- 1Joint Center for Energy Storage Research, Lemont, IL, United States
- 2Chemical Sciences and Engineering Division, Argonne National Laboratory, Lemont, IL, United States
Rechargeable multivalent ion batteries are promising tools to complement current lithium-ion batteries for a future of diverse energy storage needs. Divalent Mg and Ca are attractive candidates for their high crustal abundance, high volumetric anode capacity, and infrequent dendrite formation during electrochemical cycling. Electrolyte research is central to these efforts and continually improves coulombic efficiencies towards the ideal 100%. This mini-review discusses recent work towards fundamental understandings that push these chemistries towards practical use. Piecing together compatible cathode and electrolytes for a complete practical multivalent ion battery lacks a cohesive method for further development and refinement. Understanding liquid solvation, utilizing rational design, and probing interfacial interactions are focal points that govern electrolyte performance. The combination of these areas will be critical for meaningful development.
Introduction: Beyond Lithium
As the anthropogenic effects of growing populations and global warming increasingly loom before us, the need for zero-emissions energy storage and delivery is the sine qua non of our technologically crowded lives. Of the energy produced in 2019, renewable sources account for only 11 percent, while natural gas (32%) and petroleum (37%) makeup the majority. Among residential, commercial, and industrial use, transportation leads the group with 28% of the total energy consumed and was almost exclusively driven by petroleum (Lawrence Livermore National Laboratory, 2020). Energy transfer efficiencies of up to 80% of battery power can be converted to movement at the wheels as opposed to 20–30% in internal combustion engines for cars. (Thomas, 2014; Carlson et al., 2016). Technology for renewable energy sources continues to lower in cost and has become a real competitor to conventional fossil-fueled energy production. A significant issue facing renewable energy technologies is the asynchronous moments of peak production and consumption. As a result, the introduction of large-scale energy storage, such as lithium-ion battery grid storage, has taken place in areas like Hornsdale, South Australia, and continued research efforts prioritize energy-dense storage technologies (Denholm et al., 2013).
Obstacles intrinsic to commercial LIB technology include the inability to use a metallic anode as the propensity of lithium to form dendrites can contribute to battery short-circuits or separator material rupture (Jäckle and Groß, 2014). Short circuits or exposure of internal components to the atmosphere can cause gaseous buildup or uncontrollable fires. With LIB technology projected to plateau and the high cost of scaling up, recent efforts have increased towards multivalent metal battery technology (Thackeray et al., 2012; Arroyo-De Dompablo et al., 2019; Ma et al., 2020; Wu et al., 2021).
Metals that form multivalent ions for use in batteries have garnered research popularity for their combination of earth-abundance, multivalent charge, and safety advantages. With relatively high theoretical volumetric energy densities (Mg: 3,832 mAh/cm3, Ca: 2072 mAh/cm3, as opposed to Li: 2061 mAh/cm3, or graphite: 777 mAh/cm3), low redox potentials (Mg: −2.36 V, Ca: −2.86 V vs. SHE), and similar ionic radii provide an attractive alternative to lithium/sodium intercalation technology (Aurbach et al., 2000; Aurbach et al., 2003; Fichtner, 2020).
These metals form dendrites less readily and are safer to handle in moist open air (Jäckle and Groß, 2014; Davidson et al., 2019; Ponrouch et al., 2019). Additionally, DV ion technologies use reversible electrodeposition chemistry with physically dense metallic anodes, unlike the graphite electrode used in LIBs. A solid metal anode allows for more active electron carriers to be contained in a smaller package, making multivalent ion battery technology attractive for transportation applications (El Kharbachi et al., 2020). Unfortunately, the current state of DV ion batteries lacks compatible components for commercial use.
Recent Work in Divalent Ion Electrolytes
Electrolytes facilitate movement of ions that shuttle between electrodes during charge and discharge. LIB electrolyte technology has not carried over despite generally sharing salt and solvent components with multivalent systems. Electrochemical plating, stripping, and intercalation behavior have proven to behave differently with divalent (DV) ion electrolytes due to more significant coulombic interactions and the complexity at interfaces(Tian et al., 2020).
Regarding non-aqueous divalent electrolytes, magnesium and calcium in particular, this text will serve as a short reminder of recent work and changes in research progression moving the field forward. The gap towards practical DV batteries reveals the need to focus on multidisciplinary research with a more encompassing approach involving all components of a battery. Research themes incorporating electrolyte-electrode relationships will be discussed further, including recent thrusts particularly driven by hubs like the Joint Center of Energy Storage Research (JCESR, 2021) and the European Magnesium Interactive Battery Community (E-magic, 2021).
The bases for electrochemistry later used in multivalent rechargeable batteries found its roots in the early 20th century as Mg deposits were observed in the electrolysis of Grignard solutions (Gaddum and French, 1927). While Mg electrolytes hold the largest variety, the majority of divalent salts fall within three major categories (i.e., halide-based anions, N-containing anions, and weakly coordinating anions), resulting in pros and cons for each type (Figure 1).
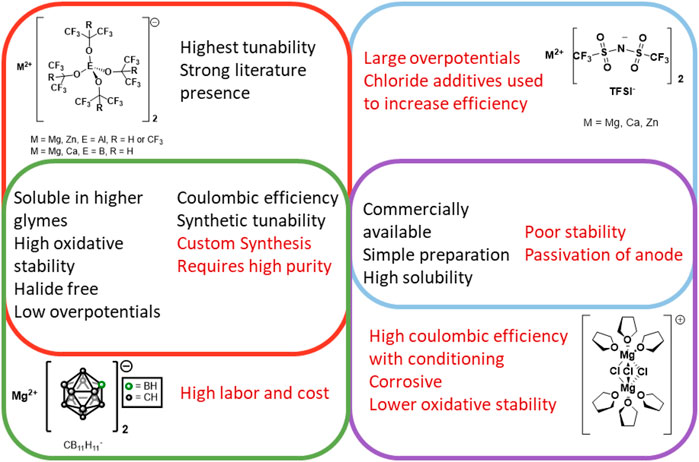
FIGURE 1. An overview of four select types of MV electrolytes and the unique and shared pros (in black) and cons (in red) associated with them.
