- 1Farm Structures Lab, Department of Natural Resources and Agricultural Engineering, Agricultural University of Athens, Athens, Greece
- 2Farm Machine Systems Lab, Department of Natural Resources and Agricultural Engineering, Agricultural University of Athens, Athens, Greece
- 3TCB Avgidis Automation SA, Neos Kosmos, Athens, Greece
The examination of recent developments and future perspectives on smart and solar greenhouse covers is significant for commercial agriculture given that traditional greenhouse relied on external energy sources and fossil fuels to facilitate lighting, heating and forced cooling. The aim of this review article was to examine smart and solar materials covering greenhouse. However, the scope was limited to intelligent PhotoVoltaic (PV) systems, optimization of some material properties including smart covers, heat loading and the use of Internet of Things (IoT) to reduce the cost of operating greenhouse. As such, the following thematic areas were expounded in the research; intelligent PV systems, optimization of the Power Conversion Efficiency (PCE), Panel Generator Factor (PGF) and other material properties, heat loading future outlook and perspectives. The intelligent PV section focused on next-generation IoT and Artificial Neural Networks (ANN) systems for greenhouse automation while the optimization of material parameters emphasized quantum dots, semi-transparent organic solar cells, Pb-based and Pb-based PVs and three dimensional (3D) printing. The evaluation translated to better understanding of the future outlook of the energy-independent greenhouse. Greenhouse fitted with transparent PV roofs are a sustainable alternative given that the energy generated was 100% renewable and economical. Conservative estimates further indicated that the replacement of conventional sources of energy with solar would translate to 40–60% energy cost savings. The economic savings were demonstrated by the Levelized cost of energy. A key constraint regarded the limited commercialization of emerging innovations, including transparent and semitransparent PV modules made of Pb-quantum dots, and amorphous tungsten oxide (WO3) films, with desirable electrochromic properties such as reversible color changes. In addition to intelligent energy harvesting, smart IoT-based materials embedded with thermal, humidity, and water sensors improved thermal regulation, frost mitigation and prevention, and the management of pests and disease. In turn, this translated to lower post-harvest losses and better yields and revenues.
1 Introduction
The review paper presents recent developments and future perspectives of smart and solar greenhouse covers. The novel applications of glass/polymers/films with customized light absorbance and emission properties to regulate solar radiation and control internal and external (greenhouse) temperatures in greenhouse, and generate energy using photovoltaic systems are discussed. The investigation of intelligent PV systems had practical implications on the optimization of PCE and plant growth factor and material properties. For example, Artificial Neural Network (ANN)-based IoT system for frost irrigation management required accurate sensors made using novel materials (Elfrink et al., 2019; Touhami et al., 2020; Gautam et al., 2021). The customization of material properties often involved a tradeoff between PCE, optical properties, plant growth factor, and sustainability (LCOE and transient heat loading). The tradeoffs justified the need for future investments in 3D/4D printing to offset the costs and delays, electrochromic technology, and QDs. Emerging patterns in R&D defined the future perspectives about the integration of fullerenes, LSCs, and PCMs in greenhouse-based PV modules.
Extensive studies have been undertaken on the development of sustainable materials for agricultural applications, including sustainable plastics for agricultural applications (Maraveas, 2020a), biodegradable plastics from agricultural waste (Maraveas, 2020b), sustainable greenhouse covering materials (Maraveas, 2019), and sensors for monitoring the health of agricultural structures (Maraveas and Bartzanas, 2021) and silos (Villacorta et al., 2021). However, there has been an inadequate emphasis on smart solar greenhouse covers for greenhouse that can serve multiple functions, including the regulation of the internal microclimate, reducing the carbon footprint, and energy generation. PV modules can reduce the carbon footprint by 1,735 kg per year (Behzadi and Ahmad, 2020). The potential reduction in carbon footprint attributed to the incorporation of smart greenhouse covering materials would be contingent on the level with which the existing limitations of the materials are addressed.
The integration of PV modules into greenhouse structures can be grouped into three distinct categories, namely; shared structure, sunlight systems shared structures and adjacent (Ravishankar et al., 2020). With the adjacent category, the PV systems are mounted on the adjacent building such as the storage building or the headhouse. Considering the need for land use optimization and judicious use of resources in commercial farming, the adjacent configuration of PV modules and greenhouse structures is considered less appropriate. The case for shared configurations is validated by BIPVs (Deb, 2000; Bjorn et al., 2011; Kwon et al., 2015; Biyik et al., 2017; Joseph et al., 2019). The current discourse on the development of PV modules for greenhouse is fixated on shared configuration, particularly smart covering materials (greenhouse roof structures made of PV modules).
The need to develop novel materials is grounded on the fact that existing PV materials and configurations of solar greenhouse have mixed benefits. Various advantages and disadvantages of PV systems have been identified over the years. For instance, PVs have advantages such as providing ideal conditions for plant growth without the need for external energy input or equipment. On the contrary, PVs have disadvantages such as low heat storage capacity of soil and optimal solar accumulation on the northern wall contributed to significant heat fluxes; this increased the need for active heating to regulate indoor soil and air temperature for optimal vegetation growth (Huang et al., 2021). There are also varied concerns about the cost of commercially available electrochromic glass or polymer dispersed liquid crystals (Baeza et al., 2020). The observations made by Huang et al. (2021) were corroborated by Ravishankar et al. (2020), who demonstrated that energy fluxes in greenhouse predicted the cooling and heating demands. The energy fluxes were a factor of physical elements within a greenhouse, such as the soil, plant, and water, and ambient conditions (solar irradiation, air exchange, convection, conduction, and related factors) (Li et al., 2020; Lunga et al., 2021; Omar et al., 2021) (see Figure 1). The control of the microclimate is vital in light of the energy conversion processes, primarily air exchange, plant transpiration, heat convention and transfer, and heat conduction within a greenhouse (Huang et al., 2020). Based on available evidence, the future of greenhouse production would be defined by smart sensors and neural networks, and thermal models.
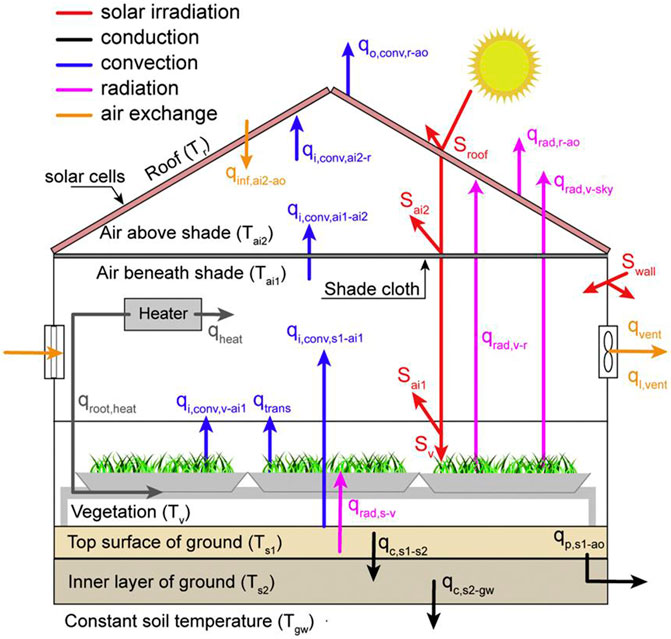
FIGURE 1. Energy fluxes in a greenhouse with semitransparent PV modules (Ravishankar et al., 2020).
The use of PV-based energy to control the internal microclimate would help reduce the energy demand for greenhouse in commercial applications, and by extension, reduce operational costs associated with artificial lighting (see Figure 2) (Shankar et al., 2021). Moretti and Marucci (2019) noted that the control of the internal greenhouse environment was largely dependent on energy supplied from different sources, including electricity from the grid and fossil fuels which was used in powering forced cooling, heating systems and lighting (Moretti and Marucci, 2019; Notte et al., 2020). However, the reliance on fossil fuels contributes to the carbon footprint in farming and elevates global warming. Considering that solar energy is s practical alternative, there is an urgent need to integrate semitransparent building-integrated photovoltaics (BIPV) (Shankar et al., 2021). BIPVs served a dual purpose. On the one hand, they facilitated the optimization of daylight savings (Scognamiglio, 2017; Aguacil et al., 2019; Gholami et al., 2019). On the other hand, they were integral to the generation of clean energy at the site of use.
