Corrigendum: The viability of implementing hydrogen in the commonwealth of Massachusetts
- 1Department of Mechanical Engineering, University of Massachusetts Lowell, Lowell, MA, United States
- 2Department of Economics, University of Massachusetts Lowell, Lowell, MA, United States
- 3Department of Chemistry, University of Massachusetts Lowell, Lowell, MA, United States
- 4Department of Chemical Engineering, University of Massachusetts Lowell, Lowell, MA, United States
- 5Merrimac Municipal Light Department, Lowell, MA, United States
In recent years, there has been an increased interest in hydrogen energy due to a desire to reduce greenhouse gas emissions by utilizing hydrogen for numerous applications. Some countries (e.g., Japan, Iceland, and parts of Europe) have made great strides in the advancement of hydrogen generation and utilization. However, in the United States, there remains significant reservation and public uncertainty on the use and integration of hydrogen into the energy ecosystem. Massachusetts, similar to many other states and small countries, faces technical, infrastructure, policy, safety, and acceptance challenges with regards to hydrogen production and utilization. A hydrogen economy has the potential to provide economic benefits, a reduction in greenhouse gas emissions, and sector coupling to provide a resilient energy grid. In this paper, the issues associated with integrating hydrogen into Massachusetts and other similar states or regions are studied to determine which hydrogen applications have the most potential, understand the technical and integration challenges, and identify how a hydrogen energy economy may be beneficial. Additionally, hydrogen’s safety concerns and possible contribution to greenhouse gas emissions are also reviewed. Ultimately, a set of eight recommendations is made to guide the Commonwealth’s consideration of hydrogen as a key component of its policies on carbon emissions and energy.
Introduction
Hydrogen (H2) is the highest energy content fuel by weight and is a building block for a wide variety of other materials (e.g., conventional and synthetic fuels, polymers, plastics, petroleum refining, fertilizer, etc.) used in manufacturing and industrial processing. The recent interest in hydrogen utilization has been motivated by several factors including: 1) the desire to reduce carbon dioxide (CO2) emissions due to the consumption of oil, propane, and natural gas for combustion; 2) the need for new climate-neutral sources of energy generation to meet ever growing human demands; 3) the significant reduction in the levelized cost of energy (LCOE) of renewable energy sources (i.e., wind and solar) that help facilitate the economic viability of green hydrogen production on a wide-scale; and 4) future opportunities to produce hydrogen at low-cost when an over-supply of renewable electricity leads to curtailments and negative power pricing. These factors provide an opportunity to economically use hydrogen for energy storage, transportable renewable energy, transportation, industrial processes, and material production.
This investigation has identified the opportunities and existing barriers to integrating hydrogen throughout the Commonwealth’s economy. It is important to mention that this research was conducted under sponsorship from the Associated Industries of Massachusetts (AIM) Foundation. The authors evaluated numerous peer reviewed publications, reports from national laboratories, and conducted interviews with a diversity of stakeholders. The opinions, findings, conclusions, or recommendations expressed in this report are those of the authors and do not reflect the views of the AIM Foundation or the stakeholders interviewed. Information regarding the stakeholders can be found in Supplementary Table S1 of the Supplementary Material. The primary topics that are considered in this paper include energy storage, thermal heating, industrial processes, transportation, safety, GHG emissions, pipeline transportation, synthetic fuels, biomass, and ammonia/fertilizer production. This paper serves as an important model for other states or countries to follow when they are considering the adoption of hydrogen into their energy portfolio
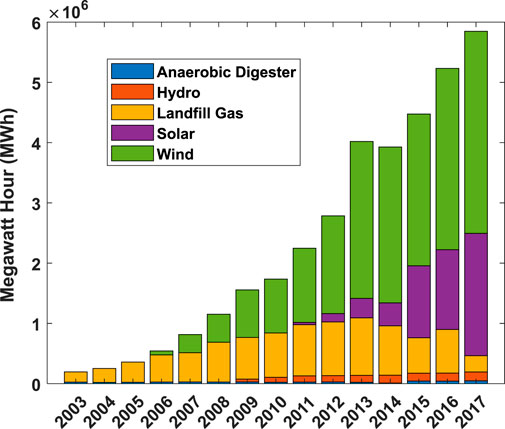
FIGURE 1. Massachusetts Renewable Energy Generation Portfolio, indicating that Massachusetts has a very large renewable energy resource from wind energy and that is expected to grow as a larger percentage in the next decade as offshore wind procurements are constructed (Massachusetts Department of Energy Resources: Renewable Energy Division, 2020).
If hydrogen is widely adopted by states and countries, it is important to distinguish the different methods of hydrogen production and the colors associated with each production method. This distinction is to ensure carbon dioxide and other greenhouse gases are not being emitted into the atmosphere when hydrogen is produced. The most widely used form of hydrogen to date is grey hydrogen, making up over 95% of the world’s hydrogen produced (Rapier, 2020). Grey hydrogen uses the process of steam methane reforming to produces a hydrogen and carbon oxide fuel gas mixture. The steam reforming process is carbon intensive and for every ton of hydrogen produced 9–12 tons of carbon dioxide are released into the atmosphere (Watson et al., 2021). Blue hydrogen is similar to grey hydrogen; however, it utilizes carbon capture utilization and storage (CCUS) to capture carbon emissions from the steam methane reforming process. Due to concerns over the efficiency of CCUS and how the system would be powered, blue hydrogen may not be viable at this time to meet carbon reduction goals. Pink hydrogen is generated through electrolysis of water by using electricity from a nuclear power plant, which help to reduce the curtailment of nuclear plants (Ajanovic et al., 2022). Green hydrogen is hydrogen generated through electrolysis of water powered by renewable energy sources (e.g., solar, wind, or hydro power). Electrolysis powered by renewables produces little to no carbon emissions. This study focuses on the benefits and applications associated with the use of green hydrogen because at this time green hydrogen is the only form of hydrogen that is viable for carbon emission reduction.
Opportunities for hydrogen utilization
A hydrogen-integrated economy relies on a diverse range of applications that utilize hydrogen. These applications include energy storage, thermal heating, industrial processes (e.g., manufacture of polymers, methanol), transportation, electricity production, synthesis of synthetic fuels, upgrading oil, and ammonia/fertilizer production. If successfully implemented, each application is likely to provide measurable benefits in meeting the carbon emission targets, including net-zero emissions by 2050, for Massachusetts (Lenton, 2021). However, successful implementation will need to overcome widespread adoption challenges, including safety concerns, to ensure the Commonwealth has a robust energy and economic infrastructure. Some of the relevant energy generation sectors and industrial hydrogen opportunities are effectively summarized in the well-known diagram created by Pivovar et al., (see Figure 2) in which the applications shown may or may not be relevant to a specific state or country (Pivovar et al., 2018). The different elements shown are now addressed specifically for Massachusetts but have direct relevance to other state, countries, and regions as well.
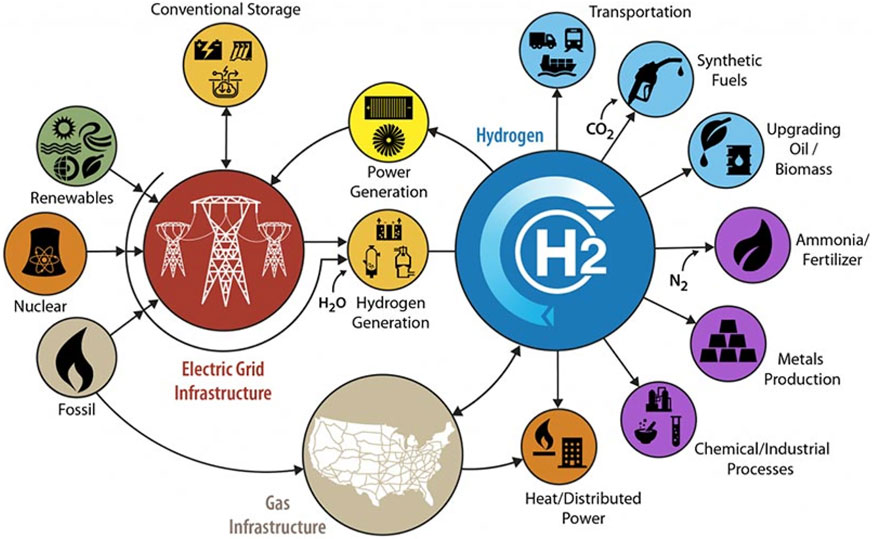
FIGURE 2. Schematic illustration of the H2@Scale concept showing the diverse range of H2 applications, such as energy storage, thermal heating, industrial processes, transportation, and electricity production (Pivovar et al., 2018).
