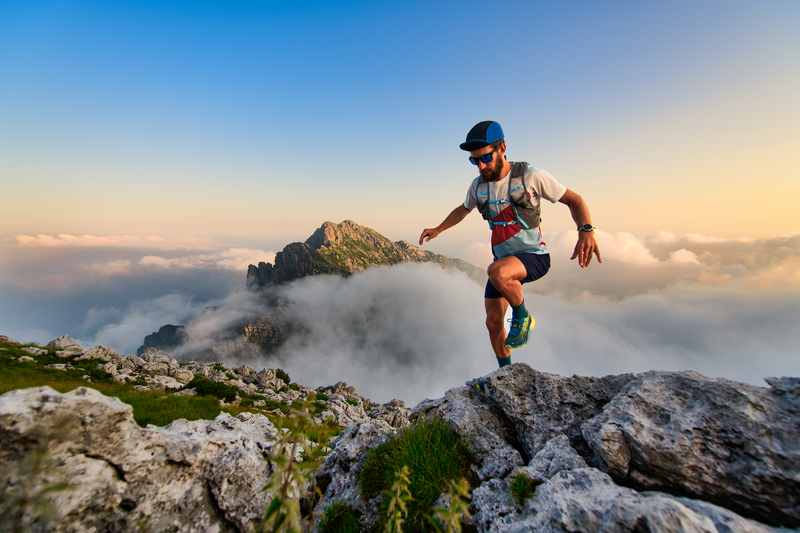
94% of researchers rate our articles as excellent or good
Learn more about the work of our research integrity team to safeguard the quality of each article we publish.
Find out more
BRIEF RESEARCH REPORT article
Front. Energy Res. , 13 December 2021
Sec. Bioenergy and Biofuels
Volume 9 - 2021 | https://doi.org/10.3389/fenrg.2021.794682
Energy resources, and their management, represent an open ongoing problem of our present days. An increasing interest in the analysis of the limits of fossil fuels’ use, and their availability, is growing in order to find solutions to the undesired impact of some anthropic activities to the environment. So, nowadays, aThe current shift to renewable energy resources has become a fundamental requirement. In this context, biofuels from micro-organisms can represent a response to the requirement of reducing the environmental impact, but also to generatinge new jobs. In this paper, the analysis of the biofuels from micro-organisms is developed by introducing the Thermodynamic Human Development Index (THDI). In particular, we show how its performance can be improved by using the third-generation biofuels in the road transport sector, and how it increases by exploiting biofuels derived from mutualistic species of some micro-organisms. The result consists inis affected by the fundamental role of the mutualistic behaviour of these species in order to increase the overall sustainability.
Since the second decade of the XIX centuryIn the 1820s, Jean Baptiste Joseph Fourier (1768–1830) developed the analysis of the Earth’s temperature in relation to the distance from the Sun; indeed, he conjectured that the Earth’s temperature results is greater than it should be due to the partial outflow of the infrared radiation from the atmosphere (Fourier, 1822), today named the greenhouse effect. This effect was experimentally proven, in 1859, by John Tyndall (1820–1893) who showed the radiant heat trapping property of carbon dioxide (CO2) and water vapour (Moriarty and Honnery, 2011a). These studies allowed Svante August Arrhenius (1859–1927) to evaluate speculate that a doubling of atmospheric CO2 concentrations can increase the Earth’s temperature of by around 4°C.
Even if controversy has been developed on global climate change for a long timeAlthough controversy has surrounded the topic of global climate change, today, it is clear the effect of the anthropogenic activities on the climate change is now clear (Arango-Miranda et al., 2018). Indeed, industrialisedindustrialized societiesy consumes large amounts of energy, mainly generated by using fossil fuels, both for the electric production and for the transportations sectors. The gGreenhouse gas emissions haveis a well-established effect (Torok and Dransfield, 2017; Qiao et al., 2019), due to the combustion processes involved in current energy systems, but, recently, alsoRecently, however, the effect of the related wasted heat, due to human activities, has also been considered (Flanner, 2009; Manowska and Nowrot, 2019), in order to analyzse the global warming and the climate change.
Moreover, worldwide economic development and population growth, allow us to conjecture that there will be an increase in the energy demands at all sectoral levels , observing that tThe world energy consumption in 1965 and 2019 were respectively 155.69 EJ and 583.88 EJ (British Petroleum, 2021), with a related decrease in its availability, or and there is the a need to change the energy use and production before 2050 (Arango-Miranda et al., 2018).
Therefore, new strategies and technologies are required in order to go effectivelyprogress towards an energetic transition, and more sustainable and decarbonized societies (Karvonen et al., 2017). In this context, the sustainable consumption and production policies represent the key pointskey ways in order to realize sustainable development, with particular regards to environmental sustainability and greenhouse gases emissions. So, economic and social well-being, environmental recovery and protection, and anthropic emissions’ reduction must be better integrated into our common practices and policies (McGlade, 2007). But, indicators are required to make and communicate policies; they are the essential tools to assess and set policies, collect data, and perform future projections. Indeed, indicators are useful to inform policy-makers, scientists, and people on current conditions, but also assist to identify adequate actions, and assess their effectiveness (McCool and Stankey, 2004). The need to dispose of sustainable development indicators, as the main tool to quantify and assess the cCountries’ performances towards sustainability, has also been highlighted also in Chapter 40 of the United Nations Action Plan Agenda 21 (United Nations General Assembly, 1992; Strezov et al., 2016).
Thus, researchers, stakeholders, governmental panels, and international organizations, etc. have made big significant efforts in order to build and introduce several sustainability indicators, even if some concerns have emerged in defining and using them effectively them, mostly due to the fact that sustainable development is a multi-stakeholder and interdisciplinary process, that implies involves considering to consider together all the different sectors and actors involved together (Moldan and Dahl, 2007; Scerri and James, 2010; Edmonds et al., 2017).
Many works have been developed in order to introduce and review sustainability indicators, such as in Refs (Munda, 2005; Böhringer and Jochem, 2007; Wilson et al., 2007; Moran et al., 2008; Nourry, 2008; Steinberger, 2008; Fiksel et al., 2012; Evans et al., 2015; Dong and Hauschild, 2017; Liu et al., 2017; Neri et al., 2021), which have also referred to specific sectors, like the energy one sector (Hacatoglu et al., 2015; Sciubba, 2019; Gunnarsdottir et al., 2020). In the following subsection, we will only summarizse some of them, in order to present a short summary.
The Index of Sustainable Economic Welfare (ISEW) was proposed by Cobb (Cobb, 1989) as an alternative to the Gross Domestic Product (GDP). Subsequently, this indicator was improved by the author himself (Cobb and Cobb, 1994; Cobb et al., 1999), expanding it also to the remaining pillars of sustainability, by developing the Genuine Progress Indicator (GPI). However, its main weak point has been identified in the non-unique methodology used to calculate it (Pais et al., 2019).