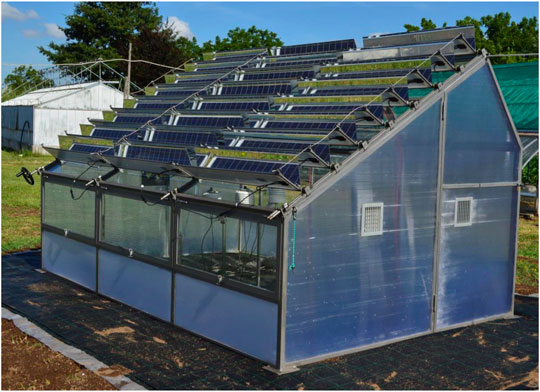
FIGURE 2. Prototype greenhouse structure fitted with PV panels (Ifeanyi et al., 2021).
Presently, commercial application is impacted by the limited demonstrated use of the materials and the initial investment costs. Mazzaro and Vomiero (2018) noted that BIPV devices could be embedded with LSC for low energy and power needs and high aesthetical requirements. The proposition is based on the low PCE of LSCs. Market estimates provided by ClearVue (2021) affirmed that the payback period for green energy was short. The duration was as short as 1 year in advanced economies such as the US. The short payback period coupled with the long-life span (>25 years) improved the appeal of semitransparent PV modules for greenhouse covering (Li et al., 2017; Aroca-delgado et al., 2019). In contrast to previous literature, certain studies have reported extended payback duration for the lifecycle cost of energy, weather-dependent energy production, difficulties in retrofitting PV panels with existing greenhouse structures, and material-related constraints (Moretti and Marucci, 2019; Notte et al., 2020; Renewable Energy Hub, 2020; Ludin et al., 2021). The challenges would be resolved over time, given that emerging PV technologies have increased exponentially.
Potential solutions include active solar heat storage release systems (Castañeda-Miranda and Castaño-Meneses, 2020b) and the BIPVs with low-voltage DC distribution systems. The former system regulates the temperatures in greenhouse through the storage of solar energy in water tanks, while the latter facilitates daytime light-harvesting and energy saving. The energy is then used to raise the ambient temperatures during cold winter nights; this does not resolve the crop yield issue. PV-embedded greenhouse structures were ideal for select crops such as off-season vegetables, mushrooms, tea tree, celery, broccoli, and tomatoes due to the different light requirements (Li et al., 2017); this means that plant-specific PAR requirements should be taken into account in the choice of smart greenhouse covering materials (Friman et al., 2019). A reduction in crop yield (<25%) among high light demanding plants (tomato, cucumber, sweet pepper) was reported in greenhouse fitted with PV modules (Cossu et al., 2020). Since low yields translated to lesser profits, PV modules on greenhouse are most suitable for low light demanding floricultural species.
The merits of the PV-based energy storage system in water proposed by Castañeda-Miranda and Castaño-Meneses, (2020b) are comparable to Camarinha-Matos et al., 2016 findings. However, in the latter case, the quantity of energy stored was dependent on the “operational restrictions and limits of the electricity production and water storage systems” (Camarinha-Matos et al., 2016, 381). The challenge could be offset by Water Pumping Storage Systems (WPSS), which store the energy surplus and regulate system electricity production during low load periods. The stored energy is channeled back during the high load periods (Nefedov and Vyatkin, 2016). An efficient WPSS system integrated with smart technology might help minimize the challenges associated with thermal energy. On the downside, most innovations do not address the photo-voltage losses in semitransparent greenhouse structures must be addressed (Xia et al., 2019); this remains a key barrier to the growth of the semitransparent PV market for commercial agriculture.
Most PV materials for greenhouse covering are unsuitable for commercial use due to the low PCE and plant conversion factor, reduction of the PAR, cost, UV degradation, lack of scalable synthetic methods, and ecological toxicity; this means there was an urgent need to develop new materials and optimize the properties of existing properties through electrochromic technologies, 3D/4D printing, replacement of traditional DSSC with wavelength-selective semitransparent dye-sensitized solar cells, integration of QDs to improve the PCE; this explain why the optimization of existing materials was reviewed under Sections 4, 5. Even though customization of DSSCs, organic PVs, Pb-QDs, amorphous tungsten oxide (WO3) film’s optical and electrical properties would translate to significant improvement in performance, amorphous tungsten oxide (WO3) films, the future outlook would be defined by a narrow class of materials, particularly carbon allotropes (graphene, and fullerenes), PCMs, and LSCs. The drawbacks attributed to LCOE and solar radiation variability could be offset by Polymer Electrolyte Membrane (PEM) fuel cell stacks, electrolyzer bank and solar photovoltaic, double skin facades (DSF) and IoT materials. In brief, each section contributed to the understanding of the present state of smart greenhouse covering materials and future prospects.
2 Novelty of the Research and Paper Organisation
The novelty of the current research paper stems from the fact that it provides a comprehensive and elaborate discussion on the development of smart and solar greenhouse covers including existent limitations and potential advantages that can enhance commercial agriculture. To the best of the researcher’s knowledge, ther lacks studies which provide elaborate review on the recent developments and future perspectives of smart and solar greenhouse covers. In addition, the current research is unique as it provides a balanced review of the advantages and shortcomings of adopting smart greenhouse covers. To further underscore the novelty, the paper is structured into seven sections in order to enhance the flow of ideas. Section 1, the introduction, provides background information on the current research problem. In particular, the challenges of energy fluxes in greenhouses and the inefficiency of relying on fossil fuels are highlighted. Section 2 outlines the research structure and novelty while Section 3 delineates the methodology. In Section 4, a discussion on the intelligent PV systems for greenhouses is undertaken where technologies such as IoT-based materials as well as ANNs and sensors are examined. Section 5 further elaborates on Approaches to optimize PCE and PCF including the use of Pb-QD PV Systems and organic transparent PV systems. In Section 6, the future outlook of smart greenhouse covers is undertaken, whereby, LSCs, PCMs and barriers challenging their adoption are examined. The final section outlines the conclusions from the research and avenues of future research. The flow of ideas is identified from the adopted organisation as the research first introduces the current challenges with use of fossil fuels and thereafter, furthers the discussion to examine the benefits and shortcomings of a variety of solutions; intelligent PV systems, PCE and PCF systems, and the future innovations that include PCMs and LSCs. As a result, the reader achieves a balanced understanding of the research area.
3 Methodology
To facilitate development of the review paper, all data was extracted from published articles sourced from databases such as Science Direct, Scopus, MDPI, and Frontiers. In order to enhance the validity and reliability of the findings, only peer-reviewed articles were considered. Additionally, an inclusion and exclusion criteria was developed to guide the selection of the different articles. Table 1 summarizes the inclusion and exclusion criteria adhered to.
As detailed in Table 1, the literature survey was delimited to focus on recent developments and future perspectives pertaining to smart and solar greenhouse covers. The peer-reviewed articles were selected from databases such as Science Direct, Scopus, MDPI and Frontiers by using keywords such as smart, solar, greenhouse covers, developments and future perspectives. The application of the inclusion and exclusion criteria began with the abstract screening followed by the full-text review which ensured that the articles selected were aligned to the scope of the study. The research also focused mainly on English-published articles in order to avoid allocating more time to translation. Similarly, the publication period was set as 15 years in order to gain comprehensive insights on how smart solar greenhouse cover technology has evolved over the years.