Energy storage
With the planned installments and reliance on gigawatts of new renewable power capacity (e.g., offshore wind and solar), there will be opportunities to store excess energy instead of curtailing power generation systems and a need for energy storage to respond to peak demands associated with the intermittency of many renewable energy sources. For a large-scale reliance on renewable energy, energy storage must be integrated to balance and create a resilient electrical grid when either a lack or overabundance of renewable energy exists.
Presently, lithium-ion batteries have been introduced in some utility-scale storage systems. Although they are appropriate in providing a cost-effective short-duration energy storage solution (typically a few hours), when considered for long-duration energy storage, lithium-ion batteries are generally not cost-effective due to their relatively short lifespan. When these batteries are used for stationary energy storage and need to last several decades, their state of health will decrease nonlinearly (including capacity fade and increase in internal resistance) (Kendall and Ambrose, 2020; Zhu et al., 2021). Another drawback to solely relying on lithium-ion batteries is the limited global resource of lithium. Lithium is a critical material and is expected to be subject to supply shortages in the future, even considering extensive recycling operations. It is estimated that the earth has approximately 26 million tons of lithium reserves. Even with an optimistic higher assessment that assumes potential extractable mineral deposits, there is an estimated 51 million tons of lithium reserves. The current demand for lithium is 0.16 million tons per year and by 2030, the annual demand for new lithium is expected to be 2 million tons per year (Greim et al., 2020; BloombergNEF, 2021). According to the International Energy Agency, in order to achieve the Paris climate goals, by 2040 lithium will need to be consumed at a rate 42 times higher than current levels (Bader, 2021). To electrify vehicles, electronics, and leverage energy storage in the electrical grid with the best policy scenario and recycling efforts, the balance of lithium demand and supply could extend only to about 2050, and the market will then begin to experience a large deficit that lasts for the remaining half of the century (Greim et al., 2020). Therefore, solely relying on lithium-ion batteries for energy storage is generally seen as not a viable long-term option.
The use of hydrogen can be an effective method for storing large amounts of energy for long periods of time (e.g., days or weeks) either as a gas, liquid, or in the form of ammonia. When coupled with fuel cells or gas turbine engines, hydrogen energy storage systems can be used to provide a reliable backup energy source to address intermittency and ensure the energy grid is resilient to disruption (Arsad et al., 2022).
A preliminary techno-economic cost analysis (TEA) comparing energy storage using lithium-ion batteries (LIB) to a hydrogen storage/fuel cell system highlights the difference between different approaches. The energy storage capacity is rated at 15 MW for 3 days (72 h) in which the following assumptions were made: 1) the LIB cost is $176/kWh with 6 years of lifetime and a round-trip-efficiency (RTE) of 80%; 2) the volumetric and gravimetric energy density of LIB are 300 Wh/L and 175 Wh/kg; 3) the capital cost per kW is based on a recent TEA performed by NREL (presented at the third Annual HydroGEN Advanced Water Splitting Technology Pathways Benchmarking and Protocols Workshop by DOE Hydrogen and Fuel Cell Technologies Office; March 2021), in which the capital cost and RTE for a 80 MW hydrogen generation system is $93.3M ($1166/kW) at 60% efficiency for solid oxide electrolysis cells, $79M ($988/kW) at 49% efficiency for polymer electrolyte membrane electrolysis cells and $90M ($1125/kW) at 45% efficiency for alkaline electrolysis cells; 4) the volumetric and gravimetric energy density of the stack are 4.3 kW/L and 5.5 kW/kg; 5) hydrogen is stored in tanks at a pressure of 700 bar, at room temperature, and a cost of $8/kWh; 6) seawater is vaporized to form steam and the energy penalty has been included in the RTE reported in the bar chart. Based on this analysis, the results indicate that for long-duration energy storage, hydrogen is more viable in terms of weight (1/193 times), volume (1/2 times), lifetime (1.5 times), and capital cost (1/7 times) than lithium-ion batteries (see Figure 3).
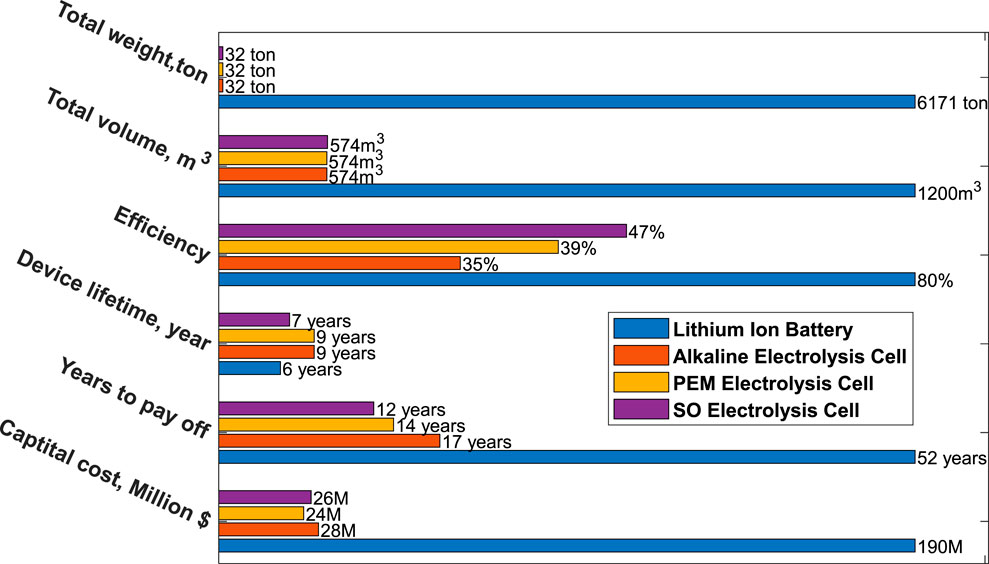
FIGURE 3. Technoeconomic analysis comparing lithium-ion battery energy storage to a proposed hydrogen storage approach for a 15 MW turbine and a 3-days energy storage solution. The results indicate that hydrogen is more viable in terms of weight (32 ton vs. 6171 ton), volume (574m3 vs. 1200 m3), lifetime (9 years vs. 6 years), and capital cost (190 M vs. 24 M) than lithium-ion batteries.
However, there are still challenges to an energy storage approach that includes hydrogen. Electrolyzer and fuel cell stack costs are still high due to limited production capability, small market share, and strict policy codes related to hydrogen generation and power-delivery devices. Furthermore, hydrogen storage and delivery capability with the existing infrastructure has not been demonstrated on a larger scale. If solutions to these challenges have been met, then hydrogen for energy storage will be able to meet cost targets and be cost competitive in the market. The overall near-term targets that have been set out by the DOE are $2/kg for hydrogen production and $2/kg for delivery and dispensing for transportation applications (Sunitha, 2021). Additional research needs to be performed in the following areas to decrease the cost and expand the hydrogen energy storage market: 1) technologies to reduce cost as well as to improve performance and reliability of fuel cell stacks and of storage and delivery methods; 2) harmonize codes and standards to address safety concerns; and 3) establish and safeguard a global supply chain and market, as well as workforce development.