The Ecological Footprint (EF) constitutes an account-based system of indicators, which considers the use of the Earth’s finite resources (Lin et al., 2018), measuring the load imposed by anthropic activities on nature. EF accounts for the productive land, useful to support a given population in relation to its level of consumption (Rees, 1992; Wackernagel and Rees, 1997; Moldan et al., 2012) giving information on the anthropic impacts on the ecosystems and biodiversity. However: it covers only the environmental pillar of sustainability, but the methods to calculate it are non-unified.
The Genuine Savings Indicator (GSI) measures the variations in total broad capital stocks, year by year, and gives an indication on how a society manages them, in order to generate streams of well-being over time (Hamilton and Naikal, 2014; Labat and Willebald, 2019). However, recently to date, using with a large time series (1800–2000) related to Sweden, no one-to-one relationship between GSI and well-being has been found (Lindmark et al., 2018).
The Environmental Sustainability Index (ESI) is a composite index to evaluate sustainability, which encloses 21 different indicators (environmental, social, and economic), combined with a finite number of variables (from two to eight) (Esty et al., 2005). Moreover, this composite index has been subsequently modified by adding other environmental indicators, and human-health- related indicators, developing the Environmental Performance Index (EPI) (Hsu et al., 2013).
Another composite index that has been developed in order to measure the level of sustainable development of a country is the Sustainable Society Index (SSI), which includes 22 different indicators, classified in five main groups (personal development, clean environment, well-balanced society, sustainable use of resources, and sustainable world) (van de Kerk and Manuel, 2008); these groups are not aggregated to an overall score, due to the correlation between human and environmental well-being (Burgass et al., 2017).
The Climate Change Performance Index (CCPI) allows us to assess and compare the climate protection level of the countries, that actually emit more than 90% of global energy-related carbon dioxide emissions. The overall score is aggregated from three main categories, which present different weights: emissions trend, emissions level, and climate policies (Burck et al., 2014).
The Economy-wide material flow indicators are a framework of aggregated indicators developed by Eurostat, based on economy-wide material flow analysis. The focus of this these indicators is the environmental pillar of sustainable development. The same characteristic can be highlighted for the European Environment Agency (EEA) core set indicators (Moldan and Dahl, 2007). We can highlight that the OECD has created its own Core Environmental Indicators (CEI), too. Moreover, in order to measure the state of the world’s biodiversity, the Living Planet Index (LPI) was also introduced, including data related to human pressures on natural ecosystems, due to the consumption of natural resources, and the consequences of pollution included.
The Well-being Index (barometer of sustainability) combines 36 indicators with other 5187 indicators ones into two indices, which are then aggregated into one single indicator, which measures how much human well-being of a country can be obtained, weighting the effect on the environment (Moldan and Dahl, 2007).
In 2006, the Happy Planet Index (HPI) was built in order to act as an alternative to GDP, measuring the environmental impact of a country, in relation to the ability of its citizens to live a long and happy life (Tausch, 2011). It is based on three variables: the lLife eExpectancy at birth, the eEcological fFootprint, and lLife sSatisfaction. The main concern, about HPI, is that lLife sSatisfaction is a subjective variable to measure the well-being (Campus and Porcu, 2010).
Another well-known indicator of sustainable development and human well-being is the Human Development Index (HDI), which was introduced by the United Nations Development Programme in the earlies 1990s (UNDP Human Development Report Office, 1990), which and has been updated and improved during the lastin recent years (UNDP Human Development Report Office, 2010; UNDP Human Development Report Office, 2015; Hickel, 2020). It is a multidimensional index, measuring the development of a country from a socio-economic stand-point, focusing in on human well-being, by considering key parameters of social development (Sagar and Najam, 1998; Hickel, 2020). The main advantage, which has favoured the spread of this indicator, has been the small number of variables included, even if this property has been also identified, at the same time, as its weak point. The HDI is a composite index, measuring a country’s average achievements in three fundamental aspects of human well-being. However, the main criticism related to this sustainable development indicator is that it does not consider the environmental domain.
Recently, the Thermodynamic Human Development Index THDI has been introduced (Lucia and Grisolia, 2021a), in order to enclose alsoalso include the environmental domain of sustainable development into the HDI, considering the greenhouse gases emissions by means of the fundamental thermodynamic quantity and, the entropy generation (Lucia et al., 2020). Indeed, by linking the first to the second law of thermodynamics, the Gouy-Stodola theorem can be proven: this theorem provides information about the available work lost during any process, quantifying the irreversibility of the process itself (Bejan, 2006).
As previously highlighted, the issue of reducing CO2 emissions due to the anthropic activities is one of the challenges of this century. Moreover, in relation to the global climate change, two different approaches have been suggested: mitigation (Barker et al., 2007) and adaptation (Parry et al., 2007). Here, we don’t do not develop any discussion on adaptation, while as we are interested in mitigation by the use of biofuels. Mitigation actions are based on the reduction of the CO2 and greenhouse gasses concentrations in the atmosphere, by reducing the emissions, increasing the storage, and introducing renewable energy sources.
Considering the transport sector, which encloses encompasses aviation, maritime, rail, heavy-duty vehicles, and light-duty vehicles, and passenger vehicles, it consumes about the 20% of the total global energy produced, accounting for about 24% of the total direct CO2 emissions (International Energy Agency, 2021). Thus, the use of biofuels in the transport sector, and everywhere anywhere the present technologies cannot be replaced by more sustainable ones, like in the developing countries, can reduce their environmental impact (Demirel, 2018b). Indeed, decarbonisingdecarbonizing transport requires a wide range of bio-based transport fuels (Alizadeh et al., 2020; Brown et al., 2020), especially advanced low-carbon fuels.
In this context, algae-based biofuels represent an interesting technology both to improve energy security and to reduce the CO2 emissions from the transportation sector (Moheimani et al., 2015; Allen et al., 2018). Biofuels include products derived from biomasses, or their residues. They contain around 80% of renewable materials constituted by biomass, obtained by photosynthesis processes, which represent carbon sequestration with energy storage and little low sulphursulfur content. Biofuels can be classified in four different sets (Demirel, 2018a; Ruan et al., 2019):
• The first generation one involves the use of agricultural biomass products, mainly derived from food-based biomasses (i.e., corn, sugar-cane, soybean, oil seeds, vegetables, etc.); they present ethical consequences in relation to the food supply chain and may not be sustainable (Demirel, 2016), even if they represent the first proof of response to energy security and global warming by using sugar and lipid platforms, without the need for new infrastructures for feedstock delivery and biomass-to-biofuel conversion (Demirel, 2018b);
• The second generation one involves the use of non-food-biomass (cellulose and hemicellulose) and agricultural waste products. Nevertheless, these biofuels are not yet profitable due to the need of for technological improvements for in biorefinery in relation to the use of waste lignin as a feedstock for new chemicals and products (Demirel, 2018b);
• The third generation one involves the use of micro-organisms such as algae, microalgae, yeasts, fungi, bacteria, and cyanobacteria: algal technology coupled with wastewater treatment facilities and the anaerobic digestion represent some of the present improvements of biofuels towards a sharper sustainability;
• The fourth generation one involves the use of engineered micro-organisms, or genetically modified living organisms, in order to improve the production of their useful co-products.