Halide-based Mg-Ion Electrolytes
The bulk of early advancements in magnesium electrolytes involved halide-based salts, first investigated through Grignard solutions in the 1990s (Gregory et al., 1990). The pioneering concept of including a Lewis acid, like AlCl3, promoting MgCl+ formation for deposition, resulted in various analogs touting reversible plating efficiencies above 90% and approaching 100% (Mizrahi et al., 2008; Pour et al., 2011; Doe et al., 2014; Liu et al., 2014). The simple acid-base combination propelled a niche area showcasing MgCl2, the Lewis base, as a key design element for optimizing halide-based electrolytes (Pan et al., 2015; Pan et al., 2016a; Pan et al., 2016b; Pan et al., 2017). The most notable example, magnesium aluminum chloride complex (MACC), displays stability up to 3.1 V vs. Mg. These systems revealed a cationic dimer structure, [Mg2 (μ-Cl)3 (THF)6]+, that acts as the electroactive plating species. This system suffers from the necessity for electrochemical conditioning of the fresh electrolyte to provide Mg deposition with optimal overpotentials and efficiencies (Barile et al., 2014). However, these halide-based systems have shown compatibility issues with cathode candidates like transition metal oxides, sulfides, and borides (Kim et al., 2011; Verrelli et al., 2018; El Kharbachi et al., 2020; Park et al., 2020). The main drawback for these Grignard-based and other halide-containing electrolytes is the poor oxidative stability and corrosivity towards stainless steel battery parts, making it unrealistic in practical use.
N-Containing Anion Electrolytes
Cheap, simple, and halide-free electrolytes are attractive for larger-scale applications. As such, imide and amide-based electrolytes incorporating commercially available hexamethyldisilazane (HMDS-) or bis(trifluorosulfonimide) (TFSI-) (and the N-free triflate fragment) have become well-studied candidates for MV electrolytes. Examples developed so far perform relatively well with coulombic efficiencies approaching 100% for Mg and <30% for Ca (with BF3 additive at high temperature) (Forero-Saboya et al., 2021). Like most candidates mentioned so far, many simple commercially available salts provide room for coordination and contact ion pairing (CIP) with multivalent cations. These systems are penalized either through lost energy during dissociation and intercalation events or by enhanced decomposition as CIPs. It is important to note that HMDS electrolytes cannot operate without MgCl2, and TFSI electrolytes are often plagued by larger overpotentials. (Han et al., 2016; Lipson et al., 2016). To combat these disadvantages, the addition of MgCl2 to relatively weakly coordinating anion electrolytes tends to improve performance through formation of complex [MgxCly]+ species that reduce cation-anion association (Shterenberg et al., 2015; Gao et al., 2017; Shterenberg et al., 2017).
Weakly Coordinating Anion Electrolytes
Weakly coordinating anions (WCA) are employed for their ability to delocalize a negative charge over an entire robust structure, thus mitigating ion-pairing. The most common WCA systems utilize carboranes, four-coordinate borates, or aluminates (Carter et al., 2014; Herb et al., 2016; Zhao-Karger et al., 2017). To overcome the poor anodic stability of early Mg(BH4)2-based electrolytes (1.7 V vs. Mg) (Mohtadi et al., 2012), a modified salt, [Mg2 (μ-Cl)3 (THF)6][MgCl(C2B10H11)2] utilizing carbaborates demonstrated oxidative stability of 3.2 V vs. Mg. The chloride-free Mg(CB11H11)2 and ArMg(CB11H12), improved oxidative stability (≥3.8 V vs. Mg) (Carter et al., 2014; Tutusaus et al., 2015; Jay et al., 2019). Ca(CB11H12)2 was later adapted as one of few electrolytes to show room temperature reversible deposition of calcium (Kisu et al., 2021). Unfortunately, these unique carborane-type electrolytes present practical use issues as they can be some of the least cost-effective options and their syntheses cumbersome.
Building on early magnesium borohydride and organoborate electrolytes, recent examples tend to focus on alkoxyborate and aluminate species. The formation of E–O bonds (where E = Al: 512 kJ/mol, B: 806 kJ/mol) instead of E-C (Al: 225 kJ/mol, B 448 kJ/mol) is favorable in forming stable anions. The combination of bond strength and commercially available starting materials make aluminates and borates synthetically accessible (Riddlestone et al., 2018). A strong and growing literature presence of tetracoordinate anions has developed, known for stability, tunability, and steric bulk (Krossing et al., 2001; Krossing and Reisinger, 2005). Alkoxyaluminates constructed with hexafluoroisopropanol (hfip) are stable to 4 V vs. Mg, and trifluoromethylation of the tertiary C (sp3)-H extends electrochemical windows by 1 V (Herb et al., 2016; Lau et al., 2019). The borate analogs have found similar popularity for relatively wide electrochemical windows and use in calcium systems, further diversifying the viable electrolyte pool (Li et al., 2019; Shyamsunder et al., 2019). Alkoxyaluminates and borates are the most promising electrolytes as they provide versatility and synthetic tunability with practical examples of novel cathode compatibility. One caveat of this group of electrolytes is many examples experience loss of performance over extended cycling compared to carborane-type salts.
Recent Prospects and Future Directions
Progress has continually moved electrolytes towards practicality with wider electrochemical stability windows and higher coulombic efficiency. Electrolytes must function properly in a full cell, meaning cycling efficiencies need to approach 100% in practice. Early issues facing MV electrolytes (i.e., corrosion and oxidative stability) have become well studied, but electrolytes that plate and strip efficiently provide no use if their incompatibilities with a cathode system are not understood. The success of divalent batteries depends on a holistic approach to new or improved intercalation mechanisms, the prospect of an adjustable interface, and the availability of both efficient electrolytes and high voltage cathodes. We encourage the reader to explore recent accounts in these areas (Liang et al., 2020; Ma et al., 2020). With that said, future viewpoints do look promising considering recent themes as case studies in the following emerging themes: tools for optimizing solvation characteristics, rational electrolyte design, and understanding interfacial properties (Figure 2).