4 Intelligent PV Systems for Greenhouse
The development of smart materials for greenhouse is critical to the regulation of radiation and heat within greenhouse structures. Greenhouse structures are subjected to atmospheric long-wave radiation, ground-reflectance radiation, scattered radiation, and beam radiation, collectively referred to as diffused radiation (Huang et al., 2020). Traditionally, optical properties, spectral radiation, and greenhouse microclimate were regulated through the customization of the cladding materials, anti-reflectance coatings (made of TEOS and other materials) (Dey and Naughton, 2019), coating materials, and transparent materials (Huang et al., 2020). Presently, research interest has been directed towards self-sustaining cover materials made of PV modules, sensors, and IoT for microclimate regulation and energy-independent greenhouse structures.
4.1 IoT-Based Materials and Intelligent Control of Microclimate
IoT-based materials, which refer to devices embedded with sensors to share data with the environment, are integral to intelligent regulation of the greenhouse microclimate and adaptable energy management based on the demand-response and time of use (Salerno et al., 2021); this might translate to significant cost savings given that consumption of electricity is optimized when there is less pressure on the grid. Considering the need to transition from traditional grid-based energy sources to renewables such as PV modules (Darwish et al., 2013; Chen et al., 2019; Shankar et al., 2021), the focus is biased towards IoT-based materials for photovoltaic systems. PVs would be part of the future smart thermal grid (featuring IoT) that would complement the existing electricity supply chain (Yang et al., 2017). Notable progress has been made in the recent past in the development of the next generation of IoT materials for PVs. For example, He and Yao (2007) synthesized tungsten PV materials through cathodic polarization—this facilitated the extension of the photo-response duration for the UV to visible light.
Doping tungsten with metal oxide semiconductors translated to improvements in absorption prior to and after coloration, while hybridization with organic amines resulted in greater photo chromatic reversibility. The observations made by He and Yao (2017) were in line with (Gesheva et al., 2012), who demonstrated the superior function of WO3–MoO3 has electrochromic materials for smart windows. Tungsten oxide was preferred based on its superior electrochromic performance, while MoO3 had a high growth rate. The attainment of the desired properties was contingent on the selection of the most appropriate synthesis method—in this case, APCVD (Gesheva et al., 2012). The progress documented by He and Yao (2007) was corroborated by Ifeanyi et al. (2021), who documented the superior performance of copper–tungsten oxide materials in photovoltaics. In the latter study, it was noted that the Cu2WSe4 was a practical alternative to the traditional sputtering techniques and exhibited Schottky diode–like optical behaviors, which are appropriate for PV applications (Ifeanyi et al., 2021). The comparative performance of tungsten oxide versus other materials was presented in Table 2.
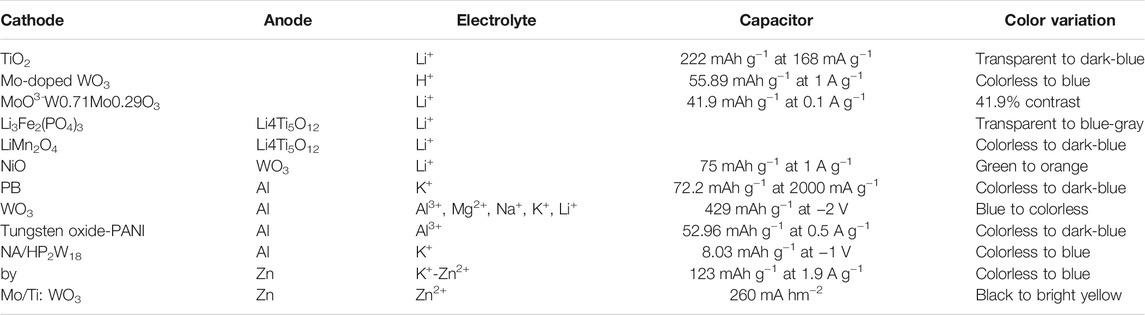
TABLE 2. Relationship between color variations, capacitance, and cathode and anode materials for PVs (Wang et al., 2020).
The higher capacitance of WO3 (429 mAh g−1 at −2 V) and Pb (2,000 mA g−1) affirm the suitability of Pb and W in the development of smart energy harvesting systems for greenhouse (Wang et al., 2020). The comparative assessment of the performance of the metal complexes and oxides highlights the unique merits of these materials vis-à-vis conducting polymers, and Triphenylamine-based polymers, plasmonic nanocrystals (Yang et al., 2018), small organic molecules, carbon materials, lithium titanate, and covalent organic frameworks. The inclusion of small organic molecules such as Fluorescent Poly (methylmethacrylate) films improved the optical properties of coumarin dyes in thin-film solar concentrators.
In contrast with Wang et al. (2020) research, Niu et al. (2021) noted that metal complexes such as phthalocyanines, porphyrins, and polypyridine complexes had rich absorption spectra (UV to IR), and well-defined redox reactions, and better performance relative to metal oxides. The absorption spectra are dependent on the metal-to-metal charge transfer (MMCT) transition and metal-to-ligand charge transfer (MLCT) in the visible and IR regions. The phenomena are also dependent on the chemical properties of the complexes; for example, π radicals augment intramolecular charge transfer in the near-infrared region. The unique capabilities afforded by metal oxides and metal complexes have mixed benefits on IoT devices for smart greenhouse structures. On the one hand, the promising absorption spectra triggered by metal-to-metal charge transfer (MMCT) transition and metal-to-ligand charge transfer (MLCT), photochromatic reversibility, and Schottky diode–like optical behaviors are critical to the commercialization of semitransparent PV modules embedded on greenhouse structures. On the other hand, issues relating to degradation, cost, and performance over extended periods are yet to be determined. Beyond capacitance, the utility of these materials is further reinforced by the desirable color variations.
Wang et al. (2020) noted that the constraints associated with the low transparency of solar cells in greenhouse and commercial structures could be resolved by nanogenerator-driven electrochromic devices. In contrast to traditional PVs, the latter featured a transparent nanogenerator to capture mechanical energies, which can power electrochromic devices. Alternatively, the color transitions and shading problem could be addressed using fuzzy logic IoT-based shading control system for smart buildings (Chiesa et al., 2020). On the downside, the system is expensive, considering it relies on the data collected by multiple sensors. The integration of multiple sensors introduces new challenges in terms of maintenance, sensor malfunction, and cost.
In the next section, the discussion on using IoT systems is enhanced by considering their application in optimization of the performance of greenhouse PV-energy.
4.2 Application of IoT Systems for Optimised Performance of System Greenhouse-PV-Energy
The employment of IoT in greenhouse to attain precision is critical to the widespread utilization of knowledge-based decision support systems, but practical commercial applications are limited—this is particularly the case for integrated Three-Device Systems and cable-bots, which are currently in the experimental phase. The scenario is anticipated with the successful transition from the research and design phase to the commercialization process. Recently, commendable efforts have been made towards the commercialization of IoT systems such as UAVs, ground stations, and LiDAR for optimized performance (Thomopoulos et al., 2021). The UAVs are integrated with LiDAR, spectral and thermal sensors for efficient monitoring of temperature changes in plants; this information is processed by AI systems to inform ground-based action, including the application of pesticides and fertilizers, irrigation, and other measures.
A fundamental constraint is the limited utility of these technologies in a confined and fixed spaces within greenhouse. The limitations of fixed-point sensors could be linked to the inability to collect adequate temporal and spatial variability data to inform decision-making compared to mobile sensors mounted on UAVs (Thomopoulos et al., 2021). The practical constraints noted by Thomopoulos et al. (2021) were confirmed by Shamshiri et al.’s study (2020, 1), which noted that the “automation of greenhouse environment using simple timer-based actuators or by means of conventional control algorithms that require feedbacks from offline sensors for switching devices are not efficient solutions in large-scale modern greenhouse.” The observations made by Thomopoulos et al. (2021) and Shamshiri et al. (2020) affirm that wireless sensors guided by AI systems were vital for modern greenhouse.