Thermal heating
The thermal heating sector includes all home and commercial business, excluding agricultural and industrial activities. Implementing hydrogen into the thermal heating sector can provide opportunities to complement electrification by meeting energy demands during peak periods and periods of intermittent renewable energy production, thereby increasing resiliency. Currently, 52.3% of Massachusetts homeowners use a natural gas system for heating (U.S. Energy Information Administration, 2021) and to meet the Commonwealth’s zero-emission goals, most of these natural gas homeowners would need to switch to either an all-electric system (e.g., heat pumps and resistive heating), decarbonized gas, a network geothermal system, or apply some other possible new residential heating solutions. This switch will be costly and cause pushback by consumers, especially for the sector of the population who are economically disadvantaged. Gas companies would also need to either repurpose or abandon the existing pipeline infrastructure. However, if increasing percentages of natural gas can be displaced by hydrogen, end-users could potentially keep their existing appliances (with some modifications or retrofits depending on the blend fraction) while also enabling the state to meet its emission goals.
There are challenges with hydrogen implementation for the thermal heating sector that do need to be overcome in order to be commercially mature. A wholesale shift to change to a 100% hydrogen system would require a significant investment in infrastructure and technology. A useful analogy is to think about gasoline and Diesel fuel. A vehicle operator cannot just simply put gasoline in a Diesel engine, or put Diesel in a gasoline vehicle. While they are both “fuels”, their properties are different and so the hardware/technology must be designed appropriately to take advantage of the unique properties. The same can be said for hydrogen versus natural gas. While they are both fuels, they are not the same, and thus cannot be treated the same. However, much of the existing research on residential and industrial appliances has shown that low blend levels of hydrogen (i.e., less than 20%) can be tolerated without a significant change in performance. This indicates that even though hydrogen and natural gas have different combustion properties, achieving higher blend ratios should be achievable without dramatic technological interventions. Because hydrogen has a lower volumetric energy density than methane, volumetric blending of hydrogen with methane does not provide a linear reduction of carbon emissions per unit energy. For example, if methane is blended with hydrogen at 20%, 50%, or 75% by volume, the reduction in carbon emission per unit energy of the blended gas will be approximately 7%, 23%, and 47%, respectively (Goldmeer, 2019) (see Figure 4).
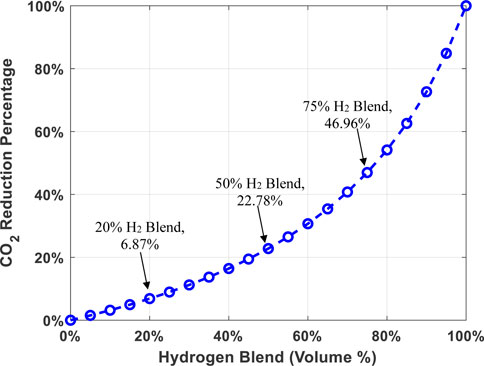
FIGURE 4. CO2 Reduction with respect to hydrogen and methane blend percentages, for example, if methane is blended with H2 at 20%, 50%, or 75% by volume, the carbon emission reductions per unit energy of the blended gas will be approximately 7%, 23%, and 47%, respectively.
Another potential challenge with using hydrogen in the thermal heating sector is hydrogen embrittlement of cast iron pipes and a lack of information and research done on how high blends and pure hydrogen in a natural gas system will affect the end-user’s appliances. Massachusetts has approximately 21,000 miles (33,796 km) of main pipelines used for the transportation of natural gas from meter stations throughout the distribution system (Pipeline Safety Information, 2021). The materials for main pipelines in Massachusetts vary depending on location and the distribution company and are made of either cast iron, steel, or a polyethylene plastic. There are approximately 7,928 miles (12,759 km) of steel pipelines, 11,016 miles (17,729 km) of plastic pipelines, and 2,809 miles (4,520 km) of cast iron pipelines.
Depending on the pipeline’s material, using hydrogen in either a pure form or a blend may cause embrittlement in pipelines. Polyethylene and lower-strength, thicker-wall steel pipelines are most compatible with hydrogen and have shown to be successful in large-scale pilot projects as well as with low blend ratios (Blanton et al., 2021). Other forms of steel pipelines are still being studied in national laboratories and individual companies. Cast iron (commonly found in distribution systems in Massachusetts and the Northeast) has been shown to be unsuitable for hydrogen (Blanton et al., 2021).
Once the blended hydrogen is delivered to the end-user appliances, functionality will vary depending on the blend ratio. Research done by HyDeploy has shown that a 20% blend by volume of hydrogen in a natural gas system has worked in city-wide pilot projects and residential appliances can function effectively up to a 28% blend of hydrogen without issue (HyDeploy, 2021). However, this project is the largest hydrogen blending project to date and little-to-no research has been done on higher blend ratios of hydrogen. To ensure the safety of the end-user and their appliances, more testing must be done to understand the effects of blending higher percentages of hydrogen in the natural gas network as well as the impact on residential appliances (i.e., stoves, furnaces, and hot water heaters) (Isaac, 2019).
For the hydrogen thermal heating sector to be successful, replacement of old and insufficient pipelines for hydrogen blending needs to be performed, more research needs to be done on higher blend ratios of hydrogen, and appliances may need to be redesigned or retrofitted to operate on hydrogen-natural gas blends or pure hydrogen. When a pipeline becomes old or damaged and needs to be replaced, it may be more cost-effective to replace the old pipeline with a hydrogen compatible pipeline made of low strength carbon steel or polyethylene if hydrogen will continue to be blended with natural gas.
Industrial processes
Hydrogen for industrial processes provides opportunities for decarbonizing industries when a large amount of heat is needed. One example is steel manufacturing that would otherwise be hard to completely electrify. Due to the processes used today to extract steel from iron ore, electricity cannot be used; instead, hydrogen is more viable as a replacement for coke (a derivative of coal) in the gasification processes used in industrial manufacturing. Using hydrogen in steel production only produces 0.056 tons of carbon dioxide for every ton of iron produced and represents 2.8% of the carbon emissions from steel production when coke is used (Vogl et al., 2018).
The primary challenges for industrial processing (requiring heat generation) to replace fossil fuels (coal, oil, natural gas) are the capital cost required to convert existing equipment as well as the cost of the fuel used in the manufacturing process. Currently, green hydrogen is more expensive for a given amount of energy compared to fossil fuels and there are no significant policies or incentives motivating companies to transition away from using fossil fuels. For example, carbon credits can be implemented to incentivize low or no carbon-emitting industrial processes and help make hydrogen fuel for industrial methods cost competitive (Vogl et al., 2018). At the federal level there is a bill that has been introduced (not passed), the “Clean H2 Production Act”, that would create production tax credits and investment tax credits for hydrogen (Sen and Thomas, 2021).
Transportation
The transportation sector generates the largest share of greenhouse gas emissions within Massachusetts and represents a sizable opportunity for hydrogen utilization through fuel cell electric powertrains and traditional internal combustion engines. The transportation sector is composed of different applications including passenger vehicles, trucks, ships, and airplanes. Opportunities that hydrogen can bring to the transportation sector include fast refueling compared to battery electric vehicles (BEVs), zero nitrogen oxides (NOx) emissions (if used in fuel cell vehicles), a longer-range driving alternative to BEVs, longer storage duration, and avoidance of CO2 emissions. Due to hydrogen’s high energy density, it allows for more energy to be stored per kilogram than other energy storage methods, including electric batteries (Muelaner, 2021).
