal–Organic framework based on a nonmetallated porphyrin linker for selective capture of CO2 and one-pot synthesis of styrene carbonates. Chem. Eur. J. 24, 16662–16669. doi:10.1002/chem.201803842
Song, J. L., Zhang, Z. F., Hu, S. Q., Wu, T. B., Jiang, T., and Han, B. X. (2009). MOF-5/n-Bu4NBr: An efficient catalyst system for the synthesis of cyclic carbonates from epoxides and CO2 under mild conditions. Green Chem. 11, 1031–1036. doi:10.1039/b902550b
Stanley, P. M., Thomas, C., Thyrhaug, E., Urstoeger, A., Schuster, M., Hauer, J., et al. (2021). Entrapped molecular photocatalyst and photosensitizer in metal–organic framework nanoreactors for enhanced solar CO2 reduction. ACS Catal. 11, 871–882. doi:10.1021/acscatal.0c04673
Stephan, D. W., and Erker, G. (2010). Frustrated Lewis pairs: Metal-free hydrogen activation and more. Angew. Chem. Int. Ed. 49, 46–76. doi:10.1002/anie.200903708
Sun, D., Liu, W., Qiu, M., Zhang, Y., and Li, Z. (2015). Introduction of a mediator for enhancing photocatalytic performance via post-synthetic metal exchange in metal–organic frameworks (MOFs). Chem. Commun. 51, 2056–2059. doi:10.1039/c4cc09407g
Vosko, S. H., Wilk, L., and Nusair, M. (1980). Accurate spin-dependent electron liquid correlation energies for local spin-density calculations - a critical analysis. Can. J. Phys. 58, 1200–1211. doi:10.1139/p80-159
Wang, C., Liu, X.-M., Zhang, M., Geng, Y., Zhao, L., Li, Y.-G., et al. (2019). Two-dimensional cobaltporphyrin-based cobalt–organic framework as an efficient photocatalyst for CO2 reduction reaction: A computational study. ACS Sustain. Chem. Eng. 7, 14102–14110. doi:10.1021/acssuschemeng.9b02699
Wang, W. M., Wang, W. T., Wang, M. Y., Gu, A. L., Hu, T. D., Zhang, Y. X., et al. (2021). A porous copper-organic framework assembled by [Cu12] nanocages: Highly efficient CO2 capture and chemical fixation and theoretical DFT calculations. Inorg. Chem. 60, 9122–9131. doi:10.1021/acs.inorgchem.1c01104
Wang, X.-K., Liu, J., Zhang, L., Dong, L.-Z., Li, S.-L., Kan, Y.-H., et al. (2019). Monometallic catalytic models hosted in stable metal–organic frameworks for tunable CO2 photoreduction. ACS Catal. 9, 1726–1732. doi:10.1021/acscatal.8b04887
Wang, Y., Huang, N. Y., Shen, J. Q., Liao, P. Q., Chen, X. M., and Zhang, J. P. (2018). Hydroxide ligands cooperate with catalytic centers in metal–organic frameworks for efficient photocatalytic CO2 reduction. J. Am. Chem. Soc. 140, 38–41. doi:10.1021/jacs.7b10107
Wang, Y. R., Huang, Q., He, C. T., Chen, Y., Liu, J., Shen, F. C., et al. (2018). Oriented electron transmission in polyoxometalate-metalloporphyrin organic framework for highly selective electroreduction of CO2. Nat. Commun. 9, 4466. doi:10.1038/s41467-018-06938-z
Wilmer, C. E., Leaf, M., Lee, C. Y., Farha, O. K., Hauser, B. G., Hupp, J. T., et al. (2011). Large-scale screening of hypothetical metal–organic frameworks. Nat. Chem. 4, 83–89. doi:10.1038/nchem.1192
Wu, Y., Song, X., Xu, S., Yu, T., Zhang, J., Qi, Q., et al. (2019). [(CH3)2NH2] [M(COOH)3] (M=Mn, Co, Ni, Zn) MOFs as highly efficient catalysts for chemical fixation of CO2 and DFT studies. Mol. Catal. 475, 110485. doi:10.1016/j.mcat.2019.110485