In contrast to the mobile sensors embedded on greenhouse, the AI-based systems offer practical benefits in terms of flexibility, contributing to the reduction of greenhouse energy, improving yield predictability, which is integral to the sustainable production of vegetables and fruits under greenhouse environments; this in line with the previous assessments made by Castañeda-Miranda and Castaño-Meneses (2020b). The utility of IoT in commercial agriculture transcends the monitoring of agricultural structures to encompass other important facets such as intelligent hazard mitigation—fire and smoke sensors guided by AI have been developed and deployed in farms (Aspragathos et al., 2019; Su et al., 2021). From an economic perspective, the economic feasibility of IoT systems in greenhouse was contingent on whether the costs of installation and maintenance were lower and sustainable over the long term.
At present, there is scant information on the economic benefits of the systems over the long term. Various arguments have been made to affirm or reject the commercial viability of intelligent systems and predictive and adaptive control algorithms in greenhouse structures. On the one hand, (Shamshiri et al., 2020) noted that the benefits attributed to greenhouse automation with IoT and smart sensors transcended the costs, given that undertaking manual measurements were often time and cost-intensive. In addition, the manual systems were incapable of accurate climate prediction.
The cost benefits of AI systems in agriculture are also grounded on the unique capabilities of IoT systems. For example, Castañeda-Miranda and Castaño-Meneses (2020b) confirmed that the systems were capable of making accurate and intelligent decisions using uncertain information about the air, temperature, and soil conditions; this was achieved through the exploitation of the learning capacity of neural networks and neuro-fuzzy systems. The case for IoT systems is further reinforced by the low power consumption rates of the wireless sensor networks (Rayhana et al., 2020) and the initial capital outlay. Standard G-IoT systems and smart IoT farm kits cost $123 and $100, respectively (Rayhana et al., 2020). Cumulatively, it would cost about $1,500 to integrate intelligent systems in a greenhouse structure. On the other hand, there are varied concerns about the suitability of IoT systems in certain greenhouse. Following the appraisal of the costs and benefits of IoT systems in greenhouse, the benefits clearly outweigh the challenges; this worldview is further supported by the statistically significant improvements in the physical parameters of plants such as length, diameter, and TSS (see Table 3).
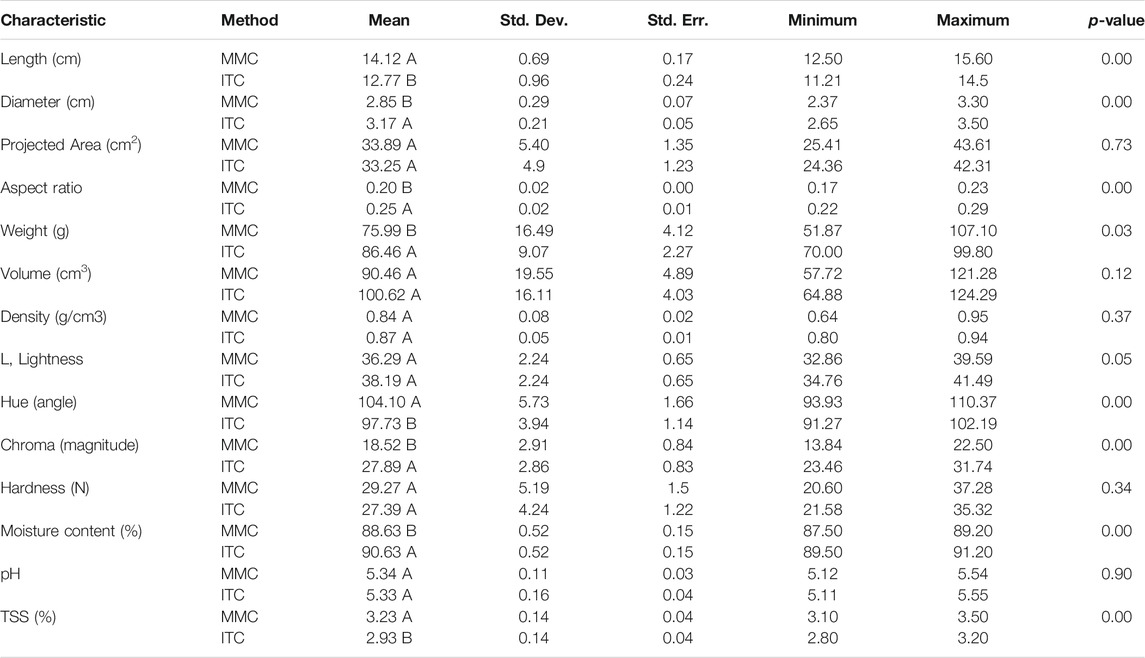
TABLE 3. Improvements in the physical parameters of plants in a greenhouse fitted with an automated water control system of medium moisture control (MMC) and irrigation timing (ITC) intelligent systems (Sagheer et al., 2020).
The improvements in the physical attributes of greenhouse-based plants following the installation of the automated water control systems of medium moisture control and irrigation timing intelligent systems are but a microcosm of the numerous benefits linked to IoT devices and sensors in greenhouse (Sagheer et al., 2020). However, IoT devices have critical limitations in remote monitoring and control and must be paired with cloud computing devices and MQTT or HTTP protocols to overcome the technical constraints (Syafarinda et al., 2018). A comparison of HTTP and MQTT protocols confirmed the utility of the latter. In contrast to the high latency of HTTP protocols, the MQTT is simpler, lighter, and has low bandwidth.
Recent studies have recommended a transition to the more advanced MQTT sensor network, which features better encryption and authentication, translating to better confidentiality and accessibility (Mishra and Kertesz, 2020). Despite the multiple benefits of MQTT protocols enumerated by Syafarinda et al. (2018), and Mishra and Kertesz (2020), there are notable constraints that might impede its utilization in IoT devices in greenhouse, including the inability to attain optimal performance in a multicore environment. The drawbacks make traditional MQTT less suitable for edge-based IoT applications. On a positive note, the challenge could be offset by recent R&D, including edge-based MQTT prototypes and scalable implementations. The limits of MQTT and other systems for PV material would not translate to greater commercialization of PV modules for greenhouse, considering existing materials have low PCE, plant conversion factor and poor optical properties.
In addition to benefits of IoT systems, the use of ANNs and sensors are also examined in the next section.
4.3 ANN and Sensors for Frost Prediction and Mitigation
The regulation of the greenhouse microclimate is integral to optimal plant growth, pest, and disease mitigation using intelligent sensors and neural networks. Castañeda-Miranda and Castaño-Meneses (2020b) documented the successful development of an Artificial Neural Network (ANN)-based IoT system for frost irrigation management. Such a system would help improve crop yield by minimizing crop damage during winter. However, the neural networks and fuzzy logic control systems are prone to errors linked to subjective appraisals, aggregation of conflicting information, redundant and contradictory data. In cases where the data is inaccurate, incomplete, or erroneous, the ANN system modeling yields an inaccurate assessment making potential applications non-deterministic. The limitations associated with ANN systems were partly resolved through the development of alternative systems. In another study, Castañeda-Miranda and Castaño-Meneses (2020b) demonstrated the utility of FES and ANN models in black-frost prediction in greenhouse. In contrast to the previous model, the latter system was appropriate considering it was self-sustaining and integrated with internet services, GSM mobile systems (Huang et al., 2011), and solar panels for energy harvesting.
The integrated fuzzy logic systems predicted the internal greenhouse temperatures and humidity. If the threshold is exceeded, the water pump is automatically activated using the fuzzy expert system. New models based on the Laplace transform have also been proven useful in predicting solar greenhouse air temperature and heating loads (Huang et al., 2021). The automated response is guided by the following variables relative humidity, wind speed, solar radiation, outside temperature, and other factors (Kotsuchibashi, 2020); this means that the performance of each sensor must be optimal, failure to which the system cannot accurately activate the anti-frost water distribution system (Castañeda-Miranda and Castaño-Meneses, 2020a; Zhang Y. et al., 2021). Artificial Neural Networks (ANN) and fuzzy associative memory (FAM) predicted temperature changes with an accuracy of more than 90% (Castañeda-Miranda and Castaño-Meneses, 2020b). ANOVA analyses affirmed the models were reliable in predicting Hard-frost (HF), Severe-frost (SF), Mild-frost (MF), Possible-frost (PF), and no-frost. The two studies affirmed that the reliability of the smart IoT-based materials for greenhouse. However, the reliability was dependent on the temperature and humidity sensors. The linkage between neural networks and autonomous regulation of greenhouse microclimates creates new challenges and opportunities in the growth and development of smart, intelligent systems for commercial agriculture, as demonstrated in the next section.