Nowadays, bioethanol and biodiesel are the most used biofuels (Demirel, 2018a), even if they are mostly derived from edible crops. This trend must be vary, due to ethical concerns and negative consequences on land use (Indirect Land Use Change) (Parra Paitan and Verburg, 2019). In order to reduce these negative effects, it is important to produce biofuels in alternative ways, and policy guiding this sector must ensure that biomass is derived sustainably (Collotta et al., 2019). In order to do so, biorefineries with multigeneration technologies are continuously improving with the development towards their integration into the existing fuels networks. In this context, biofuels from algae and cyanobacteria represent an important response to the constraints for biofuels production and use (Demirel, 2018b), such as sustainability, reduction in greenhouse gas emissions, local food security, energy conservation, soil, air and water protection, and land rights. Moreover, other fundamental aspects of sustainability are related to the biofuel productions such as human and labour rights, but also legality, rural and social development, technology improvements, and wastes treatments (Demirel, 2018b). Indeed, biofuel production could cause some adverse consequences on biological diversity through land conversion, introduction of invasive species, soil and water consumption in agriculture, habitat loss, and nutrient pollution. But, on the other hand, we must also consider that society, the environment, and the economy could benefit from a sustainable biofuels’ production and use, if well developed.
All these topics must be taken into account in the analyses of biofuels’ production and in their future developments.
The aim of this paper is to analyzse a viable way to optimize the third generation of biofuels by introducing a thermodynamic viewpoint. Indeed, we wish to introduce in this context a new indicator (THDI) (Lucia and Grisolia, 2021a), recently developed just to consider both the human well-being and the environmental impact of the human activities. In order to do so, first we summarisesummarize the fundamental aspects of this indicator, then we use it in the thermodynamic analysis of biofuels, with particular regards to the possible optimizsation of their production, based on the spontaneous behaviour of the living beings (mutualism between different species).
Consequently, the fundamental result consists in of pointing out how mutualism can improve the sustainability of biofuels derived from micro-organisms.
Since In 1990, the United Nations have introduced the Human Development Index (HDI) to measure the development level of a country, with particular regards to human well-being in relation to: education, health, and salary conditions (Javaid et al., 2018), by a geometric mean of three related normalizsed indices (UNDP Human Development Report Office, 1990; United Nations Development Program, 2020):
where:
• LEI = (LE − 20)/65 is the Life Expectancy Index, where LE is the Life Expectancy at birth, related to the overall mortality level of a population. It quantifies the years expected a newborn is expected to live at present mortality rates (World Bank Group, 2021);
• EI = 0.5 (MYSI + EYSI) is the Education Index (Saisana, 2014), with MYS/15 the Mean Years of Schooling Index and EYSI = ESI/18 the Expected Years of Schooling Index (United Nations Development Program, 2020);
• II = ln(0.01 GNIpc)/ln(750) is the Income Index, with GNIpc the gross national income per capita at purchasing power parity (PPP), with minimum and maximum values set by the United Nations (United Nations Development Program, 2020) as 100.00 $ and 75, 000.00 $, respectively.
This indicator is considered as a measure of sustainability. However, it represents a socio-economic approach to the analysis of the countries, without considering their environmental impact and their technological level. So, recently, in order to introduce the technological level and the environmental impact into the United Nation index HDI, we have improved it by considering the Gouy-Stodola theorem and, consequently, the entropy variation due to irreversibility, which is inversely proportional to the technological level of a country and to the effects of its technological level to the environment (Bejan, 2006; Lucia, 2013; Lucia, 2016; Lucia and Grisolia, 2017; Grisolia et al., 2020; Lucia et al., 2020):
where T0 is the environmental temperature,
where it is possible to introduce a relation between
where
The THDI expresses both the well-being and the well-behaviour in relation to sustainability of a country. In particular, the more the country is sustainable, the higher more the value of THDI is high.
So, from Eq. 2, it follows that a decrease in the environmental impact of a country could be achieved by decreasing its carbon dioxide emissions
To do so, we consider that, when more species co-exist in the same ambient, for some of them, symbiosis can occur (Willey et al., 2013; Oulhen et al., 2016). It is the association between at least two distinct species of living organisms (Douglas, 1994; Paracer and Ahmadjian, 2000; Willey et al., 2013) and represents a fundamental natural behaviour of living species for life development and evolution (Boucher et al., 1982; Bronstein, 1994; Dimijian, 2000; De Mazancourt et al., 2005; Stanley, 2006; Peacock, 2011; Martin and Schwab, 2013; Munzi et al., 2019). Symbiosis is a set of cooperative phenomena in biology, with different behavioural classifications. Here, we consider the mutualism, a symbiotic association in which each symbiont gets a benefit (Martin and Schwab, 2013).
So, when two species of microorganisms (i.e., bacteria, bacteria/alga, alga/alga) act together, they absorbed exergy Exin and release useful products to obtain biofuels, bioplastics, or other bioproducts. To develop our analysis, we consider that the biofuels production, from photosynthetic microorganisms is related to the biomass productivity and the amount of lipids stored inside their cells. So, in order to evaluate the effect of a mutualistic production of biofuels, we consider the lipid mass production as a useful required effect required.
To do so, we consider first the lipid production of two species separately (indicated respectively with the subscript 1 and 2), and then we compare it with the lipid production of their symbiotic condition. Therefore, when the two species live separately, they release:
• The mass of useful products m1 and m2;
• The energy (m1 + m2)hf, where hf is the formation enthalpy of the product (Demirel and Sandler, 2002; Demirel, 2010; Grisolia et al., 2020);
• In this case, Inm = T0Sg,nm/Exin, where the subscript nm indicates the non-mutualistic condition, while, if the species live in a mutualistic condition, they release:
• The mass of useful products m1 + m2 + Δm, where the amount Δm corresponds to the increase in their production due to mutualism;
• The energy (m1 + m2 + Δm)hf;
• In this case, Im = T0Sg,m/Exin = Inm + (Inm − 1) ⋅Δm/(m1 + m2) (Grisolia et al., 2020), where the subscript m indicates the mutualistic condition.
Last, after some algebraic manipulations, the consequent effect into the Thermodynamic Human Development Index results in:
where we have considered that:
and also that:
where ηr( < 1) is the efficiency of the chemical formation reaction of the biomass. Consequently, the ratio in Eq. 5 results always graterproduces a result greater than 1.