The challenges in the transportation sector that hinder the adoption of hydrogen are the lack of infrastructure for refueling stations in Massachusetts and regulations that restrict the operation of hydrogen vehicles on some roadways (particularly tunnels). There are currently zero operating public hydrogen refueling stations and only two private hydrogen refueling stations in Massachusetts. When compared to electric charging stations, there is a drastic difference as significant expansion has been made in the last decade and there are 4,090 public and 299 private electric charging stations in Massachusetts (Alternative Fuels Data Center, 2021). Currently, hydrogen fuel purchases for new automobiles are subsidized by the auto manufacturers (e.g., Toyota Motor Corp.) by providing free hydrogen fill-ups, up to $15,000 for new automobile purchases (Verlin, 2022). The limited network of hydrogen fueling stations in Massachusetts hinders the driving range for hydrogen-powered vehicles, preventing market penetration and causing relatively high prices due to a lack of economy of scale.
A convincing body of evidence in both California and internationally has revealed that hydrogen-based vehicles can be operated safely with cost competitiveness compared to gasoline or other fuels. Of the 11,674 hydrogen-powered automobiles operating in California, there have been no significant issues with fires for vehicles involved in accidents (California Fuel Cell Partnership, 2021).
In cold weather environments, as in Massachusetts’ winters, the driving range for battery electric vehicles is reduced. Several studies have reported that the average driving range for battery electric vehicles decreases by 41% depending on the temperature and driving conditions (Delos Reyes et al., 2016; American Automobile Association, 2019; Olsen, 2019). A Norwegian study tested common battery electric vehicles and their driving range in cold climates and found that there was an average decrease of 18.5% in driving range and vehicles took between 27 and 60 min to achieve an 80% charge under rapid charging conditions (NAF, 2020). In contrast, a hydrogen automobile can be refueled in approximately 3 min and its driving range is not greatly affected by cold temperature operation.
For a mid-sized city with 100,000 parking spaces and an average cost of $1,200 per electric charger, the cost to electrify would be approximately $120 million dollars, not including the wiring infrastructure cost required for electrical transmission. It is not likely feasible for the vehicle transportation sector to be carbon neutral by relying solely on electric vehicles that utilize chemical batteries because of 1) the technical limitations of lithium-ion batteries operating in cold environments, 2) the inability for all drivers to have vehicles connected to charging stations at their homes throughout the night, and 3) a lack of suitability of using batteries for the long-range trucking, shipping, and aviation sectors. The path forward for increasing hydrogen in the transportation sector would be to increase the number of hydrogen fueling stations available to the public and address policies that hinder hydrogen transportation from further developing, such as restrictions for compressed hydrogen-powered vehicles traveling in tunnels in Massachusetts.
Safety
Hydrogen as a fuel source and form of energy storage has brought up concerns with the public whether hydrogen is safe to use. This misconception has been perpetuated ever since the Hindenburg disaster in 1937. Today the United States has 1,600 miles of existing hydrogen pipeline used in the Gulf Coast that has a track record of safety commensurate if not better than natural gas (Hydrogen and Fuel Cell Technologies Office, 2022). Every year hydrogen is safely transported through these hydrogen pipelines to be used in petroleum refineries and chemical plants.
Like many gasses, hydrogen is a colorless and odorless gas making it difficult to detect if a leak has occurred. Direct coloring agents may not be possible to add to hydrogen, but odorants can be added to provide a smell for hydrogen (HyDeploy). Sensors can also be installed to allow for fast and efficient detection of leaks without having to worry about seeing or smelling hydrogen. Other safety concerns regarding hydrogen include the wide ignition range of air concentrations from 4 to 74% (Carcassi and Fineschi, 2005) and the low energy ignition required (0.019 mJ) (Kumamoto et al., 2011) making hydrogen more likely to ignite in a wider range of scenarios than other combustible gases (e.g., natural gas). When stored in tanks or equipment, hydrogen is a safe fuel source and cannot be combusted unless there is a failure in the storage system. Safety codes and standards are put into place to minimize safety concerns and ensure the proper handling of hydrogen. Testing methods are also used to ensure the rigidity and verify the lifespan of these storage methods.
Testing has also been done on hydrogen igniting in enclosed spaces such as tunnels and it was found that no additional risk existed when compared to fuels like gasoline (LaFleur et al., 2017). For example, for a typical automobile, the energy available for combustion (∼13 gallons of gasoline) is approximately 3 times higher than for a hydrogen vehicle (∼4 k kg of hydrogen). If a hydrogen fuel leak were to occur resulting from a crash, the hydrogen would disperse upward rapidly as opposed to gasoline that wets the vehicle or pavement and does not disperse quickly in an accident.
Emissions of NOx is a safety concern with the combustion of hydrogen because it is a byproduct of the combustion process in air. Combustion of hydrogen blended with natural gas increases NOx emissions by 92.81% for a 25% blend and upwards of 360% for a 75% blend (Cellek and Pinarbasi, 2018). However, it is important to note that NOx emissions can be controlled and mitigated using certain techniques and modifications (e.g., by using a lean or lower fuel-to-air ratio). NOx is generated through combustion and the quantity of NOx is dependent on the flame temperature; by reducing the flame temperature, NOx emissions can be reduced (Menzies, 2019). Water injection can also be used to reduce the hydrogen flame temperature and thereby reduce NOx for combustion in air. Other approaches, such as catalytic converters, can be used in some appliances or furnaces to aid in the removal of NOx. European manufacturers have already started working on using these techniques and modifications and have found success in producing zero to low NOx emissions residential appliances (Sadler et al., 2017).
Greenhouse gas emissions
Gases that are responsible for trapping heat in the atmosphere are referred to as greenhouse gases (GHG). There are two primary concerns regarding the use of hydrogen and its effect on climate neutrality. The first is that N2O, commonly generalized as NOx, is generated during combustion of hydrogen and has a Global Warming Potential (GWP) 265–298 times that of CO2 for a 100-years timescale and represents about 7% of the total greenhouse gas emissions. For reference the GWP of methane is 28–36 over 100 years (United States Environmental Protection Agency, 2021). However, a majority of NOx emission in the United States comes from agriculture (75%) and only about 5% comes from stationary combustion (Menzies, 2019), which can be mitigated by the emission control strategies previously mentioned. It’s important to note that with a hydrogen-based system, carbon monoxide (CO) emissions will also be avoided. This is very important, as trade-offs are typically made in designing combustion systems for hydrocarbons, whereas trying to mitigate CO usually results in more NOx. But, if CO is not a concern, then there are multiple solutions that can be utilized to reduce NOx.
Another very important point is that NOx is a “catch-all” term that usually encompasses NO, NO2, and N2O when referencing combustion emissions. The majority of emissions during hydrogen combustion are NO and NO2, not N2O, which is the worst NOx in terms of GWP. The combustion of hydrogen will raise NOx emissions by 20–40% compared to methane. However, if one compares the NOx emissions during the stationary combustion of methane, one can see that the effect of N2O is insignificant. Greenhouse gas emissions are reported in units of carbon dioxide equivalent (CO2e) by multiplying by their GWP by their emission factors (United States Environmental Protection Agency, 2018). During the combustion of natural gas, the CO2e for CO2 is 53.06 kg CO2/mmBTU while the CO2e for N2O is 0.0298 kg N2O/mmBTU. This reveals that the resulting carbon dioxide emission has approximately 1780 times stronger effect on climate than the N2O gas emission for stationary combustion of natural gas. According to reference (Myron and Thompson, 2021), “Nitrous oxide (N2O) gas should not be confused with nitric oxide (NO) or nitrogen dioxide (NO2). Neither nitric oxide nor nitrogen dioxide are greenhouse gases, although they are important in the process of creation of tropospheric ozone (O3) which is a greenhouse gas.” The nitrogen oxides (NO + NO2) do not directly affect Earth’s radiative balance, but they accelerate the generation of a direct GHG—tropospheric ozone. However, the impact on climate is difficult to directly quantify (Dentener et al., 2001). Lastly, it is important to mention NOx is only generated in combustion processes when fuels (like hydrogen and natural gas) are burned in the presence of air. However, for applications that use a direct hydrogen fuel cell (e.g., in automobiles and electricity generation), the only byproducts are water, heat, and electrical energy with zero NOx emissions.