5 Optimization of the PCE, Plant Conversion Factor, and Other Material Properties in Organic and Non-organic PVs
From a theoretical point of view, the plant conversion factor and PCE of PV-based greenhouse covering materials could be optimized through different mechanisms such as the optimization of the material properties (electrical conductivity, optical characteristics, surface exposure and risk of UV and ambient degradation, and performance under standard conditions through doping, structural and chemical modifications at the nanoscale and surface modifications. Mazzaro and Vomiero (2018) noted that the performance of CdTe/CdSe structures for PVs could be enhanced by the incorporation of type II heterostructured nanorods; this was particularly proven useful in offsetting the self-absorption losses and lower spectral overlap relative to the QD analogs. The high performance is linked to the changes in the self-absorption cross-section from 52 to 0.2% in the single dot CdSe and dot-in-rod structure, respectively (Mazzaro and Vomiero, 2018). Alternatively, nanorods in a PLMA-co-EGDM matrix could be used, despite the high reabsorption losses. In brief, the tunable properties of the materials were integral to the optimization of the PCE and PCF.
Further evidence has demonstrated the need to incorporate 1D nanorods (with high anisotropic luminescence) to augment the performance of the LSCs in real devices. The 1D nanorods might resolve the tradeoff between scalable development of LSC materials for real devices and reduction in optical activity. The utility of 1D nanorods has been demonstrated in organic molecules for PVs, where the inclusion of the nanomaterials helped in the alignment of the dyes in the crystal matrix. In addition, 1D nanorods have been proven effective in redirecting or exploiting diffuse light through light harvesting.
5.1 Pb-QD PV Systems
Drawing from the evidence relating to the Building Integrated Photovoltaic (BIPV) and Electrochromic Glazing (EG) in commercial buildings in Abu Dhabi (Katanbafnasab et al., 2013), smart photovoltaic (PV) materials would help diversify the energy sources in farms, especially in regions with intense solar radiation. Various PV concepts have been developed to advance the commercial application of transparent solar cells, but the extent of commercial application has been limited (Pulli et al., 2020). Preliminary outcomes suggest that silicon-based transparent PV modules had comparable performance to traditional PV materials; this was due to higher isotropic properties of the spherical cells, perpendicular light transmissivity—at 73%, and conversion efficiency of 0.2% (Cossu et al., 2016). However, the optimization of the wavelength-selective thin-film solar cell and non-wavelength selective solar cells remains a critical challenge, as noted by Pulli et al. (2020). Pb-based quantum dots (QDs) have been developed in an attempt to improve photo-conversion efficiency (PCE).
The existing transparent PV module comprises FTO glass, MoO3 film as holes transporting layer (HTL), and TiO2 film as an electron transporting layer (ETL). The synthesized material had a tunable bandgap and multi-exciton generation. The transparent Pb-QDs materials attained a photo-conversion efficiency of 9% (Pulli et al., 2020). On the downside, the optimization of the properties of transparent PV modules only addresses part of the problem considering that other factors must be addressed, including the greenhouse design (ratio of the roof covered with the panels), angle of the incident solar rays, roof panel configuration, seasonal light availability. If the latter conditions are not optimized, the optimal PCE would have negligible benefits on the greenhouse.
Cossu et al. noted there was a 1% reduction in conversion efficiency for over 1% reduction in solar radiation. Other researchers have raised concerns about the viability of Pb-QDs in solar cells due to inadequate stability, loss of performance, and surface oxidation (resulting in the formation of PbO and Pb(OH)2), and ambient degradation. The net effect is loss of performance due to the shrinkage of the effective core size. The problem is anticipated to persist, considering existing technologies are unable to address these constraints considering that surface oxidation is primarily induced by visible and UV light exposure (Albaladejo-Siguan et al., 2021). Mixed data has been presented on the implications of surface oxidation. In contrast to Albaladejo-Siguan et al. (2021), Becker-Koch et al. (2019) argued that the formation of the PbO had beneficial effects, at least in the short-term given that surface oxidation helped to mitigate degradation and pathways for current leakage and molecular recombination, which in turn, translated to a partial improvement in device performance. The contrasting observations made by Albaladejo-Siguan et al. (2021) and Becker-Koch et al. (2019) demonstrate the need for further research and development and long-term monitoring of Pb-based QDs. The drawbacks of the QDs had influenced the development of alternative materials and wavelength-selective technologies for PV greenhouse (see Figure 3).
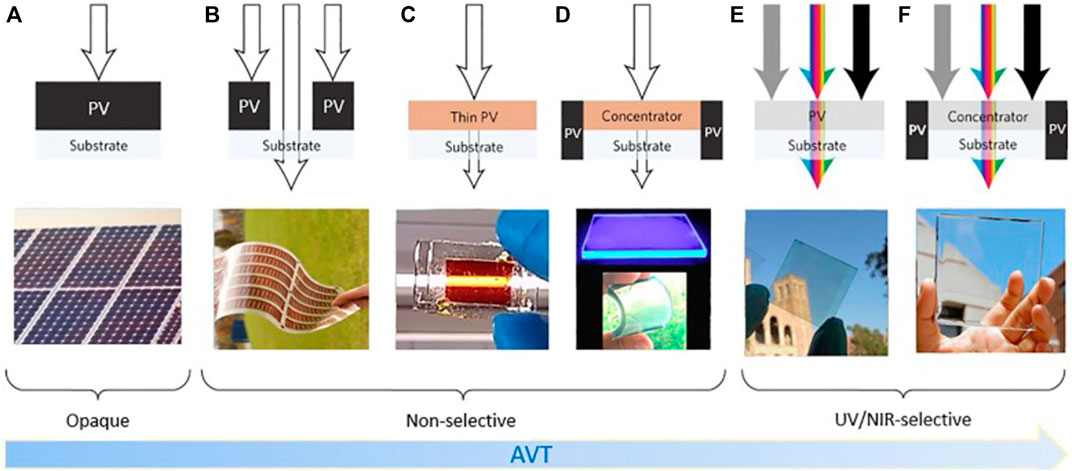
FIGURE 3. Average wavelength-selective technologies for PV greenhouse materials (Pulli et al., 2020).
5.2 Pb-free, Halide Perovskite, and Copper-Doped InP/ZnSe Quantum Dots
The limits of Pb-based QDs motivated recent R&D projects that led to the development of alternative materials such as copper-doped InP/ZnSe QDs and Pb-free materials. Pb Halide Perovskites are a practical alternative to the Pb-QDs due to the high efficiency of 26% and excellent bandgap of about 1.5–1.6 Ev (Becker-Koch et al., 2019). The performance of the Pb-halide perovskite was threefold higher (25.5%) (Albaladejo-Siguan et al., 2021); this contrasted with the wavelength-selective semitransparent dye-sensitized solar cells, which had a PCE of 8% (Wang et al., 2021), and wavelength selective semitransparent polymer solar cell’s 7.7% (Shi et al., 2019). The performance of perovskite materials in solar energy harvesting was also confirmed by Lian et al. (2019) in a study of 2D-perovskite films made via spacer cation-assisted growth. However, the PCE was 14%, which is significantly low compared to the Pb-halide perovskites. The variations in performance could be linked to the in grain and between grain blockages of charge transport in the 2D perovskite film (Lian et al., 2019). A phenomenon that has not been corrected with the preferred alignment and orientation of [Bn − 1MnX3n + 1]2−. However, the organic solar cell’s 17.5% PCE (Wang et al., 2021) could be a suitable alternative given the toxicity of Pb. Eren et al. (2021) study provides a compelling argument about the need to diversify materials for solar concentrators.