In this Section, we develop some examples of the use of the analytical results obtained in the previous Section, by considering the production of biofuels that involves photosynthetic microorganisms. In particular, we analyzse the results due to their co-cultivation, exploiting their natural positive symbiotic interactions, in order to increment their lipid productivity.
Photosynthesis is the bio-process that leads to complex organic molecules starting from simple molecules and by absorbing solar radiation (Andriesse and Hollestelle, 2001; Albarrán-Zavala and Angulo-Brown, 2007). Any species presents a metabolic pathway to live; consequently, we could examine any single metabolic pathway to obtain numerical evaluation. But, in Ecology and Biology, it is possible to develop a general analysis by considering the following chemical reaction, for plants and cyanobacteria, combining different mass production for any species pathway (Albarrán-Zavala and Angulo-Brown, 2007; Lucia and Grisolia, 2018):
The mean efficiency value of the chemical reaction formation of the biomass results ηr = 27.288% (Albarrán-Zavala and Angulo-Brown, 2007).
By using the data collected in Refs (Asmare et al., 2014; Rashid et al., 2019; Zhao et al., 2014), three different examples of comparison for the mutualistic and non-mutualistic cultivation can be summarizsed as follows:
• Coproduction of Scenedesmus dimorphus and Chlorella vulgaris (Asmare et al., 2014):
• Scenedesmus dimorphus lipid mass produced in mono-cultivation m1 = 68.15 ± 13.89 mg L−1;
• Chlorella vulgaris lipid mass produced in mono-cultivation m2 = 11.46 ± 0.84 mg L−1;
• Mutualistic lipid production of Ettlia sp. and Chlorella sp. m1 + m2 + Δm = 101.6 ± 15.11 mg L−1;
• Coproduction of Ettlia sp. and Chlorella sp. (Rashid et al., 2019):
• Ettlia sp. lipid mass produced in mono-cultivation m1 = 30.3 ± 4.7 mg L−1;
• Chlorella sp. lipid mass produced in mono-cultivation m2 = 201.7 ± 8.2 mg L−1;
• Mutualistic lipid production of Ettlia sp. and Chlorella sp. m1 + m2 + Δm = 353.7 ± 6.0 mg L−1;
• Coproduction of Chlorella sp. and Monoraphidium sp. (Zhao et al., 2014):
• Chlorella sp. lipid mass produced in mono-cultivation m1 = 370.6 ± 177.3 mg L−1;
• Monoraphidium sp. lipid mass produced in mono-cultivation m2 = 95.6 ± 26.7 mg L−1;
• Mutualistic lipid production of Chlorella sp and Monoraphidium sp. m1 + m2 + Δm = 592.6 ± 184.6 mg L−1.
These numerical evaluations allow us to stress that the mutualism represents a possible improvement in the biofuels production, related to the reduction of environmental impact.
Now, we consider the data for the Italian road transport, summarizsed in the Report (Romano et al., 2021). In 2019, the Italian road transport carbon dioxide emissions were 97.739
• The carbon dioxide emissions from the use of biodiesel from micro-organisms, related to the present conditions;
• The carbon dioxide emissions from the use of biodiesel from micro-organisms in mutualistic conditions, related to the present conditions, in relation to the three previous cases of co-cultivation. In Figure 1 the results obtained are represented.
FIGURE 1. The Italian case (2019 data): ratio between the THDI values in three different cases: THDIpresent, which considers the actual carbon dioxide emissions of road transport; THDIbio, which considers the carbon dioxide emissions in the case of use of biodiesel from micro-organisms instead of the actual supply and THDImutualistic, which considers the case of mutualistic biodiesel use.
The Figure 1 shows that the use of biofuel from microalgae reduces the CO2 emissions, and also that the mutualistic conditions improve this decrease. Consequently, the mutualistic conditions result in a powerful way to improve the sustainability of the biofuels’ use. Indeed, the values of THDI result higher in the biofuel scenario, and, moreover, the value of the mutualistic condition is even greater than the biofuels one.
Nowadays, the present energy resources, and their management, must be analysedanalyzed in relation to their future availability (Moriarty and Honnery, 2011b). Indeed, recently, there has been an increasing interest in the analysis of the limits of fossil fuels use and their availability, but also in the ecosystem in relation to fresh water and air pollution (Beddoe et al., 2009; Day et al., 2009), with particular regards to (Rockström et al., 2009): climate change, rate of biodiversity loss, nitrogen cycle and the phosphorus cycle, stratospheric ozone depletion, ocean acidification, global freshwater use, change in land use, atmospheric aerosol loading, and chemical pollution. These analyses have pointed out that human activities could have already exceeded safe limits; indeed, in relation to climate change, atmospheric CO2 peak levels are 420 ppm compared with the recommended threshold of 350 ppm (Moriarty and Honnery, 2011b). This implies several challenges across multiple sectors. So, sustainable policies play a central role in our present dayscurrent society and for the next generations, too: policy-makers, stakeholders, and people could should take into account the actual and future needs of social, economic, and environmental systems. This goal can be facilitated by adopting indicators, needed to assess progress towards goals for sustainability (Shields et al., 2002).
As concerns sustainability indicators, it has been highlighted that most indicator sets of sustainable development neglect some of the fundamental aspects of sustainable development itself, or the interlinkage among all the domains, despite their relevance in policy-making (Moldan and Dahl, 2007).
At present, a widespread approach to overcome the limits in the fossil fuels provisions is represented by renewable energy technologies (Ausubel and George, 2009); indeed, some considerations have been developed on the possibility to replace fossil fuels with non-conventional ones, and on the possible mitigation of the global climate change by introducing, on a large-scale of use, new non-carbon energy sources, carbon capture and sequestration, and geoengineering (Moriarty and Honnery, 2011b).
Then, a shift to renewable energy resources is a fundamental requirement of our time. Just considering only the solar radiation captured by the Earth, it is possible to understand the important role that the renewable energy could play in the human life; indeed, the power output from the Sun is
But, this energy can be stored in the biomass obtained by microorganisms, and it can be used in order to obtain biofuels, which can support humans to decrease their impact on the Earth system.