The second concern is that hydrogen itself (GWP of 5.8 over a 100-years timescale) is an indirect greenhouse gas that reacts in the atmosphere with tropospheric hydroxyl (OH) radicals and disrupts the distribution of methane in the ozone and thereby cause an increase in global warming. The release of hydrogen prolongs methane’s atmospheric residence time, increasing its accumulation and greenhouse gas impact (Derwent et al., 2006). According to one study by Derwent et al. (2018), if a global hydrogen economy replaced the current fossil fuel-based energy system and exhibited a leakage rate of 1% or 10%, then it would decrease the climate impact to 0.6% or 6% of the current fossil fuel based system, respectively. Another more recent literature review on the atmospheric impacts of hydrogen from heating found that the most likely outcome is that hydrogen has a greenhouse gas effect that is small but not zero, and the global atmospheric impacts are likely to be small (Derwent, 2018). Within the existing body of literature presented, there is significant uncertainty and additional research on this topic should be conducted. These findings emphasize the importance to ensure that leaks in hydrogen production, transportation, and utilization are minimized.
Pipeline transportation
Pipelines are the most cost-effective way to transport hydrogen compared to truck or rail. Although technical challenges remain on blending hydrogen with methane, solutions are being studied to identify how to increase the blending ratio while using the existing pipeline infrastructure (Melaina et al., 2013). Hydrogen has approximately one-third the heat value per unit volume compared to methane and so for the same pressure level, higher volumes of hydrogen need to be transported to deliver an equivalent amount of useable energy. This would require higher compression horsepower and will result in some additional energy losses compared to methane. The metering equipment used in gas distribution pipelines would also likely need modification based on the blend ratios (Blanton et al., 2021).
Gas emissions via leaks in pipelines and other distribution equipment are also important when assessing the GHG emissions of the carrier fuel, whether it is methane or hydrogen (Abel, 2021). Leaks are emitted via permeation through the pipe wall or through joints, fittings, and threads. For steel and ductile iron pipes, leakage mainly occurs through threads or mechanical joints and the volume leakage rate for hydrogen is about a factor of three higher than that for natural gas. For plastic pipes, permeation accounts for the majority of gas losses and are estimated to be about 4–5 times faster than for methane (United States Environmental Protection Agency, 2021). However, the leak rate depends on the blend percentage, pressure, and other factors. For example, in one study of a Dutch pipeline system, the experimentally estimated gas leakage rate was 0.0005% with a 17% hydrogen blend and considered to be insignificant (Haines et al., 2003). Because hydrogen is a smaller molecule than methane, hydrogen was thought to permeate through plastic pipelines more readily than methane, however, recent research has shown those leak rates are similar. Additionally, an application of an epoxy to thinly coat the steel pipe has been shown to successfully prevent hydrogen embrittlement, and threaded pipe fittings to prevent hydrogen leaks (Mejia et al., 2020; Lei et al., 2022). Another study calculated that the yearly loss of hydrogen by leakage through polyethylene pipelines amount to approximately 0.0005–0.001 percent of the total transported volume (Klopffer et al., 2015; Wassenaar and Micic, 2020).
One of the recommendations of a study performed by the Columbia University—Center on Global Energy Policy was to change the regulations on methane leak detection and repair the existing pipeline to be as low emission as possible, as well as accelerate the pace of cast-iron pipeline replacement (Blanton et al., 2021). These recommendations and others within their study are applicable to the Commonwealth of Massachusetts.
Synthetic fuels
Synthetic fuels are hydrocarbon fuels that are produced by chemically combining hydrogen with carbon sources such as CO2 or biomass. Synthetic fuels can be created to emulate common fuels such as gasoline, diesel, methane, and kerosene. The opportunities of using synthetic fuels over regular fuels is the use of CO2 (e.g., from atmospheric sequestration) in the manufacturing process and its compatibility with existing distribution systems, fueling stations, and conversion technologies without significant modifications to existing infrastructure or equipment. By using CO2 to produce synthetic fuels, it prevents additional CO2 emissions in the atmosphere and helps in meeting net-zero emissions goals. For example, renewable or synthetic natural gas can be created by combining waste CO2 from anaerobic digesters or power plants in MA with green hydrogen in a process referred to as methanation (Tsiotsias et al., 2020). Synthetic fuels can also be used in already existing refueling stations and combustion engines, which allow for a cost-effective transition to this carbon-neutral fuel.
Massachusetts currently lacks the existing infrastructure dedicated to producing synthetic fuels and the green hydrogen necessary to make these fuels carbon neutral. The processing facilities to produce synthetic fuels are currently expensive and there are only a few test plants in operation. Massachusetts currently has no test plants for synthetic fuels or a large-scale infrastructure of green hydrogen to produce synthetic fuels. Massachusetts is currently not a leader in the production of conventional fossil fuels. However, in the future, with an established large offshore wind resource, the low cost generated electricity could potentially position the Commonwealth to be an early mover or leader in production of economically viable synthetic fuels.
The path forward for Massachusetts to produce synthetic fuels would need to include more research to be done on the production of synthetic fuels as well as the development of a synthetic fuel infrastructure and market. Once more testing facilities have shown the benefits and challenges of synthetic fuels, then Massachusetts will be able to better assess if a synthetic fuel infrastructure would be beneficial for the Massachusetts economy. Before an economically viable carbon-neutral synthetic fuel infrastructure is developed, a large-scale green hydrogen facility would first need to be created.
Biomass, bio-oil, and bio-gas
Biomass, including bio-oil and bio-gas, can be used in steam reforming and water-gas shift processes to produce hydrogen. The opportunity with using biomass as a feedstock for hydrogen production is that biomass waste products are an available resource and can be used to sequester carbon dioxide from the atmosphere. It is estimated that up to 1 billion dry tons of sustainable biomass is available for energy generation use annually, which amounts to approximately 13–14 quadrillion Btu/year (in 2030) (Hydrogen and Fuel Cell Technologies Office, 2021). Biomass can also lead to an offset in carbon dioxide emissions because of the consumption of carbon dioxide in the production process of biomass.
Currently, there are no biomass production sites in Massachusetts that are used for hydrogen generation and therefore is not currently part of the Massachusetts economy. More research needs to be performed on the carbon offset and economic benefits for the Commonwealth. The challenges with reforming biomass include the cost of biomass-derived liquid, capital cost, and carbon emissions. Biomass-derived liquids are composed of larger molecules with more carbon atoms than natural gas and this makes them more difficult to separate and reform in the steam reforming process. Steam reforming processes for biomass have a high capital equipment cost as well as operation and maintenance cost. There are processes other than steam reforming that can produce hydrogen through biomass such as pyrolysis, but they are more costly and should be further researched and investigated before implementation.
Ammonia/fertilizer
Hydrogen can be produced and stored in the form of ammonia (NH3). The opportunity with ammonia for hydrogen storage is that it does constitutes a practical, low-cost storage alternative, not requiring high pressure or cryogenic temperatures. Ammonia can be liquified at a pressure of 10 bar and contained at a temperature of −33°C. When compared to liquid hydrogen, liquefaction requires pressures of about 100 bars and containment at temperatures of −253°C or lower. This significant decrease in pressure and temperature allows for a less energy-intensive method to store and transport hydrogen. Ammonia is an inhibitor for hydrogen embrittlement, meaning that ammonia can be safely transported through existing iron and steel natural gas pipelines.
The challenge with ammonia is the carbon-intensive processes currently used for its production. Today the common production of ammonia requires both the generation of hydrogen through steam methane reforming and nitrogen through air separation. Hydrogen and nitrogen are used as inputs to form ammonia in a catalyzed process at high temperature and pressure (i.e., the Haber-Bosch process). The use of green hydrogen in ammonia production is not currently economical. There still needs to be development in enhanced ammonia production before ammonia can be used at a large scale for green hydrogen storage or as an energy carrier. Currently, there are no ammonia or fertilizer production sites in Massachusetts and therefore it is not part of the state’s economy. More research needs to be performed on the economic benefits of manufacturing ammonia or fertilizer and their carbon impact on the Commonwealth.