The need to develop complementary materials beyond Pb-QDs has also led to the development of luminescent solar concentrators (LSCs) featuring copper-doped InP/ZnSe QDs (Eren et al., 2021) and CdSe QDs (Albaladejo-Siguan et al., 2021). Similar to the DSSCs, the LSCs are cost-effective and simple solar energy harvesting devices. However, in contrast to the Pb-QDs, these materials were environmentally benign; this means they posed the least ecological impact to the environment compared to Pb-QDs (Eren et al., 2021). On the downside, the unique benefits were offset by reabsorption losses, and correction of the anomaly required expensive stokes shift engineering. The application of stokes shift engineering in commercial production was criticized by Mazzaro and Vomiero (2018), who claimed it had limited effectiveness in improving the performance of CdSe-Qds. The poor performance was attributed to the elongated structure’s exciton confinement effects.
The challenges enumerated by Albaladejo-Siguan et al. (2021), Eren et al. (2021), Becker-Koch et al. (2019) following the synthesis of Pb-Free, Halide Perovskite, and Cu-InP/ZnSe QDs illustrates that the elimination of Pb only addresses part of the problem. The shelf-life of CdSe-QDs was unsatisfactory. Longer storage led to poor performance—up to an 80% reduction in PCE within 72 h (Albaladejo-Siguan et al., 2021). A similar phenomenon was noted when the QDs were subjected to continuous illumination, which decreased the PCE by 25% after 200 irradiations. Other stress factors that impair the performance of QDs are presented in Figure 4.
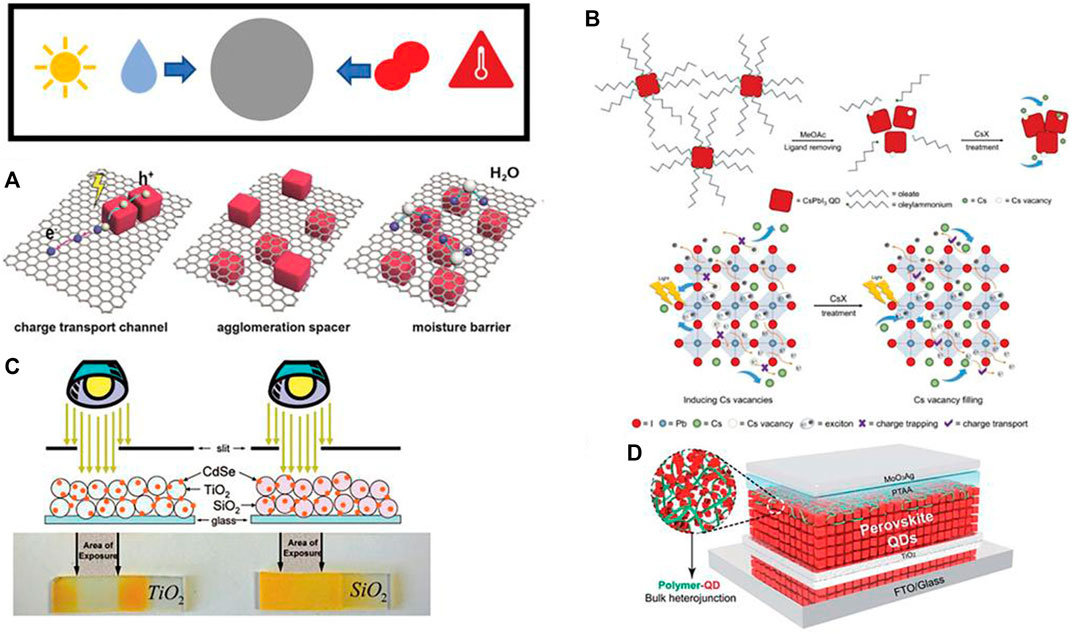
FIGURE 4. Stress factors that impair the performance of QDs and interventions including; (A) Prevention of moisture accumulation using a thin layer of graphene, (B) the use of Ce ions for passivating the QDs, (C) QD film degradation using electron transport layers using as CdSe, TiO2, and SiO2 (D) Organic/polymer QD layer to protect the QDs from oxidation (Albaladejo-Siguan et al., 2021).
The complexity of integrating different interventions such as thin layers of graphene, Ce ions for passivating the QDs, CdSe, TiO2, and SiO2 electron transport layers and organic/polymer QD layer to protect the QDs from oxidation coupled with the environmental risk factors (Albaladejo-Siguan et al., 2021) validate the need for the utilization of diverse materials. On the downside, diversification of existing materials would require significant investment in analytical chemistry and nanotechnology R&D beyond QDs, including the investigation of differential light scattering of nanoparticles (Wyatt, 2021), to gain a better understanding of the nanoparticle structure and function, aggregation processes, macromolecular interactions, and molar mass distribution processes, that impact the PCE of solar cells. The observations made by Wyatt (2021) were in agreement with (Lian et al., 2019), who demonstrated the utility of dynamic and differential light scattering in improving the performance of solar cells. The fabrication of 2D Ruddlesden–Popper perovskite-based solar cells (PVSCs) was augmented by dynamic light scattering (DLS) (Bershadskii, 2008; Bhattarai and Wilczura-wachnik, 2015), through the identification of stages with a higher risk of aggregation, especially in the precursor solutions. DLS has proven to be a reliable technique for customizing the optical properties of PV materials (Giglio and Vailati, 1995). The aggregation and nucleation challenge was partly offset by the application of the phenyl ammonium SSC. The net product was an optimized inverted planar PVSC with a PCE of 14.1% (Lian et al., 2019). The complex synthetic routes coupled with inadequate PCE highlight the need to develop complementary materials, including transparent PV systems for solar cells.
5.3 Organic Transparent PV Systems for Solar Cells
Organic and polymer-based PV transparent materials are a viable alternative to Pb-QDs in greenhouse applications. The observation has been supported by the extended range of applications for these materials and proven effectiveness in previous research studies. Wang et al. (2021) successfully developed high-performance and eco-friendly semitransparent (ST) organic solar cells (OSCs) that were lightweight and transparent, and ideal for greenhouse applications. The conversion efficiency was 17.5%, which is significantly higher compared to Cu2O films, which had a conversion efficiency of 10% under specific conditions (Ifeanyi et al., 2021). The recorded PCE (17%) and the plant growth factor (24.7%) represented the best performance among existing OSCs; this is true given that previous materials often had a higher crop growth factor and a lower PCE. For instance, wavelength selective semitransparent polymer solar cell (ST-PSC) developed by Shi et al. (2019) had a PCE of 7.7% and a plant growth factor of 24.8% (Shi et al., 2019). The superior conversion efficiency of materials made by Wang et al. (2021) could be linked to the facile and scalable synthetic routes. The ST-OCS was developed using novel quaternary blends and green fabrication techniques, which are suitable for greenhouse. Nonetheless, commercial application of the materials was contingent on the resolution of existing constraints, including insufficient performance parameters.
The utility of OSCs demonstrated by Wang et al. (2021) was confirmed by Chalkias et al. (2021), who synthesized low-cost organic dyes with tailored blue-green light absorption (wavelength-selective semitransparent dye-sensitized solar cells DSSCs); this was critical in increasing the level of transparency in the active photosynthetic radiation for greenhouse. However, in contrast to the ST-OSCs developed by Wang et al. (2021), Chalkias et al. (2021) wavelength-selective semitransparent dye-sensitized solar cells (DSSCs) attained a PCE of 8%, which is unsatisfactory compared to the 17.5% PCE (Wang et al., 2021). However, the PCE recorded by Chalkias et al. (2021) was slightly higher relative to the wavelength selective semitransparent polymer solar cell (ST-PSC) developed by Shi et al. (2019). The latter materials attained a PCE of 7.7% (Shi et al., 2019), exclusive of high electron mobility fullerene acceptor. The addition of the fullerene blends translated to a notable improvement in the PCE from 7.7 to 10% (Shi et al., 2019). On the downside, the binary blend (PC71BM into J52: IEICO-4F) with a PCE of 7.7% was highly preferred considering it possessed suitable open-circuit voltage, charge extraction, and trap-assisted recombination.