In this context, microalgae represent a possible pathway to obtain biomass feedstock, in order to produce biofuels. This can be linked to different characteristics of microalgae (Lucia et al., 2021b):
1. They can provide a continuous biomass supply, due to the non-seasonality of their harvesting;
2. They can be harvested in all kinds of water, such as: seawater, freshwater, or wastewater, that can result in a fewerless freshwater consumption for their harvesting and the possibility to use areas not otherwise exploitable;
3. They have a short doubling time during their exponential growth, usually less than 3.5 h;
4. They present a great potential yield per unit of cultivated area, if compared to land-based crops, presenting a biofuel yield 10–102 times greater;
5. Their ability to live in mutualistic conditions.
In this paper, we have suggested to introduce the mutualism as a possible improvement of biofuels’ production, and we have analyzsed it by means of a recently developed thermodynamic indicator, related to human well-being, which encloses the main socio-economic quantities, already used in the HDI, and an environmental related one. The introduction of a thermodynamic quantity into this indicator, directly related to irreversibility by the Gouy-Stodola theorem, brings to some advantages that can be summarizsed as follows (Demirel, 2002):
• It allows us to see the unavoidable footprint of each process;
• It allows us to consider the energy quality: it is a measure of the useful energy wasted during a process, and the degradation of the performance of any engineering system;
• It allows us to introduce engineering optimisationoptimization methods to improve the performance of a system, which means also to takealso taking into account the technological level of any process considered.
We have shown that the use of biofuels derived from micro-organisms can improve the performance of the THDI, by reducing the GHG emissions, and this behaviour increases by using biofuels, obtained from two positive symbiont species.
The original contributions presented in the study are included in the article/supplementary material, further inquiries can be directed to the corresponding author.
UL: Conceptualization; Methodology; Formal analysis; Investigation; Writing—Original Draft; Writing—Review and Editing; Supervision; Project administration; Funding acquisition. GG: Methodology; Investigation; Writing—Review and Editing; Visualization; Data Curation.
The authors declare that the research was conducted in the absence of any commercial or financial relationships that could be construed as a potential conflict of interest.
All claims expressed in this article are solely those of the authors and do not necessarily represent those of their affiliated organizations, or those of the publisher, the editors and the reviewers. Any product that may be evaluated in this article, or claim that may be made by its manufacturer, is not guaranteed or endorsed by the publisher.
Albarrán-Zavala, E., and Angulo-Brown, F. (2007). A Simple Thermodynamic Analysis of Photosynthesis. Entropy 9, 152–168. doi:10.3390/e9040152
Alizadeh, R., Lund, P. D., and Soltanisehat, L. (2020). Outlook on Biofuels in Future Studies: A Systematic Literature Review. Renew. Sustain. Energ. Rev. 134, 110326. doi:10.1016/j.rser.2020.110326
Allen, J., Unlu, S., Demirel, Y., Black, P., and Riekhof, W. (2018). Integration of Biology, Ecology and Engineering for Sustainable Algal-Based Biofuel and Bioproduct Biorefinery. Bioresour. Bioproc. 5, 47. doi:10.1186/s40643-018-0233-5
Andriesse, C. D., and Hollestelle, M. J. (2001). Minimum Entropy Production in Photosynthesis. Biophys. Chem. 90, 249–253. doi:10.1016/s0301-4622(01)00146-6
Arango-Miranda, R., Hausler, R., Romero-López, R., Glaus, M., and Ibarra-Zavaleta, S. (2018). An Overview of Energy and Exergy Analysis to the Industrial Sector, a Contribution to Sustainability. Sustainability 10, 153. doi:10.3390/su10010153
Archer, C., and Caldeira, K. (2009). Global Assessment of High-Altitude Wind Power. Energies 2, 307–319. doi:10.3390/en20200307
Asmare, A., Demessie, B., and Murthy, G. (2014). Investigation of Microalgae Co-cultures for Nutrient Recovery and Algal BiomassProduction from Dairy Manure. Appl. Eng. Agric. 30, 335–342. doi:10.13031/aea.30.10151
Barker, T., Bashmakov, I., Bernstein, L., Bogner, J., Bosch, P., Dave, R., et al. (2007). “Summary of Policymakers,” in Technical Summary: Mitigation of Climate Change. Editors B. Metz, O. R. Davidson, P. R. Bosch, R. Dave, and E. L. Meyer (Cambridge: IPPC).
Beddoe, R., Costanza, R., Farley, J., Garza, E., Kent, J., Kubiszewski, I., et al. (2009). Overcoming Systemic Roadblocks to Sustainability: The Evolutionary Redesign of Worldviews, Institutions, and Technologies. PNAS 106, 2483–2489. doi:10.1073/pnas.0812570106
Böhringer, C., and Jochem, P. E. P. (2007). Measuring the Immeasurable - a Survey of Sustainability Indices. Ecol. Econ. 63, 1–8. doi:10.1016/j.ecolecon.2007.03.008
Boucher, D. H., James, S., and Keeler, K. H. (1982). The Ecology of Mutualism. Annu. Rev. Ecol. Syst. 13, 315–347. doi:10.1146/annurev.es.13.110182.001531
British Petroleum (2021). BP Statistical Review of World Energy 2020. [Dataset]. Available at: http://www.bp.com/statisticalreview (Accessed September 9, 2021).
Bronstein, J. L. (1994). Conditional Outcomes in Mutualistic Interactions. Trends Ecol. Evol. 9, 214–217. doi:10.1016/0169-5347(94)90246-1
Brown, A., Waldheim, L., Landälv, I., Saddler, J., Ebadian, M., McMillan, J., et al. (2020). Advanced Biofuels - Potential for Cost Reduction. Technology Collaboration Programme: Task 41. Berlin: IEA Bioenergy Technology Collaboration Programme.
Burck, J., Hermwille, L., and Bals, C. (2014). The Climate Change Performance index: Background and Methodology. Report. Berlin: Germanwatch.
Burgass, M. J., Halpern, B. S., Nicholson, E., and Milner-Gulland, E. J. (2017). Navigating Uncertainty in Environmental Composite Indicators. Ecol. Indicators 75, 268–278. doi:10.1016/j.ecolind.2016.12.034
Campus, A., and Porcu, M. (2010). Reconsidering the Well-Being: The Happy Planet Index and the Issue of Missing Data. Cagliari: CENTRO RICERCHE ECONOMICHE NORD SUD.
Cobb, C., and Cobb, J. (1994). The Green National Product: A Proposed Index of Sustainable Economic Welfare. Lanham: University Press of America.
Cobb, C., Goodmann, G., and Wackernagel, M. (1999). Why Bigger Isn’t Better : The Genuine Progress Indicator : 1999 Update. San Francisco: Redefining Progress.