Hydrogen integration
Further integration of green hydrogen into the Massachusetts economy enables a diversification of energy sources, supports market competition, affords greater energy resiliency, enables sector coupling, and minimizes changes of existing infrastructure to meet zero-emission goals. By diversifying Massachusetts’ fuel sources, hydrogen integration allows for the promotion of consumer choice, market competitiveness, and enhanced grid reliability. Consumers will be able to choose a low carbon energy source that best fits their needs and what may be more suitable in their area or for their socioeconomic status. Sector coupling with hydrogen energy allows for an increased integration of energy end-use and multiple supply sectors (He et al., 2021; Travers, 2021). This allows for an increased efficiency and flexibility of a hydrogen economy, as well as working with electrification to reduce the cost of decarbonization (Van Nuffel, 2018). For maximum carbon reduction, only green hydrogen should be considered as a future potential fuel source as opposed to other forms (grey and blue hydrogen) that require the utilization of fossil fuels in their production or because carbon sequestration technologies are not presently effective for net-zero large-scale production. In the future, new technologies may make the production/sequestration of blue or pink hydrogen have a lower carbon footprint and more economically viable. Blending methane with green hydrogen may be considered as a transitional fuel until sufficient electrification infrastructure exists, appropriate pipeline replacements are completed, the cost of green hydrogen is reduced, and the distribution infrastructure can accommodate 100% green hydrogen or carbon neutral synthetic fuels. The Massachusetts electrification efforts for commercial and residential heating and cooling (i.e., heat pumps) should initially be implemented in locations that currently rely solely on the dirtier fossil fuels (e.g., coal or oil) and do not have access to the natural gas infrastructure. Direct use of renewable electricity for heat and power should be a first consideration, when possible and economical, rather than using renewable energy to generate fuel or for storage because of round trip efficiency losses. Complete electrification may be difficult or impossible due to several factors such as intermittency, physical constraints, retrofitting limitations, transmission line augmentation, infrastructure replacement, permitting, public acceptance, and cost. Massachusetts’ climate 2030 goals include electrifying 100,000 homes per year, but in 2020 only 461 homes made the switch revealing an extreme shortfall in electrification progress for a variety of reasons (Shankman, 2021). In the end, to achieve widespread electrification and hydrogen production and distribution, the technology that will be embraced by consumers will be driven by cost per unit energy, performance, ease of implementation, capital expenditures required for retrofit and new energy infrastructure, and policy.
Challenges that a hydrogen economy will face includes producing green hydrogen at a cost competitive rate, incorporating the necessary infrastructure for safe utilization, addressing public acceptance, as well as adopting policies and creating incentives that enable hydrogen integration and consumption. Other countries and states (e.g., United Kingdom and NY) have already begun to explore the potential role of green hydrogen as part of a comprehensive decarbonization strategy (gov, 2021). In order to achieve carbon neutrality in Massachusetts, research and advancements need to be made in green hydrogen technology and further integration should be embraced. New policies and programs need to support the de-risking of large-scale commercial projects and pilot studies for technology and safety validation as well as public acceptance. These may include state or federal tax credits and other subsidies, loan guarantee programs, and research funding. New carbon neutral energy standards and infrastructure (e.g., fueling stations) will generate demand, help reduce costs, and increase energy resiliency, enabling widespread use of hydrogen for the commercial, residential and transportation industries relevant to Massachusetts.
The Commonwealth of Massachusetts, the United States, and the world are reaching an inflection point to address climate change and immediate action is necessary to transition to a world that does not rely on fossil fuels as its main energy source. Accomplishing this goal, in the short time necessary to make a difference, will require the planning and deployment of differing options, some of which are in initial or intermediate levels of development, but are anticipated to be realized in the near future or still face challenges with public perception and acceptance. For example, the vast renewable energy resource from offshore wind is expected to be available, but currently does not exist. Diversity, flexibility, and forward thinking will be necessary to make sure the Commonwealth’s energy supply is resilient to disruption. Some of these technologies may be useful in more than one sector, others may not be. For practical reasons, there is no one size fits all approach that will transition all sectors quickly and efficiently. All options need to be evaluated and considered, and it is particularly important to continue research into those technologies that are still in their nascent stage. The use of hydrogen, in some applications that currently use fossil fuels, will reduce overall greenhouse gas emissions, and help contribute to meeting the Commonwealth’s 2050 net-zero carbon goals, and if widely adopted, help reduce CO2 emissions globally. Challenges related to the use of hydrogen (e.g., cost, safety, public perception) can be overcome with proper and appropriate technological advancements, public awareness, and regulations.
Conclusions and recommendations for Massachusetts
To make the use of green hydrogen a reality, the Commonwealth should consider the following:
1. The development of an overall hydrogen policy that integrates the use of hydrogen to reduce the intensity of or eliminate the carbon of the fuels used in the thermal sector in Massachusetts.
2. A continuation of studies regarding the advantages of green hydrogen within the transportation system (passenger, medium and heavy-duty vehicles, marine, rail, and aviation sectors) that would enable a cost-effective market and reduction in carbon footprint.
3. A re-evaluation of the policies in place that hinder hydrogen transportation from further development, such as traveling restrictions for compressed hydrogen-powered vehicles.
4. Continued research into the use of long-duration energy storage using hydrogen in partnership with the offshore wind industry and other renewable energy sources available to Massachusetts.
5. The establishment of an optional pilot program implemented in participating gas local distribution companies’ (LDCs) distribution systems for a blended mix of hydrogen with natural gas to reduce the amount of carbon for thermal delivery.
6. The alignment of the existing Gas System Enhancement Program (GSEP) (Pipeline Safety Division, 2014) with the net-zero reduction goals of the Commonwealth to make sure the pipeline system is as low emission as possible and ready for use when the expected green hydrogen resource becomes available to address the thermal needs. The GSEP should also incorporate hydrogen compatible design standards.
7. The creation of a renewable procurement standard for natural gas utilities and suppliers similar to the electric renewable portfolio standard (RPS) programs, allowing green hydrogen that is produced with surplus renewable energy to qualify for “thermal renewable energy credits” (TRECs) that will encourage its use to further reduce the carbon footprint in the Commonwealth of Massachusetts.
8. Electrification efforts should initially be implemented in locations that currently rely solely on the fossil fuels (e.g., coal or oil) and do not have access to the natural gas infrastructure. Direct use of renewable electricity for heat and power should be a first consideration, when possible and economical, rather than using renewable energy to generate fuel or for storage because of round trip efficiency losses.
Author contributions
All authors listed have made a substantial, direct, and intellectual contribution to the work and approved it for publication.
Funding
The research in this study was conducted under sponsorship from the Associated Industries of Massachusetts (AIM) Foundation (Award# Agmt03012021). Any opinions, findings, conclusions or recommendations expressed in this report are those of the authors and do not reflect the views of the AIM Foundation or the stakeholders interviewed.
Conflict of interest
The authors declare that the research was conducted in the absence of any commercial or financial relationships that could be construed as a potential conflict of interest.
Publisher’s note
All claims expressed in this article are solely those of the authors and do not necessarily represent those of their affiliated organizations, or those of the publisher, the editors and the reviewers. Any product that may be evaluated in this article, or claim that may be made by its manufacturer, is not guaranteed or endorsed by the publisher.