The PCE variability could be linked to the synthetic routes. In the latter case, Chalkias et al. (2021) relied on a transparent iodine-free electrolyte coupled with a low-cost tri-phenyl-amine-based dye as a replacement to the conventional expensive ruthenium-based dyes to achieve the desired level of optical transparency. However, it could be argued that the poor performance was complemented by the higher quantum efficiency of 85% in the blue and green sections of the visible region of the electromagnetic spectrum and the plant growth factor of 35% (Chalkias et al., 2021), which is slightly higher than the 24% documented by Wang et al. (2021). The variable outcomes demonstrate that the customization of the ST-OSC properties often involves a tradeoff between the plant/crop growth factor, PCE, and quantum efficiency in the visible spectrum. Following the appraisal of the existing body of knowledge on the subject, it was deduced that the high-performance and eco-friendly semitransparent (ST) organic solar cells (OSCs) developed by Wang et al. (2021) offered the best practical and commercially viable materials for cladding greenhouse roofs; this means that spectral engineering-related improvements in quantum efficiency were less beneficial compared to the maximization of the PCE in the development of organic PV modules for energy-autonomous greenhouse.
Despite the low performance of wavelength-selective semitransparent dye-sensitized solar cells (DSSCs), emerging innovations in research and development could translate to better PCE and plant conversion factors. The current constraints associated with the existing materials could be addressed through spectral engineering of the absorbance spectrum of the PV modules to attain optimal light-harvesting (PAR transmittance) and matching of the absorption spectra of the photoreceptors (chlorophyll in plants) with the transmission spectra of the ST devices. The optimization of the spectra properties would translate to better solar harvesting and photosynthesis. In the meantime, greenhouse cladding materials made of PV materials would record mixed efficiency in terms of quantum efficiency, PCE, and the plant growth factor, and this would, in turn, impair the commercial uptake of the novel and smart materials for greenhouse structures. The varied performance of the DSSCs, QDs, and friendly semitransparent (ST) organic solar cells (OSCs) in terms of plant growth factor and PCE raises fundamental questions about the commercial viability of transparent greenhouse fitted with PV modules. At present, the R&D innovations might not be commercially viable given the risk of environmental degradation and oxidation.
5.4 Electrochromic Technology for Greenhouse Covers
Electronic materials have a broad range of applications that encompass thermal storage, smart window, military camouflage, and optical fiber telecommunications due to reversible transmittance and/or reflectance within the IR and visible ranges of the electromagnetic spectrum (Niu et al., 2021). Recently, the technology has found new applications in greenhouse, where reversible color changes are critical to energy storage, thermal regulation, energy harvesting, and sensing. However, the application of the technology depends on the nature of the electrochemical stimuli and materials. Organic small molecules, metal complexes, carbon materials, lithium titanate, plasmonic nanocrystals, covalent organic framework, conducting polymers, and Triphenylamine-based polymers have different electrochromic properties and wavelength ranges (Niu et al., 2021); this partly explains why a greater emphasis on metal oxides particularly, tungsten oxides due to their commendable chemical stability, electrochemical reversibility, light modulation ranges, coloration efficiency, and commercially scalable and facile synthetic routes.
Amorphous tungsten oxide (WO3) films were proven to possess electrochromic properties defined by reversible color changes through complex redox reactions in the 1960s (Wang et al., 2020); this, in turn, influenced the reflectance, transmittance, and absorbance. The phenomenon is triggered following exposure to external current or voltage (Wang et al., 2020). The electrochromic technology has practical applications in smart greenhouse, particularly in the development of smart, intelligent materials for energy storage, thermal regulation, energy harvesting, and sensing (see Figure 5). Despite the extensive research conducted on tungsten materials, there are limited commercial applications in thin-film solar cells compared to organic PV cells, copper indium gallium selenide, cadmium telluride, and amorphous silicon (Renewable Energy Hub, 2020). However, the scenario is anticipated to change with the recent progress made in energy-band engineering of semiconductor materials that resulted in the improvement of tungsten photo-chromism.
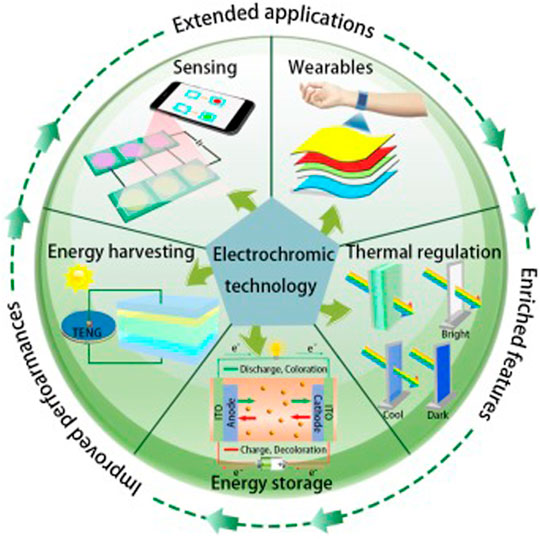
FIGURE 5. Potential applications of electrochromic technology in greenhouse (Wang et al., 2020).
From a purely commercial standpoint, vanadium oxide (VOx) is a practical alternative to WO3; consider why. First, VOx exhibits unique phase change behaviors when exposed to IR energy. The semiconductor behavior of the oxide is diminished after IR-induced phase changes. The metallic phase of the material reflects IR energy, while the semiconductor phase of the material is transparent to IR. The periodical transition in the optical properties epitomizes the thermochromic behavior of VOx. A plausible area of application of VOx is in the reduction of the visible light transparency of greenhouse covering materials (Makableh et al., 2021). Second, there are proven facile and scalable methods for customizing the thermochromic properties of greenhouse cover materials, such as the design of new core-shell structures, periodical patterns, micro-roughness modulation, and porosity control (Makableh et al., 2021). The case for VOx materials documented by Makableh et al. (2021) was corroborated by Kamalisarvestani et al. (2020), who linked the superior performance of VOx to the different synthetic routes.
5.5 Organic PV
Ravishankar et al. (2020) noted that the use of semitransparent organic PVs was integral to the realization of the net-zero energy greenhouse. The development of smart PV materials for solar greenhouse is validated by the following considerations. Shared configurations have been proven ideal in the design of greenhouse structures due to the immense economic advantages in terms of the balance of system savings. Opaque solar modules compete with plants for the light used in photosynthesis; this creates new challenges in terms of solar energy absorption and crop yield—crop yield is dependent on the rate of photosynthesis. Additionally, the opaque PV modules reduce the intensity of solar radiation available to plants and interfere with the greenhouse microclimate temperature (Aroca-delgado et al., 2019). The drawbacks of opaque PV modules reinforces the need for transparent PV modules for greenhouse structures.
Alternative measures proposed focus on overcoming the photosynthetically active radiation (PAR) spectrum (400–700 nm) barrier to ensure that PV modules do not prevent sunlight generation (Ravishankar et al., 2020). The new PV systems are designed in a manner that optimizes power generation using other spectrums of solar radiation. Technological breakthroughs have been achieved using wavelength-selective optics that focus sunlight on the opaque PV modules. The function of the wavelength-selective optics can be optimized through the integration of luminescent solar concentrators (Moretti and Marucci, 2019; Pulli et al., 2020; Eren et al., 2021). The shortcomings of opaque PV modules and the distinctive advantages of transparent/semitransparent materials provide a compelling justification for the development of semitransparent wavelength-selective photovoltaics for power generation in greenhouse.