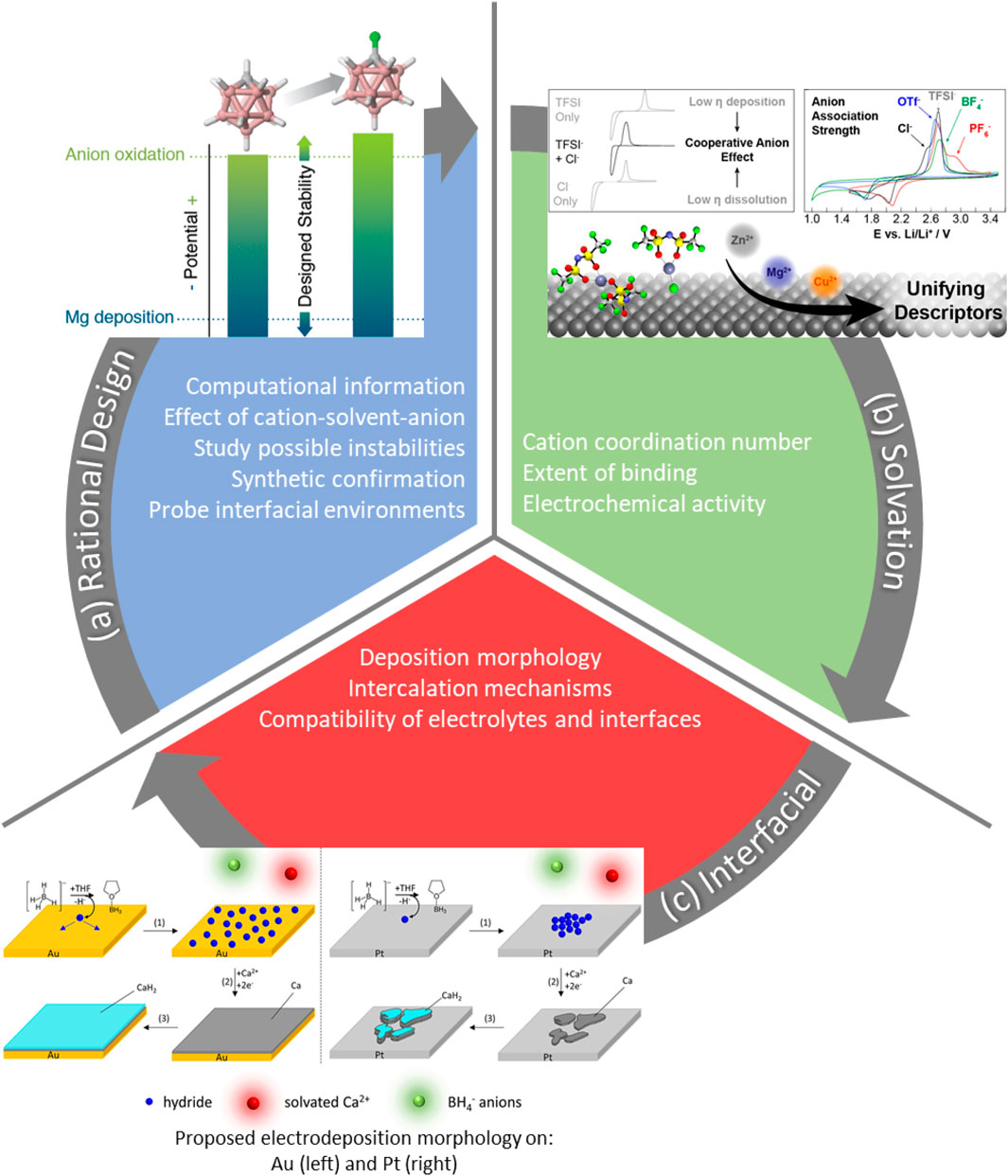
FIGURE 2. Possible outcomes from studying a feedback loop of rational design, solvation, and interfacial understanding with case-studies from literature: (A) computationally informed design of carbaborate electrolytes (Hahn et al., 2018), (B) electrochemical activity as a cause of varied additive binding (Connell et al., 2020), (C) morphological features of calcium deposition as a cause of plating surface (Ta et al., 2019) Reprinted (adapted) with permission from references (Hahn et al., 2018; Ta et al., 2019; Connell et al., 2020). Copyright (2021) American Chemical Society.
Solvation Environments
Electrolytes must have a high cation concentration to avoid issues with mass transport and maintain high conductivity. Early work focused on those electrolytes that displayed reversible plating and high solubility (Carter et al., 2014; Herb et al., 2016; Muldoon et al., 2017). While bulk solubility is essential, considering cation solvation helps understand detailed intermolecular interactions specific to plating and stripping. It is vital to both avoid CIP formation and encourage desolvation. Significant strides have occurred in studying liquid solvation properties and explaining linked electrochemical phenomena. In their nascence as electrolytes, WCAs had emerged to provide robustness and stability, but the explanation for this functionality was less understood.
Recent work studying the solvent-cation-anion relationship has brought to light the complexity of electrochemical plating and stripping in divalent electrolytes (Mohtadi et al., 2021). Despite the general assumption of weak coordination and basicity, it has been revealed that there is, in fact, cation-anion interaction in bulky alkoxyaluminates (Lau et al., 2019). Disfavoring this interaction by dilution helps avoid anion decomposition by Mg+ reduction while increasing anodic stability. The added stability was accomplished using a perfluoro-tert-butoxide ligand to form the TPFA− anion (tetrakis (perfluoro-tert-butoxy)aluminate) in place of the hfip ligand, with less electron withdrawing character. Electrochemical and computational data showed this system to further widen the overall electrochemical window relative to its previous counterparts. Using x-ray absorption measurements and infrared spectroscopy, Zn2+ solvation was observed in the analogous Zn [TPFA]2. Less CIP formation was seen with zinc at higher concentrations compared to magnesium (Yang et al., 2020). While the anion remains the same, each divalent system poses unique challenges due to its size, electrostatic charge, or even the anode surface reactivity.