Supplementary material
The Supplementary Material for this article can be found online at: https://www.frontiersin.org/articles/10.3389/fenrg.2022.1005101/full#supplementary-material
Abbreviations
BEV, Battery Electric Vehicles; CO, Carbon Monoxide; CO2, Carbon Dioxide; CO2E, Carbon Dioxide Equivalent; GHG, Greenhouse Gas; GSEP, Gas System Enhancement Program; GWP, Global Warming Potential; LCOE, Levelized Cost of Energy; LDCs, Local Distribution Companies’; MA, Massachusetts; N2O, Nitrous Oxide; NH3, Ammonia; NO, Nitric Oxide; NO2, Nitrogen Dioxide; NOx, Nitrogen Oxides; O3, Tropospheric Ozone; OH, Tropospheric Hydroxyl, RPS; Renewable Portfolio Standard; TREC, Thermal Renewable Energy Credit.
References
United States Environmental Protection Agency. “Emission factors for greenhouse gas inventories”, 2018 (Accessed November 1, 2021) Available at: https://www.epa.gov/sites/default/files/2018-03/documents/emission-factors_mar_2018_0.pdf
Abel, D. (2021). Massachusetts vastly underestimates emissions from natural gas, study finds”. Boston, MA: Boston Globe. Available at: https://www.bostonglobe.com/2021/10/25/science/state-vastly-underestimates-emissions-natural-gas-study-finds/ (Accessed November 1, 2021).
Ajanovic, A., Sayer, M., and Haas, R. (2022). The economics and the environmental benignity of different colors of hydrogen. Int. J. Hydrogen Energy 47 (57), 24136–24154. ISSN 0360-3199. doi:10.1016/j.ijhydene.2022.02.09424136-24154
Alternative Fuels Data Center. 2021. Massachusetts transportation Data for alternative fuels and vehicles”. Washington, DC: U.S. Department of Energy. Available at: https://afdc.energy.gov/states/ma (Accessed Sept. 27, 2021).
American Automobile Association (2019). AAA electric vehicle range testing” AAA news room. Florida United States: AAA.
Arsad, A. Z., Hannan, M. A., Al-Shetwi, A. Q., Mansur, M., Muttagi, K. M., Dong, Z. Y., et al. (2022). Hydrogen energy storage integrated hybrid renewable energy systems: A review analysis for future research directions. Int. J. Hydrogen Energy 47 (39), 1728517285–1731217312. ISSN 0360-3199. doi:10.1016/j.ijhydene.2022.03.208
Bader, J. (2021). The Big hurdle to jump-starting solar, wind energy and electric cars”. Atlanta, GA: CNN. Available at: https://www.cnn.com/2021/10/12/opinions/climate-change-clean-energy-bader/index.html (Accessed October 13, 2021).
Blanton, E. M., Lott, M. C., and Smith, K. N. (2021). Investing in the US natural gas pipeline system to support net-zero targets”. Columbia: Columbia SIPA Center on Global Energy Policy.
BloombergNEF. 2021. New energy outlook 2021”. Available at: https://about.bnef.com/new-energy-outlook/ (Accessed June 26, 2021).
California Fuel Cell Partnership. 2021. FCEV sales, FCEB, & hydrogen station Data”. West Sacramento, CA: California Fuel Cell Partnership. Available at: https://cafcp.org/by_the_numbers (Accessed Oct 8, 2021).
Carcassi, M., and Fineschi, F. (2005). Deflagrations of H2-air and CH4-air lean mixtures in a vented multi-compartment environment. Energy 30, 1439–1451. doi:10.1016/j.energy.2004.02.012
Cellek, M. S., and Pinarbasi, A. (2018). Investigations on performance and emission characteristics of an industrial low swirl burner while burning natural gas, methane, hydrogen-enriched natural gas and hydrogen as fuels. Int. J. Hydrogen Energy 11, 1194–1207. doi:10.1016/j.ijhydene.2017.05.107
Delos Reyes, J., Parsons, R., and Hoemsen, R. (2016). “Winter happens: The effect of ambient temperature on the travel range of electric vehicles,” in Proceeding IEEE Transaction on Vehiclular Technology, 18 March 2016 (IEEE),65 4016–4022. doi:10.1109/TVT.2016.2544178
Dentener, F., Derwent, R., Dlugokencky, E., Holland, E., Isaksen, I., Katima, J., et al. (2001). Atmospheric chemistry and greenhouse gases”. Geneva Switzerland: IPCC.
Derwent, R. 2018. Hydrogen for heating: Atmospheric impacts. London: Department for Business, Energy & Industrial Strategy. BEIS Research Paper Number 2018: no. 21, 2018.
Derwent, R., Simmonds, P., O’Doherty, S. J., Manning, A., Collins, W., and Stevenson, D. (2006). Global environmental impacts of the hydrogen economy. Int. J. Nucl. Hydrogen Prod. Appl. 1 (1), 57–67. doi:10.1504/IJNHPA.2006.009869
gov, N. Y. (2021). Governor cuomo announces New York will explore potential role of green hydrogen as part of comprehensive decarbonization strategy. NYSERDA. Available at: https://www.nyserda.ny.gov/About/Newsroom/2021-Announcements/2021-07-08-Governor-Cuomo-Announces-New-York-Will-Explore-Potential-Role-of-Green-Hydrogen (Accessed November 6, 2021).
Greim, P., Solomon, A., and Breyer, C. (2020). Assessment of lithium criticality in the global energy transition and addressing policy gaps in transportation. Nat. Commun. 11, 4570. doi:10.1038/s41467-020-18402-y
Haines, M. R., Polman, W., and de Laat, J. C. (2003). Reduction of CO2 emissions by addition of hydrogen to natural gas. Vancouver, Canada: Presented at the 2004 Greenhouse Gas Control Technologies conference.
He, G, Mallapragada, D S., Bose, A, Heuberger-Austin, C. F, and Gençer, E (2021). Sector coupling via hydrogen to lower the cost of energy system decarbonization. Energy Environ. Sci. 14 (9), 4635–4646. doi:10.1039/d1ee00627d
HyDeploy (2021). Demonstrating non-distrputive carbon savings through hydrogen blending. Keele, UK: Cadent, Keele University, Northern Gas Networks, ITM Power, Progressive Energy, HSE.
HyDeploy. (2022)“Hydrogen is invisible and does not smell. How will we know if there is a leak?”, (Accessed Oct, 8, 2021) Available at: https://hydeploy.co.uk/faqs/hydrogen-invisible-not-smell-will-know-leak/.
Hydrogen and Fuel Cell Technologies Office (2022). Hydrogen Pipelines Office Energy Effic. Renew. Energy. Available at: https://www.energy.gov/eere/fuelcells/hydrogen-pipelines (Accessed April 27, 2022).
Hydrogen and Fuel Cell Technologies Office. 2021. Hydrogen production: Biomass-derived liquid reforming”. Washington, DC: Office of Energy Efficiency & Renewable Energy. Available at: https://www.energy.gov/eere/fuelcells/hydrogen-production-biomass-derived-liquid-reforming (Accessed Sept. 28, 2021).
Isaac, T. (2019). HyDeploy: The UK’s first hydrogen blending deployment project. Clean. Energy 3, 114–125. doi:10.1093/ce/zkz006
Kendall, A., and Ambrose, H. (2020). Battery reuse: A second-life for electric vehicle batteries”. Davis, CA: NGI.
Klopffer, M., Berne, P., and Espuche, E. (2015). Development of innovating materials for distributing mixtures of hydrogen and natural gas. Study of the barrier properties and durability of polymer pipes. Oil Gas. Sci. Technol. –. Rev. IFP. Energies Nouv. 70, 305–315. doi:10.2516/ogst/2014008
Kumamoto, A., Iseki, H., Ono, R., and Oda, T. (2011). “Measurement of minimum ignition energy in hydrogen-oxygen-nitrogen premixed gas by spark discharge,” in Proceeding Internal Conference on Electrostatics, 301, 012039. doi:10.1088/1742-6596/301/1/012039J. Phys. Conf. Ser.