Collotta, M., Champagne, P., Tomasoni, G., Alberti, M., Busi, L., and Mabee, W. (2019). Critical Indicators of Sustainability for Biofuels: An Analysis through a Life Cycle Sustainabilty Assessment Perspective. Renew. Sustain. Energ. Rev. 115, 109358. doi:10.1016/j.rser.2019.109358
Day, J. W., Hall, C. A., Yáñez-Arancibia, A., Pimentel, D., Martí, C. I., and Mitsch, W. J. (2009). Ecology in Times of Scarcity. BioScience 59, 321–331. doi:10.1525/bio.2009.59.4.10
De Mazancourt, C., Loreau, M., and Dieckmann, U. (2005). Understanding Mutualism when There Is Adaptation to the Partner. J. Ecol. 93, 305–314. doi:10.1111/j.0022-0477.2004.00952.x
Demirel, Y., and Sandler, S. I. (2002). Thermodynamics and Bioenergetics. Biophys. Chem. 97, 87–111. doi:10.1016/s0301-4622(02)00069-8
Demirel, Y. (2002). “Entropy and Exergy,” in Nonequilibrium Thermodynamics. Editor Y. Demirel (Amsterdam: Elsevier Science), 102–123. doi:10.1016/B978-044450886-7/50005-9
Demirel, Y. (2010). Nonequilibrium Thermodynamics Modeling of Coupled Biochemical Cycles in Living Cells. J. Non-Newtonian Fluid Mech. 165, 953–972. doi:10.1016/j.jnnfm.2010.02.006
Demirel, Y. (2016). Energy: Production, Conversion, Storage, Conservation, and Coupling. 2nd ed. London: Springer.
Demirel, Y. (2018a). “1.22 Biofuels,” in Comprehensive Energy Systems. Editor I. Dincer (Amsterdam: Elsevier), Vol. 1, 875–908. doi:10.1016/B978-0-12-809597-3.00125-5
Demirel, Y. (2018b). Sugar versus Lipid for Sustainable Biofuels. Int. J. Energ. Res 42, 881–884. doi:10.1002/er.3914
Dimijian, G. G. (2000). Evolving Together: The Biology of Symbiosis, Part 1. Baylor Univ. Med. Cent. Proc. 13, 217–226. doi:10.1080/08998280.2000.11927677
Dong, Y., and Hauschild, M. Z. (2017). Indicators for Environmental Sustainability. Proced. CIRP 61, 697–702. doi:10.1016/j.procir.2016.11.173
Edmonds, H., Lovell, J., and Lovell, C. (2017). A New Composite index for Greenhouse Gases: Climate Science Meets Social Science. Resources 6, 62. doi:10.3390/resources6040062
Esty, D., Levy, M., Srebotnjak, T., and Sherbinin, A. (2005). Environmental Sustainability index: Benchmarking National Environmental Stewardship. New Haven: Yale Center for Environmental Law & Policy, 47–60.
Evans, A., Strezov, V., and Evans, T. (2015). Measuring Tools for Quantifying Sustainable Development. EJSD 4, 291–300. doi:10.14207/ejsd.2015.v4n2p291
Fiksel, J., Eason, T., and Frederickson, H. (2012). A Framework for Sustainability Indicators at EPA. Technical Report. Cincinnati: National Risk Management Research Laboratory Office of Research and Development U.S. Environmental Protection Agency.
Flanner, M. G. (2009). Integrating Anthropogenic Heat Flux with Global Climate Models. Geophys. Res. Lett. 36, L02801. doi:10.1029/2008GL036465
Grisolia, G., Fino, D., and Lucia, U. (2020). Thermodynamic Optimisation of the Biofuel Production Based on Mutualism. Energ. Rep. 6, 1561–1571. doi:10.1016/j.egyr.2020.06.014
Gunnarsdottir, I., Davidsdottir, B., Worrell, E., and Sigurgeirsdottir, S. (2020). Review of Indicators for Sustainable Energy Development. Renew. Sustain. Energ. Rev. 133, 110294. doi:10.1016/j.rser.2020.110294
Hacatoglu, K., Dincer, I., and Rosen, M. A. (2015). A New Model to Assess the Environmental Impact and Sustainability of Energy Systems. J. Clean. Prod. 103, 211–218. doi:10.1016/j.jclepro.2014.06.050
Hamilton, K., and Naikal, E. (2014). “Genuine Saving as an Indicator of Sustainability,” in Handbook of Sustainable Development. Editors G. Atkinson, S. Dietz, E. Neumayer, and M. Agarwala (London: Edward Elgar Publishing), 4, 336–347.
Hickel, J. (2020). The Sustainable Development index: Measuring the Ecological Efficiency of Human Development in the Anthropocene. Ecol. Econ. 167, 106331. doi:10.1016/j.ecolecon.2019.05.011
Hsu, A., Lloyd, A., and Emerson, J. W. (2013). What Progress Have We Made since rio? Results from the 2012 Environmental Performance index (Epi) and Pilot Trend Epi. Environ. Sci. Pol. 33, 171–185. doi:10.1016/j.envsci.2013.05.011
International Energy Agency (2021). Tracking Transport 2020. [Dataset]. Available at: https://www.iea.org/reports/tracking-transport-2020 (Accessed September 9, 2021).
Javaid, A., Akbar, A., and Nawaz, S. (2018). A Review on Human Development index. Pakistan J. Humanities Soc. Sci. 6, 357–369. doi:10.52131/pjhss.2018.0603.0052
Karvonen, J., Halder, P., Kangas, J., and Leskinen, P. (2017). Indicators and Tools for Assessing Sustainability Impacts of the forest Bioeconomy. For. Ecosyst. 4, 2. doi:10.1186/s40663-017-0089-8
Labat, J., and Willebald, H. (2019). Do we Need Just One Sustainable Development Indicator? Could Genuine Savings Be that One? IJESNR 16, 555938. doi:10.19080/ijesnr.2019.16.555938
Lin, D., Hanscom, L., Murthy, A., Galli, A., Evans, M., Neill, E., et al. (2018). Ecological Footprint Accounting for Countries: Updates and Results of the National Footprint Accounts, 2012-2018. Resources 7, 58. doi:10.3390/resources7030058
Lindmark, M., Nguyen Thu, H., and Stage, J. (2018). Weak Support for Weak Sustainability: Genuine Savings and Long-Term Wellbeing in Sweden, 1850-2000. Ecol. Econ. 145, 339–345. doi:10.1016/j.ecolecon.2017.11.015
Liu, G., Brown, M., and Casazza, M. (2017). Enhancing the Sustainability Narrative through a Deeper Understanding of Sustainable Development Indicators. Sustainability 9, 1078. doi:10.3390/su9061078
Lucia, U., and Grisolia, G. (2017). Unavailability Percentage as Energy Planning and Economic Choice Parameter. Renew. Sustain. Energ. Rev. 75, 197–204. doi:10.1016/j.rser.2016.10.064
Lucia, U., and Grisolia, G. (2018). Cyanobacteria and Microalgae: Thermoeconomic Considerations in Biofuel Production. Energies 11, 156. doi:10.3390/en11010156
Lucia, U., and Grisolia, G. (2021). The Gouy-Stodola Theorem-From Irreversibility to Sustainability-The Thermodynamic Human Development Index. Sustainability 13, 3995. doi:10.3390/su13073995
Lucia, U., and Grisolia, G. (2021). Irreversible Thermodynamics and Bioeconomy: Toward a Human-Oriented Sustainability. Front. Phys. 9, 659342. doi:10.3389/fphy.2021.659342