Liquid solvation studies of commercial electrolyte systems have revealed the drastic influence of anion or solvent association on plating reversibility and stability. A seemingly simple study of divalent cations (Mg2+, Zn2+, and Cu2+) with TFSI− was used to describe the extent of anion association as a common property to related to deposition. Electrochemical methods revealed the role Cl− plays in improving divalent-ion electrodeposition with mixed anion electrolytes. The work was further bolstered by computational methods to generalize the anion-effect to other coordinating mixed systems, ranking their degrees of association (Figure 2B) (Connell et al., 2020).
In the absence of stronger associating X− ions, increased solvent coordination to Ca2+ or Zn2+ results in the difference between forming contact ion pairs or solvent separated ion pairs (Han et al., 2021). Higher chelating ethers have even been shown to block plating and stripping activity (Hahn et al., 2020). This phenomena was further studied in the context of a Ca [B (hfip)4]2 electrolyte that found cyclic ethers provide a less rigid solvation structure to Ca2+ as opposed to glyme solvents (Driscoll et al., 2020). Solvation studies have provided a strong basis for understanding how current anion systems may translate in different cation-solvent environments. Understanding solvent association gives the researcher an array of options to consider. Ultimately, solvent and anion choice afford the most tunability towards refining the practical system through ionic interactions. Provided the price of custom electrolytes adjusts with demand, they grant the best tools for future use in batteries.
Rational Design
Unfortunately, general screening and optimization used in chemistries like catalysis provide slower improvements to electrolytes. Traditionally, chemical intuition informs synthetic modification, while working against the hurdles purification at a multigram scale requires the luxury of time. This is particularly true for carboranes, where syntheses can be challenging to produce pure electrolytes at scale (Tutusaus et al., 2015; Hahn et al., 2018; Fisher et al., 2019). It is often underappreciated the care and proficiency required for pure electrolyte synthesis and incredibly dry conditions, without which results are rendered worthless.
Considering solvation studies, one can see the utility of cross-discipline work by confirming experimental results with the potential to expand observations with multiple electrolyte combinations. Opening a link between computational and experimental chemists can help lower the synthetic burden through rational design, where experimental understanding helps inform synthetic changes. This method has been demonstrated in which computed redox stabilities and degrees of ion-pairing were observed to change with corresponding variation in C-functionalization of a carbaborate anion (Hahn et al., 2018). Monofluorinated carbaborates were predicted to be more oxidatively stable than the unfunctionalized counterpart (Figure 2A). Confirmation of the model was carried out synthetically to show that the monofluorinated example was indeed more stable to higher potentials and maintained a favorable voltammetric response.
So far, computational efforts have reinforced experimental work in understanding the extents of ion-pairing, solvent-ion interaction, and in one case, optimizing electrolyte design. Concentrating on utilizing computation for rational design, and incorporating characterization of interfacial interactions will provide the feedback loop necessary for practical improvements.
Interfacial Understanding and Manipulation
The unique promise of divalent-ion batteries is the ability to safely use a metal anode in an energy-dense package. As a result, much of the field has focused on developing those DV electrolytes for reversible deposition and understanding mechanisms and resulting morphologies. Contrary to the introduction of many publications, multivalent ion systems still pose risks of irregular deposition morphologies, even dendrites, under specific conditions (Bucur et al., 2015; Ta et al., 2018; Ta et al., 2019).
As the cation solvation varies, so does the mechanism and morphology of deposition. Comparing Mg and Zn deposition mechanisms, zinc deposits through a simple two-electron transfer while magnesium undergoes a chemical-electrochemical transition involving a change in complexation (Ta et al., 2018). This study exposes the dependence of magnesium on its dimeric solvation structure discussed previously. Calcium borohydride electrolytes also undergo chemical-electrochemical transitions in plating (Ta et al., 2019). The complexity of the reactive calcium borohydride can also be dependent on the surface as the reaction at gold occurs slower than that at a platinum electrode. These different environments result in smooth, uniform deposits on gold and patchier deposits on platinum (Figure 2C).
In studying deposition, incompatible conditions have been observed, resulting in insulating decomposition deposits. For example, while borohydrides and alkoxyborates perform relatively well as magnesium electrolytes, reproducing those efficiencies proves difficult with the increased reactivity of a calcium system (Wang et al., 2018; Li et al., 2019; Shyamsunder et al., 2019; Ta et al., 2019). Unlike lithium ions, MV ions do not readily travel through passivated layers. Given the reactivity of high-voltage cathodes and metal anodes, what remains to be understood is the decomposition pathways and methods to monitor them effectively.
In MV-metal batteries, the overall energy density is determined by that of the cathode. Viable MV cathodes have been limited to sulfides, selenides, and organic cathode materials, but do not surpass the energy density of Li-ion batteries (Gu et al., 2015; Kwon et al., 2020b; Kim et al., 2020; Liang et al., 2020).
Current efforts to expand investigation into high energy density oxide cathodes have been plagued by irreversible electrochemistry and parasitic electrolyte decomposition, reflecting the reality that investigations of novel electrolytes at interfaces remain anode centric. However, recent studies of cathode materials have used electrolytes with greater stability at the electrolyte-cathode interface, such as those based on the TFSI− and TPFA− anions, to probe cathodic reactivity with greater confidence. Custom electrolytes, like MgTPFA, provide the best chance to enable further cathode development as opposed to commercial salts. Despite displaying the highest anodic stability, parasitic decomposition reactions are still observed even in these electrolyte systems, meaning that MV reactivity in cathode systems has to be holistically verified by structural, elemental, and redox probes as discussed in a recent perspective (Johnson et al., 2021). Therefore, the development of electrolytes or interfaces that are more stable with high voltage cathodes is crucial to advance MV battery technologies. Only through concerted efforts from the three themes discussed can we take lessons from cathode-electrolyte compatibility and further improve electrolytes through solvation study and rational design for practical gains.