LaFleur, C., Bran-Anleu, G., Muna, A., Ehrhart, B., Blaylock, M., and Houf, W. “Hydrogen fuel cell electric vehicle tunnel safety study”. SANDIA REPORT, Technical Report OSTI.GOV.2017
Lei, Y., Hosseini, E., Liu, L., Scholes, C. A., and Kentish, S. E. (2022). Internal polymeric coating materials for preventing pipeline hydrogen embrittlement and a theoretical model of hydrogen diffusion through coated steel. Int. J. Hydrogen Energy. ISSN 0360-3199. doi:10.1016/j.ijhydene.2022.07.034
Lenton, C. (2021). Massachusetts setting net zero emissions goal by 2050. Nat. Gas. Intell. 29, 2021.
Massachusetts Department of Energy Resources: Renewable Energy Division. 2020, Renewable Energy Snapshot: Amount of Solar, wind and combined heat and power (CHP) installed in Massachusetts. Mass. Gov. Available at: https://www.mass.gov/info-details/renewable-energy-snapshot#:∼:text=Massachusetts%20has%20a%20long%20history, MW%20of%20Wind%20by%202020 (Accessed April 27, 2022).
Mejia, A., Brouwer, J., and Kinnon, M. (2020). Hydrogen leaks at the same rate as natural gas in typical low-pressure gas infrastructure. Int. J. Hydrogen Energy 45 (15), 8810–8826. doi:10.1016/j.ijhydene.2019.12.159
Melaina, M. W., Antonia, O., and Penev, M. (2013). Blending hydrogen into natural gas pipeline networks: A review of key issues”. Colorado Springs: NREL: National Renewable Energy Laboratory.
Menzies, M. (2019). Hydrogen: The burning question. Chem. Eng. Available at: https://www.thechemicalengineer.com/features/hydrogen-the-burning-question/(Accessed October 22, 2021).
Muelaner, J. (2021). Comparing EV battery and fuel cell energy density. Battery Power Tips. Available at: https://www.batterypowertips.com/comparing-ev-battery-and-fuel-cell-energy-density-faq/(Accessed April 27, 2022).
Myron, B., and Thompson, A. 2021“Chapter 2 – C3. Greenhouse gases: Nitrous oxide (N2O)” (Accessed November 1, 2021) Available at: http://www.soest.hawaii.edu/mguidry/Unnamed_Site_2/Chapter%202/Chapter2C3.html#
NAF, “20 popular Evs tested in Norwegian winter conditions” Veihjelp, 2020, (Accessed October 21, 2021) Available at: https://www.naf.no/elbil/aktuelt/elbiltest/ev-winter-range-test-2020/
Olsen, P. (2019). Buying an electric car for a cold climate double down on range. Consum. Rep. Available at: https://www.consumerreports.org/hybrids-evs/buying-an-electric-car-for-a-cold-climate-double-down-on-range/(Accessed October 21, 2021).
Pipeline Safety Division 2014. GSEPs pursuant to 2014 gas leaks Act. Boston, MA. Mass.gov. Available at: https://www.mass.gov/lists/gseps-pursuant-to-2014-gas-leaks-act (Accessed April 28, 2022).
Pipeline Safety Information Natural gas distribution”. Mass. Gov. Available at: https://www.mass.gov/info-details/natural-gas-distribution (Accessed October 10, 2021).
Pivovar, B., Rustagi, N., and Satyapal, S. (2018). Hydrogen at scale key to a clean, economic, and sustainable energy system, Electrochem. Soc. Interface, Spring, 27. Electrochemical Society Interface. Number 1.
Rapier, R. (2020). Estimating the carbon footprint of hydrogen production”. Jersey City, NJ. Forbes. Available at: https://www.forbes.com/sites/rrapier/2020/06/06/estimating-the-carbon-footprint-of-hydrogen-production/?sh=7ea375c424bd (Accessed April 28, 2021).
Sadler, D., Cargill, A., Crowther, M., Rennie, A., Watt, J., Burton, S., et al. (2017). H21 lead city gates project report” leads city gate. Leeds, UK: IGEM.
Shankman, S. (2021). Massachusetts should be converting 100,000 homes a year to electric heat. Boston, MA: Boston Globe. The actual number: 461”Available at: https://www.bostonglobe.com/2021/08/21/science/massachusetts-should-be-converting-100000-homes-year-electric-heat-actual-number-461/#:∼:text=According%20to%20the%20state's%20own,data%20reviewed%20by%20the%20Globe (Accessed November 1, 2021).
Sunitha, S. (2021) .Hydrogen and fuel cell technologies office director sunita satyapal sec world hydrogen summit conference. Washington D.C: Energy Efficiency and Renewable Energy.
Travers, K. (2021). Coupling power and hydrogen sector pathways to benefit decarbonization. MIT News. Available at: https://news.mit.edu/2021/coupling-power-hydrogen-sector-pathways-benefit-decarbonization-1021 (Accessed November 1, 2021).
Tsiotsias, A., Charisiou, N., Yentekakis, I., and Goula, M. (2020). Capture and methanation of CO2 using dual-function materials (DFMs). Chem. Proc. Novemb. 9. doi:10.3390/ECCS2020-07567
United States Environmental Protection Agency.2021 “Greenhouse gas emissions: Understanding global warming potentials”. Accessed November 1, 2021 Available at: https://www.epa.gov/ghgemissions/understanding-global-warming-potentials
U.S. Energy Information Administration. “Massachusetts state energy profile”2021 (Accessed Sept. 27, 2021), Available at: https://www.eia.gov/state/print. Php?sid=MA
Van Nuffel, L. (2018). Sector Coupling: How can it be enhanced in the EU to foster grid stability and decarbonize?”. Brussels Belgium: ITRE committee.
Verlin, K. (2022). Toyota mirai comes with $15, 000 of free hydrogen. NewsWheel, November 29, 2021. Available at: https://thenewswheel.com/2022-toyota-mirai-comes-with-15000-of-free-hydrogen/ (Accessed April 27, 2022).
Vogl, V., Âhman, M., and Nilsson, L. (2018). Assessment of hydrogen direct reduction for fossil-free steelmaking. J. Clean. Prod. 203, 736–745. doi:10.1016/j.jclepro.2018.08.279
Watson, G., Judd, V., and Franicevic “Grey, J. (2021). Green, Pink and Blue: We can sing a hydrogen rainbow too. Environ. J. Available at: https://environmentjournal.online/articles/grey-green-pink-and-blue-we-can-sing-a-hydrogen-rainbow-to/.
Keywords: hydrogen energy, hydrogen economy, hydrogen infrastructure, energy storage, thermal heating, Massachusetts
Citation: Hammerstrom B, Niezrecki C, Hellman K, Jin X, Ross MB, Mack JH, Agar E, Trelles JP, Liu F, Che F, Ryan D, Narasimhadevara MS and Usovicz M (2022) The viability of implementing hydrogen in the Commonwealth of Massachusetts. Front. Energy Res. 10:1005101. doi: 10.3389/fenrg.2022.1005101
Received: 27 July 2022; Accepted: 26 August 2022;
Published: 19 September 2022.
Edited by:
Daniel Zanetti De Florio, Federal University of ABC, BrazilReviewed by:
Tom Autrey, Pacific Northwest National Laboratory (DOE), United StatesPrasad Lakkaraju, Georgian Court University, United States
Copyright © 2022 Hammerstrom, Niezrecki, Hellman, Jin, Ross, Mack, Agar, Trelles, Liu, Che, Ryan, Narasimhadevara and Usovicz. This is an open-access article distributed under the terms of the Creative Commons Attribution License (CC BY). The use, distribution or reproduction in other forums is permitted, provided the original author(s) and the copyright owner(s) are credited and that the original publication in this journal is cited, in accordance with accepted academic practice. No use, distribution or reproduction is permitted which does not comply with these terms.
*Correspondence: Xinfang Jin, WGluZmFuZ19KaW5AdW1sLmVkdQ==