Wu, Y., Song, X., Zhang, J., Xu, S., Gao, L., Zhang, J., et al. (2019). Mn-based MOFs as efficient catalysts for catalytic conversion of carbon dioxide into cyclic carbonates and DFT studies. Chem. Eng. Sci. 201, 288–297. doi:10.1016/j.ces.2019.02.032
Xu, G., Zhang, H., Wei, J., Zhang, H. X., Wu, X., Li, Y., et al. (2018). Integrating the g-C3N4 nanosheet with B-H bonding decorated metal–organic framework for CO2 activation and photoreduction. ACS Nano 12, 5333–5340. doi:10.1021/acsnano.8b00110
Xu, K., Moeljadi, A. M. P., Mai, B. K., and Hirao, H. (2018). How does CO2 react with styrene oxide in Co-MOF-74 and Mg-MOF-74? Catalytic mechanisms proposed by QM/MM calculations. J. Phys. Chem. C 122, 503–514. doi:10.1021/acs.jpcc.7b09790
Yan, Z. H., Du, M. H., Liu, J., Jin, S., Wang, C., Zhuang, G. L., et al. (2018). Photo-generated dinuclear {Eu(II)}2 active sites for selective CO2 reduction in a photosensitizing metal–organic framework. Nat. Commun. 9, 3353. doi:10.1038/s41467-018-05659-7
Yang, K., and Jiang, J. (2020). Computational design of a metal-based frustrated Lewis pair on defective UiO-66 for CO2 hydrogenation to methanol. J. Mat. Chem. A Mat. 8, 22802–22815. doi:10.1039/d0ta07051c
Yang, K., and Jiang, J. (2022). Highly efficient CO2 conversion on a robust metal–organic framework Cu(I)-MFU-4l: Prediction and mechanistic understanding from DFT calculations. J. CO2 Util. 63, 102148. doi:10.1016/j.jcou.2022.102148
Yang, K., and Jiang, J. (2021). Transforming CO2 into methanol with N-heterocyclic carbene-stabilized coinage metal hydrides immobilized in a metal–organic framework UiO-68. ACS Appl. Mat. Interfaces 13, 58723–58736. doi:10.1021/acsami.1c18885
Ye, J., and Johnson, J. K. (2016). Catalytic hydrogenation of CO2 to methanol in a Lewis pair functionalized MOF. Catal. Sci. Technol. 6, 8392–8405. doi:10.1039/c6cy01245k
Ye, J., and Johnson, J. K. (2015). Design of Lewis pair-functionalized metal organic frameworks for CO2 hydrogenation. ACS Catal. 5, 2921–2928. doi:10.1021/acscatal.5b00396
Ye, J., and Johnson, J. K. (2015). Screening Lewis pair moieties for catalytic hydrogenation of CO2 in functionalized UiO-66. ACS Catal. 5, 6219–6229. doi:10.1021/acscatal.5b01191
Ye, J., Li, L., and Johnson, J. K. (2018). The effect of topology in Lewis pair functionalized metal organic frameworks on CO2 adsorption and hydrogenation. Catal. Sci. Technol. 8, 4609–4617. doi:10.1039/c8cy01018h
Yu, C. H., Huang, C. H., and Tan, C. S. (2012). A Review of CO2 capture by absorption and adsorption. Aerosol Air Qual. Res. 12, 745–769. doi:10.4209/aaqr.2012.05.0132
Yu, F., Jing, X., Wang, Y., Sun, M., and Duan, C. (2021). Hierarchically porous metal–organic framework/MoS2 interface for selective photocatalytic conversion of CO2 with H2 O into CH3 COOH. Angew. Chem. Int. Ed. Engl. 60, 25053–25057. doi:10.1002/ange.202108892
Zhang, G., Wei, G., Liu, Z., Oliver, S. R. J., and Fei, H. (2016). A robust sulfonate-based metal–organic framework with permanent porosity for efficient CO2 capture and conversion. Chem. Mat. 28, 6276–6281. doi:10.1021/acs.chemmater.6b02511
Zhang, J., An, B., Li, Z., Cao, Y., Dai, Y., Wang, W., et al. (2021). Neighboring Zn-Zr sites in a metal–organic framework for CO2 hydrogenation. J. Am. Chem. Soc. 143, 8829–8837. doi:10.1021/jacs.1c03283