Lucia, U., Fino, D., and Grisolia, G. (2020). Thermoeconomic Analysis of Earth System in Relation to Sustainability: a Thermodynamic Analysis of Weather Changes Due to Anthropic Activities. J. Therm. Anal. Calorim. 145, 701–707. in press. doi:10.1007/s10973-020-10006-4
Lucia, U., Fino, D., and Grisolia, G. (2021). A Thermoeconomic Indicator for the Sustainable Development with Social Considerations. Environ. Dev. Sustain.in press. doi:10.1007/s10668-021-01518-6
Lucia, U., Fino, D., and Grisolia, G. (2021). Biofuels from Abandoned Mines: A Starting point for Future Developments. Atti dell’Accademia Peloritana dei Pericolanti 99, SC1. doi:10.1478/AAPP.992SC1
Lucia, U. (2013). Entropy and Exergy in Irreversible Renewable Energy Systems. Renew. Sustain. Energ. Rev. 20, 559–564. doi:10.1016/j.rser.2012.12.017
Lucia, U. (2016). Econophysics and Bio-Chemical Engineering Thermodynamics: The Exergetic Analysis of a Municipality. Phys. A: Stat. Mech. Appl. 462, 421–430. doi:10.1016/j.physa.2016.06.119
Manowska, A., and Nowrot, A. (2019). The Importance of Heat Emission Caused by Global Energy Production in Terms of Climate Impact. Energies 12, 3069. doi:10.3390/en12163069
Martin, B. D., and Schwab, E. (2013). Current Usage of Symbiosis and Associated Terminology. Int. J. Biol. 5, 32–45. doi:10.5539/ijb.v5n1p32
McCool, S. F., and Stankey, G. H. (2004). Indicators of Sustainability: Challenges and Opportunities at the Interface of Science and Policy. Environ. Manage. 33, 294–305. doi:10.1007/s00267-003-0084-4
McGlade, J. (2007). “Foreword: Finding the Right Indicators for Policymaking,” in Sustainablity Indicators: A Scientific Assessment. Editors T. Hák, B. Moldan, and A. Dahl (Washington DC: Island Press).
Moheimani, N. R., McHenry, M. P., de Boer, K., and Bahri, P. A. (2015). Biomass and Biofuels from Microalgae. Cham: Springer.
Moldan, B., and Dahl, A. (2007). “Chapter 1: Challenges to Sustainability Indicators,” in Sustainablity Indicators: A Scientific Assessment. Editors T. Hák, B. Moldan, and A. Dahl (Washington DC: Island Press).
Moldan, B., Janoušková, S., and Hák, T. (2012). How to Understand and Measure Environmental Sustainability: Indicators and Targets. Ecol. Indicators 17, 4–13. doi:10.1016/j.ecolind.2011.04.033
Moran, D. D., Wackernagel, M., Kitzes, J. A., Goldfinger, S. H., and Boutaud, A. (2008). Measuring Sustainable Development - Nation by Nation. Ecol. Econ. 64, 470–474. doi:10.1016/j.ecolecon.2007.08.017
Moriarty, P., and Honnery, D. (2011). Rise and Fall of the Carbon Civilisation. London: Springer-Verlag.
Moriarty, P., and Honnery, D. (2009). “Renewable Energy in a Warming World,” in Energy Policy: Economic Effects, Security Aspects and Environmental Issues. Editor N. B. Jacobs (Hauppauge: Nova Science Publishers).
Munda, G. (2005). "Measuring Sustainability": A Multi-Criterion Framework. Environ. Dev. Sustain. 7, 117–134. doi:10.1007/s10668-003-4713-0
Munzi, S., Cruz, C., and Corrêa, A. (2019). When the Exception Becomes the Rule: An Integrative Approach to Symbiosis. Sci. Total Environ. 672, 855–861. doi:10.1016/j.scitotenv.2019.04.038
Neri, A., Cagno, E., Lepri, M., and Trianni, A. (2021). A Triple Bottom Line Balanced Set of Key Performance Indicators to Measure the Sustainability Performance of Industrial Supply Chains. Sustain. Prod. Consumption 26, 648–691. doi:10.1016/j.spc.2020.12.018
Nourry, M. (2008). Measuring Sustainable Development: Some Empirical Evidence for france from Eight Alternative Indicators. Ecol. Econ. 67, 441–456. doi:10.1016/j.ecolecon.2007.12.019
Oulhen, N., Schulz, B. J., and Carrier, T. J. (2016). English translation of Heinrich Anton de Bary's 1878 speech, 'Die Erscheinung der Symbiose' ('De la symbiose'). Symbiosis 69, 131–139. doi:10.1007/s13199-016-0409-8
Pais, D. F., Afonso, T. L., Marques, A. C., and Fuinhas, J. A. (2019). Are Economic Growth and Sustainable Development Converging? Evidence from the Comparable Genuine Progress Indicator for Organisation for Economic Co-operation and Development Countries. IJEEP 9, 202–213. doi:10.32479/ijeep.7678
Paracer, S., and Ahmadjian, V. (2000). Symbiosis: An Introduction to Biological Associations. 2 edn. New York, USA: Oxford University Press.
Parra Paitan, C., and Verburg, P. (2019). Methods to Assess the Impacts and Indirect Land Use Change Caused by Telecoupled Agricultural Supply Chains: A Review. Sustainability 11, 1162. doi:10.3390/su11041162
M. L. Parry, O. F. Canziani, J. P. Palutikof, P. van der Linden, and C. Hanson (Editors) (2007). Climate Change 2007: Impacts, Adaptation and Vulnerability. Technical Summary (Cambridge: IPPC).
Peacock, K. A. (2011). “Symbiosis in Ecology and Evolution,” in Philosophy of Ecology. Editors K. deLaplante, B. Brown, and K. A. Peacock (Amsterdam: North-Holland), 11, 219–250. Handbook of the Philosophy of Science. doi:10.1016/b978-0-444-51673-2.50009-1
Qiao, H., Zheng, F., Jiang, H., and Dong, K. (2019). The Greenhouse Effect of the Agriculture-Economic Growth-Renewable Energy Nexus: Evidence from G20 Countries. Sci. Total Environ. 671, 722–731. doi:10.1016/j.scitotenv.2019.03.336
Rashid, N., Ryu, A. J., Jeong, K. J., Lee, B., and Chang, Y.-K. (2019). Co-cultivation of Two Freshwater Microalgae Species to Improve Biomass Productivity and Biodiesel Production. Energ. Convers. Manage. 196, 640–648. doi:10.1016/j.enconman.2019.05.106
Rees, W. E. (1992). Ecological Footprints and Appropriated Carrying Capacity: what Urban Economics Leaves Out. Environ. Urbanization 4, 121–130. doi:10.1177/095624789200400212
Rockström, J., Steffen, W., Noone, K., Persson, A., Chapin, F. S., Lambin, E. F., et al. (2009). A Safe Operating Space for Humanity. Nature 461, 472–475. doi:10.1038/461472a
Romano, D., Arcarese, C., Bernetti, A., Caputo, A., Cordella, M., Lauretis, R. D., et al. (2021). Italian Greenhouse Gas Inventory 1990-2019. National Inventory Report 2021. Rome: ISPRA - Istituto Superiore per la Protezione e la Ricerca Ambientale. Technology collaboration programme: Task 41.