Conclusion
Fundamental studies in solvation, rational design, and interfacial understanding will continue to play a crucial role in pushing electrolyte performance. There needs to be general methods and descriptors to scrutinize electrolyte fitness and assess intercalation in cathode materials to highlight high-performing components. Current methods of analysis are often post-mortem or bulk analyses. Discerning bulk behavior from anomalies at surfaces will help assess shortcomings in full cell batteries. Proposing theories to electrolyte incompatibility at the cathode interface needs the development of methods to tease out problems with the salt, solvent, and cathode. Coordinating efforts from different fields has resulted in new ways to study and develop pioneering MV ion electrolytes (Hahn et al., 2018; Lau et al., 2019; Driscoll et al., 2020), which has enabled the development of new cathode systems (Kwon et al., 2020a; Kwon et al., 2020b). Effectively utilizing these three themes in a circulating fashion holds promise as a robust framework to bridge the gap for practical next-generation batteries. The diverse crop of tools and components can lead us to fit the correct pieces to each unique energy storage technology.
Author Contributions
NL: Literature research, conceptualization, writing, editing, review. MH: Literature research, Mg-electrolyte review. CL: conceptualization, writing, editing, review.
Funding
Work done by Argonne National Laboratory was supported as part of the Joint Center for Energy Storage Research, an Energy Innovation Hub funded by the United States. Department of Energy, Office of Basic Energy Sciences, under contract DE-AC02-06CH11357.
Conflict of Interest
The authors declare that the research was conducted in the absence of any commercial or financial relationships that could be construed as a potential conflict of interest.
The handling editor declared a past co-authorship/collaboration with one of the authors CL.
Publisher’s Note
All claims expressed in this article are solely those of the authors and do not necessarily represent those of their affiliated organizations, or those of the publisher, the editors and the reviewers. Any product that may be evaluated in this article, or claim that may be made by its manufacturer, is not guaranteed or endorsed by the publisher.
Acknowledgments
The authors would like to acknowledge Ian Johnson and Brian Ingram of JCESR for their expert advice in multivalent battery systems and cathodes.
References
Arroyo-De Dompablo, M. E., Ponrouch, A., Johansson, P., and Palacín, M. R. (2019). Achievements, Challenges, and Prospects of Calcium Batteries. Chem. Rev. 120 (14), 6331–6357. 10.1021.acs.chemrev.9b00339
Aurbach, D., Lu, Z., Schechter, A., Gofer, Y., Gizbar, H., Turgeman, R., et al. (2000). Prototype Systems for Rechargeable Magnesium Batteries. Nature 407 (6805), 724–727. doi:10.1038/35037553
Aurbach, D., Weissman, I., Gofer, Y., and Levi, E. (2003). Nonaqueous Magnesium Electrochemistry and its Application in Secondary Batteries. Chem. Rec. 3 (1), 61–73. doi:10.1002/tcr.10051
Barile, C. J., Barile, E. C., Zavadil, K. R., Nuzzo, R. G., and Gewirth, A. A. (2014). Electrolytic Conditioning of a Magnesium Aluminum Chloride Complex for Reversible Magnesium Deposition. J. Phys. Chem. C 118 (48), 27623–27630. doi:10.1021/jp506951b
Bucur, C. B., Gregory, T., Oliver, A. G., and Muldoon, J. (2015). Confession of a Magnesium Battery. J. Phys. Chem. Lett. 6 (18), 3578–3591. doi:10.1021/acs.jpclett.5b01219
Carlson, R. B., Wishart, J., and Stutenberg, K. (2016). On-Road and Dynamometer Evaluation of Vehicle Auxiliary Loads. SAE Int. J. Fuels Lubr. 9 (1), 260–268. doi:10.4271/2016-01-0901
Carter, T. J., Mohtadi, R., Arthur, T. S., Mizuno, F., Zhang, R., Shirai, S., et al. (2014). Boron Clusters as Highly Stable Magnesium-Battery Electrolytes. Angew. Chem. Int. Ed. 53 (12), 3173–3177. doi:10.1002/anie.201310317
Connell, J. G., Zorko, M., Agarwal, G., Yang, M., Liao, C., Assary, R. S., et al. (2020). Anion Association Strength as a Unifying Descriptor for the Reversibility of Divalent Metal Deposition in Nonaqueous Electrolytes. ACS Appl. Mater. Inter. 12 (32), 36137–36147. doi:10.1021/acsami.0c09404
Davidson, R., Verma, A., Santos, D., Hao, F., Fincher, C., Xiang, S., et al. (2019). Formation of Magnesium Dendrites during Electrodeposition. ACS Energ. Lett. 4, 375–376. doi:10.1021/acsenergylett.8b02470
Denholm, P., O'Connell, M., Brinkman, G., and Jorgenson, J. (2013). Overgeneration from Solar Energy in California: A Field Guide to the Duck Chart. Golden, CO, United States: National Renewable Energy Lab (NREL).
Doe, R. E., Han, R., Hwang, J., Gmitter, A. J., Shterenberg, I., Yoo, H. D., et al. (2014). Novel, Electrolyte Solutions Comprising Fully Inorganic Salts with High Anodic Stability for Rechargeable Magnesium Batteries. Chem. Commun. 50 (2), 243–245. doi:10.1039/c3cc47896c
Driscoll, D. M., Dandu, N. K., Hahn, N. T., Seguin, T. J., Persson, K. A., Zavadil, K. R., et al. (2020). Rationalizing Calcium Electrodeposition Behavior by Quantifying Ethereal Solvation Effects on Ca2+ Coordination in Well-Dissociated Electrolytes. J. Electrochem. Soc. 167 (16), 160512. doi:10.1149/1945-7111/abc8e3
E-magic (2021). European Magnesium Interactive Battery Community (E-Magic). Available: www.e-magic.eu (Accessed January 25, 2021).