Zhao, Y., and Truhlar, D. G. (2006). A new local density functional for main-group thermochemistry, transition metal bonding, thermochemical kinetics, and noncovalent interactions. J. Chem. Phys. 125, 194101. doi:10.1063/1.2370993
Zhao, Y., and Truhlar, D. G. (2008). The M06 suite of density functionals for main group thermochemistry, thermochemical kinetics, noncovalent interactions, excited states, and transition elements: Two new functionals and systematic testing of four M06-class functionals and 12 other functionals. Theor. Chem. Acc. 120, 215–241. doi:10.1007/s00214-007-0310-x
Zhao, Y., Zheng, L., Jiang, D., Xia, W., Xu, X., Yamauchi, Y., et al. (2021). Nanoengineering metal–organic framework-based materials for use in electrochemical CO2 reduction reactions. Small 17, 2006590. doi:10.1002/smll.202006590
Zhao, Z. H., Zheng, K., Huang, N. Y., Zhu, H. L., Huang, J. R., Liao, P. Q., et al. (2021). A Cu(111)@metal-organic framework as a tandem catalyst for highly selective CO2 electroreduction to C2H4. Chem. Commun. 57, 12764–12767. doi:10.1039/d1cc05376k
Zhong, H., Ghorbani-Asl, M., Ly, K. H., Zhang, J., Ge, J., Wang, M., et al. (2020). Publisher Correction: Synergistic electroreduction of carbon dioxide to carbon monoxide on bimetallic layered conjugated metal–organic frameworks. Nat. Commun. 11, 1721. doi:10.1038/s41467-020-15668-0
Zhu, H.-L., Huang, J.-R., Zhang, X.-W., Wang, C., Huang, N.-Y., Liao, P.-Q., et al. (2021). Highly efficient electroconversion of CO2 into CH4 by a metal–organic framework with trigonal pyramidal Cu(I)N3 active sites. ACS Catal. 11, 11786–11792. doi:10.1021/acscatal.1c02980
Keywords: computational chemistry, metal–organic framework (MOF), CO2 conversion, density functional theory (DFT), computational catalysis
Citation: Chen H (2022) Recent advances in computational study and design of MOF catalysts for CO2 conversion. Front. Energy Res. 10:1016406. doi: 10.3389/fenrg.2022.1016406
Received: 10 August 2022; Accepted: 14 October 2022;
Published: 28 October 2022.
Edited by:
Kui Tan, The University of Texas at Dallas, United StatesReviewed by:
Nicolaas Vermeulen, NuMat Technologies, United StatesCopyright © 2022 Chen. This is an open-access article distributed under the terms of the Creative Commons Attribution License (CC BY). The use, distribution or reproduction in other forums is permitted, provided the original author(s) and the copyright owner(s) are credited and that the original publication in this journal is cited, in accordance with accepted academic practice. No use, distribution or reproduction is permitted which does not comply with these terms.
*Correspondence: Haoyuan Chen, aGFveXVhbi5jaGVuQHV0cmd2LmVkdQ==
Disclaimer: All claims expressed in this article are solely those of the authors and do not necessarily represent those of their affiliated organizations, or those of the publisher, the editors and the reviewers. Any product that may be evaluated in this article or claim that may be made by its manufacturer is not guaranteed or endorsed by the publisher.
Research integrity at Frontiers
Learn more about the work of our research integrity team to safeguard the quality of each article we publish.