Ruan, R., Zhang, Y., Chen, P., Liu, S., Fan, L., Zhou, N., et al. (2019). “Biofuels: Introduction,” in Biofuels: Alternative Feedstocks and Conversion Processes for the Production of Liquid and Gaseous Biofuels. Editors A. Pandey, C. Larroche, C. G. Dussap, E. Gnansounou, S. K. Khanal, and S. Ricke. Second Edition (Academic Press), 3–43. doi:10.1016/B978-0-12-816856-1.00001-4
Sagar, A. D., and Najam, A. (1998). The Human Development index: a Critical Review. Ecol. Econ. 25, 249–264. doi:10.1016/s0921-8009(97)00168-7
Saisana, M. (2014). “Education index,” in Encyclopedia of Quality of Life and Well-Being Research. Editor A. C. Michalos (Dordrecht: Springer), 1816–1819. doi:10.1007/978-94-007-0753-5_840
Scerri, A., and James, P. (2010). Accounting for Sustainability: Combining Qualitative and Quantitative Research in Developing 'indicators' of Sustainability. Int. J. Soc. Res. Methodol. 13, 41–53. doi:10.1080/13645570902864145
Sciubba, E. (2019). Exergy-based Ecological Indicators: From Thermo-Economics to Cumulative Exergy Consumption to Thermo-Ecological Cost and Extended Exergy Accounting. Energy 168, 462–476. doi:10.1016/j.energy.2018.11.101
Shields, D., Šolar, S., and Martin, W. (2002). The Role of Values and Objectives in Communicating Indicators of Sustainability. Ecol. Indicators 2, 149–160. doi:10.1016/S1470-160X(02)00042-0
Stanley, G. D. (2006). Photosymbiosis and the Evolution of Modern Coral Reefs. Science 312, 857–858. doi:10.1126/science.1123701
Steinberger, J. K. (2008). Sustainability Indicators: A Scientific Assessmentedited by Tomas Hak, Bedrich Moldan, and Arther Lyon Dahl. J. Ind. Ecol. 12, 802–804. doi:10.1111/j.1530-9290.2008.00091_2.x
Strezov, V., Evans, A., and Evans, T. J. (2016). Assessment of the Economic, Social and Environmental Dimensions of the Indicators for Sustainable Development. Sust. Dev. 25, 242–253. doi:10.1002/sd.1649
Tausch, A. (2011). Costa rica, Superstar? Some Reflections on the Global Drivers and Bottlenecks of the Happy Planet index. SSRN J. 8, 1–20. doi:10.2139/ssrn.1924130
UNDP Human Development Report Office (1990). Concept and Measurement of Human Development. Human Development Report 1990. New York: UNDP-United Nations Development Programme.
UNDP Human Development Report Office (2010). The Real Wealth of Nations: Pathways to Human Development. Human Development Report 2010. New York: UNDP-United Nations Development Programme.
UNDP Human Development Report Office (2015). Training Material for Producing National Human Development Reports. Occasional Paper. New York: UNDP.
United Nations Development Program (2020). Calculating the Human Development Indices - Graphical Presentation. United Nations: Technical notes HDR.
United Nations General Assembly (1992). Report of the United Nations Conference on Environment and Development - Agenda 21. Report. United Nations.
van de Kerk, G., and Manuel, A. R. (2008). A Comprehensive index for a Sustainable Society: The SSI - the Sustainable Society Index. Ecol. Econ. 66, 228–242. doi:10.1016/j.ecolecon.2008.01.029
Willey, J., Sherwood, L., and Woolverton, C. (2013). Prescott’s Microbiology. 9th edn. New York: McGraw-Hill Education.
Wilson, J., Tyedmers, P., and Pelot, R. (2007). Contrasting and Comparing Sustainable Development Indicator Metrics. Ecol. Indicators 7, 299–314. doi:10.1016/j.ecolind.2006.02.009
World Bank Group (2021). Life Expectancy at Birth, Total (Years). [Dataset]. Available at: https://data.worldbank.org/indicator/SP.DYN.LE00.IN (Accessed March 17, 2021).
Zhao, P., Yu, X., Li, J., Tang, X., and Huang, Z. (2014). Enhancing Lipid Productivity by Co-cultivation of Chlorella Sp. U4341 and Monoraphidium Sp. Fxy-10. J. Biosci. Bioeng. 118, 72–77. doi:10.1016/j.jbiosc.2013.12.014
Latin symbols
GNI Gross national income [$]
HDI Human development index
I Indicator suggested [W h−1]
II Income index
S Entropy production [J K−1]
T Temperature [K]
THDI Thermodynamic human development index
Greek symbols
ηλ Second law inefficiency
Subscript
0 Environment or reference
h Hour
pc Per capita
λ Lost due to irreversibility and dissipation
Keywords: algae, biofuels, cyanobacteria, sustainability, human development index, thermoeconomics
Citation: Lucia U and Grisolia G (2021) Biofuels Analysis Based on the THDI Indicator of Sustainability. Front. Energy Res. 9:794682. doi: 10.3389/fenrg.2021.794682
Received: 26 October 2021; Accepted: 10 November 2021;
Published: 13 December 2021.
Edited by:
Abdul-Sattar Nizami, Government College University, Lahore, PakistanReviewed by:
Keat Teong Lee, Universiti Sains Malaysia (USM), MalaysiaCopyright © 2021 Lucia and Grisolia. This is an open-access article distributed under the terms of the Creative Commons Attribution License (CC BY). The use, distribution or reproduction in other forums is permitted, provided the original author(s) and the copyright owner(s) are credited and that the original publication in this journal is cited, in accordance with accepted academic practice. No use, distribution or reproduction is permitted which does not comply with these terms.
*Correspondence: Umberto Lucia, dW1iZXJ0by5sdWNpYUBwb2xpdG8uaXQ=
Disclaimer: All claims expressed in this article are solely those of the authors and do not necessarily represent those of their affiliated organizations, or those of the publisher, the editors and the reviewers. Any product that may be evaluated in this article or claim that may be made by its manufacturer is not guaranteed or endorsed by the publisher.
Research integrity at Frontiers
Learn more about the work of our research integrity team to safeguard the quality of each article we publish.