El Kharbachi, A., Zavorotynska, O., Latroche, M., Cuevas, F., Yartys, V., and Fichtner, M. (2020). Exploits, Advances and Challenges Benefiting beyond Li-Ion Battery Technologies. J. Alloys Comp. 817, 153261. doi:10.1016/j.jallcom.2019.153261
Fichtner, M. (2020). “Chapter 1: Motivation for a Magnesium Battery,” in Magnesium Batteries: Research and Applications; Energy and Environment Series. Croydon, UK: Royal Society of Chemistry, 1–16.
Fisher, S. P., Tomich, A. W., Guo, J., and Lavallo, V. (2019). Teaching an Old Dog New Tricks: New Directions in Fundamental and Applied Closo-Carborane Anion Chemistry. Chem. Commun. 55, 1684–1701. doi:10.1039/C8CC09663E
Forero-Saboya, J., Bodin, C., and Ponrouch, A. (2021). A boron-based Electrolyte Additive for Calcium Electrodeposition. Electrochemistry Commun. 124, 106936. doi:10.1016/j.elecom.2021.106936
Gaddum, L. W., and French, H. E. (1927). The Electrolysis of Grignard Solutions1. J. Am. Chem. Soc. 49 (5), 1295–1299. doi:10.1021/ja01404a020
Gao, T., Hou, S., Wang, F., Ma, Z., Li, X., Xu, K., et al. (2017). Reversible S 0/MgS X Redox Chemistry in a MgTFSI 2/MgCl 2/DME Electrolyte for Rechargeable Mg/S Batteries. Angew. Chem. Int. Ed. 56 (43), 13526–13530. doi:10.1002/anie.201708241
Gregory, T. D., Hoffman, R. J., and Winterton, R. C. (1990). Nonaqueous Electrochemistry of Magnesium: Applications to Energy Storage. J. Electrochem. Soc. 137 (3), 775–780. doi:10.1149/1.2086553
Gu, Y., Katsura, Y., Yoshino, T., Takagi, H., and Taniguchi, K. (2015). Rechargeable Magnesium-Ion Battery Based on a TiSe2-Cathode with D-P Orbital Hybridized Electronic Structure. Sci. Rep. 5 (1), 12486. doi:10.1038/srep12486
Hahn, N. T., Driscoll, D. M., Yu, Z., Sterbinsky, G. E., Cheng, L., Balasubramanian, M., et al. (2020). Influence of Ether Solvent and Anion Coordination on Electrochemical Behavior in Calcium Battery Electrolytes. ACS Appl. Energ. Mater. 3 (9), 8437–8447. doi:10.1021/acsaem.0c01070
Hahn, N. T., Seguin, T. J., Lau, K.-C., Liao, C., Ingram, B. J., Persson, K. A., et al. (2018). Enhanced Stability of the Carba-Closo-Dodecaborate Anion for High-Voltage Battery Electrolytes through Rational Design. J. Am. Chem. Soc. 140 (35), 11076–11084. doi:10.1021/jacs.8b05967
Han, K. S., Hahn, N. T., Zavadil, K. R., Jaegers, N. R., Chen, Y., Hu, J. Z., et al. (2021). Factors Influencing Preferential Anion Interactions during Solvation of Multivalent Cations in Ethereal Solvents. J. Phys. Chem. C 125 (11), 6005–6012. doi:10.1021/acs.jpcc.0c09830
Han, S.-D., Rajput, N. N., Qu, X., Pan, B., He, M., Ferrandon, M. S., et al. (2016). Origin of Electrochemical, Structural, and Transport Properties in Nonaqueous Zinc Electrolytes. ACS Appl. Mater. Inter. 8 (5), 3021–3031. doi:10.1021/acsami.5b10024
Herb, J. T., Nist-Lund, C. A., and Arnold, C. B. (2016). A Fluorinated Alkoxyaluminate Electrolyte for Magnesium-Ion Batteries. ACS Energ. Lett. 1 (6), 1227–1232. doi:10.1021/acsenergylett.6b00356
Jäckle, M., and Groß, A. (2014). Microscopic Properties of Lithium, Sodium, and Magnesium Battery Anode Materials Related to Possible Dendrite Growth. J. Chem. Phys. 141 (17), 174710. doi:10.1063/1.4901055
Jay, R., Tomich, A. W., Zhang, J., Zhao, Y., De Gorostiza, A., Lavallo, V., et al. (2019). Comparative Study of Mg(CB11H12)2 and Mg(TFSI)2 at the Magnesium/Electrolyte Interface. ACS Appl. Mater. Inter. 11 (12), 11414–11420. doi:10.1021/acsami.9b00037
JCESR (2021). Joint Center for Energy Storage Research. Available: https://www.jcesr.org/(Accessed January 25, 2021).
Johnson, I., Cabana, J., and Ingram, B. (2021). The Quest for Functional Oxide Cathodes for Magnesium Batteries: A Critical Perspective. ACS Energy Lett. 6 (5), 1892–1900. doi:10.1021/acsenergylett.1c00416
Kim, H. S., Arthur, T. S., Allred, G. D., Zajicek, J., Newman, J. G., Rodnyansky, A. E., et al. (2011). Structure and Compatibility of a Magnesium Electrolyte with a sulphur Cathode. Nat. Commun. 2 (427), 1–6. doi:10.1038/ncomms1435
Kim, S., Yin, L., Lee, M. H., Parajuli, P., Blanc, L., Fister, T. T., et al. (2020). High-Voltage Phosphate Cathodes for Rechargeable Ca-Ion Batteries. ACS Energ. Lett. 5 (10), 3203–3211. doi:10.1021/acsenergylett.0c01663
Kisu, K., Kim, S., Shinohara, T., Zhao, K., Züttel, A., and Orimo, S.-I. (2021). Monocarborane Cluster as a Stable Fluorine-free Calcium Battery Electrolyte. Sci. Rep. 11 (1). doi:10.1038/s41598-021-86938-0
Krossing, I., Brands, H., Feuerhake, R., and Koenig, S. (2001). New Reagents to Introduce Weakly Coordinating Anions of Type Al(ORF)4-: Synthesis, Structure and Characterization of Cs and Trityl Salts. J. Fluorine Chem. 112, 83–90. doi:10.1016/S0022-1139(01)00490-0
Krossing, I., and Reisinger, A. (2005). Perfluorinated Alkoxyaluminate Salts of Cationic Brønsted Acids: Synthesis, Structure, and Characterization of [H(OEt 2 ) 2 ][Al{OC(CF 3 ) 3 } 4 ] and[H(THF) 2 ][Al{OC(CF 3 ) 3 } 4 ]. Eur. J. Inorg. Chem. 2005 (10), 1979–1989. doi:10.1002/ejic.200400436
Kwon, B. J., Lau, K.-C., Park, H., Wu, Y. A., Hawthorne, K. L., Li, H., et al. (2020a). Probing Electrochemical Mg-Ion Activity in MgCr2-xVxO4 Spinel Oxides. Chem. Mater. 32 (3), 1162–1171. doi:10.1021/acs.chemmater.9b04206
Kwon, B. J., Yin, L., Park, H., Parajuli, P., Kumar, K., Kim, S., et al. (2020b). High Voltage Mg-Ion Battery Cathode via a Solid Solution Cr-Mn Spinel Oxide. Chem. Mater. 32 (15), 6577–6587. doi:10.1021/acs.chemmater.0c01988
Lau, K.-C., Seguin, T. J., Carino, E. V., Hahn, N. T., Connell, J. G., Ingram, B. J., et al. (2019). Widening Electrochemical Window of Mg Salt by Weakly Coordinating Perfluoroalkoxyaluminate Anion for Mg Battery Electrolyte. J. Electrochem. Soc. 166 (8), A1510–A1519. doi:10.1149/2.0751908jes
Lawrence Livermore National Laboratory (2020). U.S. Energy Use Rises to Highest Level Ever. Available: https://www.llnl.gov/news/us-energy-use-rises-highest-level-ever (Accessed February 7, 2021).
Li, Z., Fuhr, O., Fichtner, M., and Zhao-Karger, Z. (2019). Towards Stable and Efficient Electrolytes for Room-Temperature Rechargeable Calcium Batteries. Energy Environ. Sci. 12, 3496–3501. doi:10.1039/c9ee01699f
Liang, Y., Dong, H., Aurbach, D., and Yao, Y. (2020). Current Status and Future Directions of Multivalent Metal-Ion Batteries. Nat. Energ. 5 (9), 646–656. doi:10.1038/s41560-020-0655-0
Lipson, A. L., Han, S.-D., Pan, B., See, K. A., Gewirth, A. A., Liao, C., et al. (2016). Practical Stability Limits of Magnesium Electrolytes. J. Electrochem. Soc. 163 (10), A2253–A2257. doi:10.1149/2.0451610jes
Liu, T., Shao, Y., Li, G., Gu, M., Hu, J., Xu, S., et al. (2014). A Facile Approach Using MgCl2 to Formulate High Performance Mg2+ Electrolytes for Rechargeable Mg Batteries. J. Mater. Chem. A. 2 (10), 3430. doi:10.1039/c3ta14825d
Ma, L., Schroeder, M. A., Borodin, O., Pollard, T. P., Ding, M. S., Wang, C., et al. (2020). Realizing High Zinc Reversibility in Rechargeable Batteries. Nat. Energ. 5 (10), 743–749. doi:10.1038/s41560-020-0674-x
Mizrahi, O., Amir, N., Pollak, E., Chusid, O., Marks, V., Gottlieb, H., et al. (2008). Electrolyte Solutions with a Wide Electrochemical Window for Rechargeable Magnesium Batteries. J. Electrochem. Soc. 155 (2), A103. doi:10.1149/1.2806175
Mohtadi, R., Matsui, M., Arthur, T. S., and Hwang, S.-J. (2012). Magnesium Borohydride: From Hydrogen Storage to Magnesium Battery. Angew. Chem. Int. Ed. 51 (39), 9780–9783. doi:10.1002/anie.201204913
Mohtadi, R., Tutusaus, O., Arthur, T. S., Zhao-Karger, Z., and Fichtner, M. (2021). The Metamorphosis of Rechargeable Magnesium Batteries. Joule 5 (3), 581–617. doi:10.1016/j.joule.2020.12.021
Muldoon, J., Bucur, C. B., and Gregory, T. (2017). Fervent Hype behind Magnesium Batteries: An Open Call to Synthetic Chemists—Electrolytes and Cathodes Needed. Angew. Chem. Int. Ed. 56 (40), 12064–12084. doi:10.1002/anie.201700673
Pan, B., Huang, J., He, M., Brombosz, S. M., Vaughey, J. T., Zhang, L., et al. (2016a). The Role of MgCl2 as a Lewis Base in ROMgCl–MgCl2 Electrolytes for Magnesium‐Ion Batteries. ChemSusChem 9, 595–599. doi:10.1002/cssc.201501557
Pan, B., Huang, J., Sa, N., Brombosz, S. M., Vaughey, J. T., Zhang, L., et al. (2016b). MgCl2: The Key Ingredient to Improve Chloride Containing Electrolytes for Rechargeable Magnesium-Ion Batteries. J. Electrochem. Soc. 163 (8), A1672–A1677. doi:10.1149/2.0821608jes
Pan, B., Lau, K.-C., Vaughey, J. T., Zhang, L., Zhang, Z., and Liao, C. (2017). Ionic Liquid as an Effective Additive for Rechargeable Magnesium Batteries. J. Electrochem. Soc. 164 (4), A902–A906. doi:10.1149/2.1551704jes
Pan, B., Zhang, J., Huang, J., Vaughey, J. T., Zhang, L., Han, S.-D., et al. (2015). A Lewis Acid-free and Phenolate-Based Magnesium Electrolyte for Rechargeable Magnesium Batteries. Chem. Commun. 51, 6214–6217. doi:10.1039/c5cc01225b
Park, M. J., Yaghoobnejad Asl, H., and Manthiram, A. (2020). Multivalent-Ion versus Proton Insertion into Battery Electrodes. ACS Energ. Lett. 5 (7), 2367–2375. doi:10.1021/acsenergylett.0c01021
Ponrouch, A., Bitenc, J., Dominko, R., Lindahl, N., Johansson, P., and Palacin, M. R. (2019). Multivalent Rechargeable Batteries. Energy Storage Mater. 20, 253–262. doi:10.1016/j.ensm.2019.04.012
Pour, N., Gofer, Y., Major, D. T., and Aurbach, D. (2011). Structural Analysis of Electrolyte Solutions for Rechargeable Mg Batteries by Stereoscopic Means and DFT Calculations. J. Am. Chem. Soc. 133 (16), 6270–6278. doi:10.1021/ja1098512
Riddlestone, I. M., Kraft, A., Schaefer, J., and Krossing, I. (2018). Taming the Cationic Beast: Novel Developments in the Synthesis and Application of Weakly Coordinating Anions. Angew. Chem. Int. Ed. 57, 13982–14024. doi:10.1002/anie.201710782
Shterenberg, I., Salama, M., Gofer, Y., and Aurbach, D. (2017). Hexafluorophosphate-Based Solutions for Mg Batteries and the Importance of Chlorides. Langmuir 33 (37), 9472–9478. doi:10.1021/acs.langmuir.7b01609
Shterenberg, I., Salama, M., Yoo, H. D., Gofer, Y., Park, J.-B., Sun, Y.-K., et al. (2015). Evaluation of (CF3SO2)2N−(TFSI) Based Electrolyte Solutions for Mg Batteries. J. Electrochem. Soc. 162 (13), A7118–A7128. doi:10.1149/2.0161513jes
Shyamsunder, A., Blanc, L. E., Assoud, A., and Nazar, L. F. (2019). Reversible Calcium Plating and Stripping at Room Temperature Using a Borate Salt. ACS Energ. Lett. 4 (9), 2271–2276. doi:10.1021/acsenergylett.9b01550
Ta, K., See, K. A., and Gewirth, A. A. (2018). Elucidating Zn and Mg Electrodeposition Mechanisms in Nonaqueous Electrolytes for Next-Generation Metal Batteries. J. Phys. Chem. C 122 (25), 13790–13796. doi:10.1021/acs.jpcc.8b00835
Ta, K., Zhang, R., Shin, M., Rooney, R. T., Neumann, E. K., and Gewirth, A. A. (2019). Understanding Ca Electrodeposition and Speciation Processes in Nonaqueous Electrolytes for Next-Generation Ca-Ion Batteries. ACS Appl. Mater. Inter. 11 (24), 21536–21542. doi:10.1021/acsami.9b04926
Thackeray, M. M., Wolverton, C., and Isaacs, E. D. (2012). Electrical Energy Storage for Transportation - Approaching the Limits of, and Going beyond, Lithium-Ion Batteries. Energy Environ. Sci. 5, 7854–7863. doi:10.1039/C2EE21892E
Thomas, J. (2014). Drive Cycle Powertrain Efficiencies and Trends Derived from EPA Vehicle Dynamometer Results. SAE Int. J. Passeng. Cars - Mech. Syst. 7 (4), 1374–1384. doi:10.4271/2014-01-2562
Tian, Y., Zeng, G., Rutt, A., Shi, T., Kim, H., Wang, J., et al. (2020). Promises and Challenges of Next-Generation "Beyond Li-Ion" Batteries for Electric Vehicles and Grid Decarbonization. Chem. Rev. 121, 1623–1669. doi:10.1021/acs.chemrev.0c00767
Tutusaus, O., Mohtadi, R., Arthur, T. S., Mizuno, F., Nelson, E. G., and Sevryugina, Y. V. (2015). An Efficient Halogen-free Electrolyte for Use in Rechargeable Magnesium Batteries. Angew. Chem. Int. Ed. 54 (27), 7900–7904. doi:10.1002/anie.201412202
Verrelli, R., Black, A. P., Pattanathummasid, C., Tchitchekova, D. S., Ponrouch, A., Oró-Solé, J., et al. (2018). On the Strange Case of Divalent Ions Intercalation in V2O5. J. Power Sourc. 407, 162–172. doi:10.1016/j.jpowsour.2018.08.024
Wang, D., Gao, X., Chen, Y., Jin, L., Kuss, C., and Bruce, P. G. (2018). Plating and Stripping Calcium in an Organic Electrolyte. Nat. Mater 17 (1), 16–20. doi:10.1038/NMAT5036
Wu, Q., Shu, K., Sun, L., and Wang, H. (2021). Recent Advances in Non‐nucleophilic Mg Electrolytes. Front. Mater. 7 (435). doi:10.3389/fmats.2020.612134
Yang, M., Driscoll, D. M., Balasubramanian, M., and Liao, C. (2020). Solvation Structure and Electrochemical Properties of a New Weakly Coordinating Aluminate Salt as a Nonaqueous Electrolyte for Zinc Batteries. J. Electrochem. Soc. 167 (16), 160529. doi:10.1149/1945-7111/abcd46
Keywords: multivalent electrolytes, electrodeposition, magnesium electrolyte, interfaces, solvation
Citation: Leon NJ, He M and Liao C (2022) Solvation, Rational Design, and Interfaces: Development of Divalent Electrolytes. Front. Energy Res. 9:802398. doi: 10.3389/fenrg.2021.802398
Received: 26 October 2021; Accepted: 13 December 2021;
Published: 05 January 2022.
Edited by:
Ka Cheong (Tim) Lau, Evonik Corporation, United StatesReviewed by:
Rana Mohtadi, Toyota, United StatesCopyright © 2022 Leon, He and Liao. This is an open-access article distributed under the terms of the Creative Commons Attribution License (CC BY). The use, distribution or reproduction in other forums is permitted, provided the original author(s) and the copyright owner(s) are credited and that the original publication in this journal is cited, in accordance with accepted academic practice. No use, distribution or reproduction is permitted which does not comply with these terms.
*Correspondence: Chen Liao, bGlhb2NAYW5sLmdvdg==