Even though there is a compelling case for wavelength-selective focusing lenses, certain drawbacks must be resolved, including the need to develop complex greenhouse structures that are expensive to maintain, requirements for solar tracking, and configurations that focus the primary direct sunlight (Ravishankar et al., 2020). In reality, the enumerated challenges could be offset through the development of smart greenhouse covering materials embedded with luminescent solar concentrators. In contrast to the opaque PV materials, the performance of the luminescent solar concentrators is grounded on the following basic principle; the luminescent species are responsible for solar energy harvesting, that is latter channeled to a transparent waveguide, before being re-emitted at a different wavelength; this is achieved in all different directions. The emitted photons are blocked by the internal reflection due to the refractive index within a greenhouse structure (Pulli et al., 2020). The process contributes to the redirection of the photons towards the waveguide edges, where the PV unit converts the radiation to electrical power. The power efficiency of luminescent solar concentrators is calculated using the following formula in Eq. (1) (Pulli et al., 2020); nLSC denotes the power efficiency of the solar concentrators, while nopt and n* represent the optical efficiency (ratio of impimping photons on the active area and photons transported out of the LSC edge) and the PCE under monochromatic irradiation, respectively (Pulli et al., 2020). The optical efficiency of other LSC configurations is presented in Table 3.
A key barrier to the widespread use of solar concentrators was the low power conversion efficiency. The observations made by Ravishankar et al. (2020) were corroborated by Eren et al. (2021), who synthesized copper-doped InP/ZnSe quantum dots for luminescent solar concentrators with an extremely lower power conversion efficiency of 3.4% and external quantum efficiency levels (EQE) of 5.9% (Eren et al., 2021). The PCEs of the materials was significantly low relative to Semitransparent (ST) organic solar cells with a PCE of 17%, Cu2O films (PCE—10%) (Ifeanyi et al., 2021), semitransparent polymer solar cells (PCE—7.7%) (Shi et al., 2019), Pb-halide perovskite (PCE—25.5%) (Albaladejo-Siguan et al., 2021). The low PCE offsets the unique benefits associated with the LSCs. The observation is supported by a broad assessment of the optical efficiency, absorption, and PCE of different LSC configurations in Table 4.
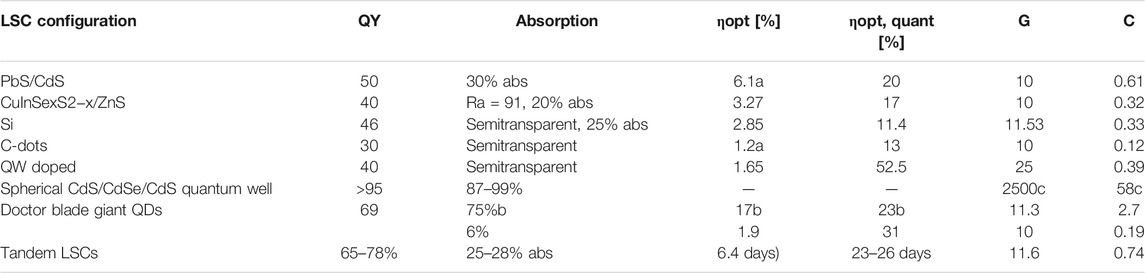
TABLE 4. Properties of different LSC configurations (Mazzaro and Vomiero, 2018). Configuration—outer section of the greenhouse is covered by PV cells while the others are fitted with reflective mirrors. G calculated at a single wavelength; micro-PV cell embedded measured resonant optical cavity configuration.
Even though there is broad consensus in the scholarly literature on the poor energy and power capacity of LSCs (Albaladejo-Siguan et al., 2021; Eren et al., 2021), there are varied opinions on the potential areas of commercial application of the LSCs. Mazzaro and Vomiero (2018) note that LSC nanomaterials with tunable optical properties were ideal for use in BIPV devices. Nonetheless, there is minimal evidence to support the integration of Si-based and alternative BIPVs in greenhouse covering structures (Chan, 2019). The existing body of knowledge is fixated on standard buildings and not greenhouse. Drawing from experimental evidence, BIPVs could prove useful in greenhouse applications, but certain parameters must be optimized, including the air gap between the greenhouse covering materials, and the PV modules, ventilation, slope, orientation of the solar collector surface, quantity of incident radiation and location (Agathokleous et al., 2018). The drawbacks of LSCs and opaque PV modules incentivized researchers to develop semitransparent organic solar cells (Ravishankar et al., 2020). Organic PV materials are attractive alternatives given that they feature a tunable active layer absorption spectrum that can match the different material selections. In addition, the semitransparent organic materials can be integrated into the existing greenhouse structures, given they are thin and lightweight. The unique properties help to reduce construction costs.
5.5.1 Transient Heat Loading in Solar Greenhouse
The optimal energy benefits associated with advances in DSSCs, QDs, and friendly semitransparent (ST) organic solar cells (OSCs) and other materials for PV modules depend on the transient heat loading in solar greenhouse. Temperature variations and materials used in the construction of greenhouse materials contribute to significant variations in the heating loads and heat transfer loss. Despite the benefits associated with semitransparent solar materials, (Huang et al., 2021) argued that semitransparent materials for greenhouse have poor thermal inertia and high heat coefficients that make the microclimate to be extremely vulnerable to external environmental/meteorological parameters (Friman-peretz et al., 2021); this means the energy-related benefits associated with the installation of transparent PV modules, and transparent nanogenerators to capture the mechanical energies, through optimized fuzzy logic IoT (Chiesa et al., 2020) could be constrained by the changes in the greenhouse microclimate linked to the poor thermal inertia and high heat coefficients. Vanadium oxide is an ideal material for thermal insulation of greenhouse due to its unique optical properties (100% reversible phase changes/transformation) and demonstrated applications in real-life smart-window applications (Makableh et al., 2021). However, the optimal performance of the material is contingent on the incorporation of other metal oxides (ZnO, TiO2, ZrO2, SnO2, CeO2, WO3 and Cr2O3) to achieve greater anti-reflection.
5.6 3D and 4D Printing of Smart PVs
The four-dimensional printing of greenhouse covering materials is critical to the mass production of affordable materials with PV modules, given that objects with high levels of geometric complexity can be customized within a short duration and at an affordable cost (González-Henríquez et al., 2019). The need for on-time and on-demand customization of smart-materials for greenhouse is critical considering the absorption and concentration of solar radiation was contingent on the size of the absorber (light in 6 and 8 cm focus) (Korecko et al., 2010). However, the utilization of 3D and 4D printing techniques in the development of greenhouse covering materials is still in its infancy (González-Henríquez et al., 2019; Maraveas et al., 2021). Promising 3D and 4D printing techniques include materials extrusion, powder bed fusion, material jetting, binder jetting, vat photo-polymerization, and sheet lamination. The choice of 3D and 4D printing processes for greenhouse covering materials is influenced by a wide array of factors, including the cost of the process and desired application (González-Henríquez et al., 2019). The real-life application of 4D printing in agriculture is at its infancy, given that initial attempts were directed towards biomedical applications. The exploitation of the benefits afforded by 4D printed materials depends on the LCA and LCOE of materials, mitigation of transient loading coupled with the development of innovative solutions to counter the variable solar irradiation caused by weather patterns, weather impact on PV performance—dust and sand accumulation reduce the efficiency of PV modules.
6 Future Outlook and Perspectives
The future outlook of smart and solar greenhouse covers for solar panels would be dependent on the rate of R&D and the commercialization of developed technologies and cost-benefits of novel materials. At present, researchers are still contending with diverse challenges, ranging from the variable PCE of different PV materials, and there is an urgent need to develop advanced and smart greenhouse covering materials made of PV modules with optimal PCE and the plant conversion factor. The underlying industry needs for new materials are validated by the cost benefits of existing materials.
Semitransparent (ST) organic solar cells have higher PCE relative to Cu2O films (17 versus 10%) (Ifeanyi et al., 2021). However, this was not the case for semitransparent polymer solar cells (ST-PSC), which had a PCE of 7.7% (Shi et al., 2019). The evidence demonstrates that a transition from metal oxides to organic materials does not automatically translate to significant improvements in PCE. Various options are available to address the constraints associated with the plant growth factor, including the adoption of unique synthetic routes and materials such as novel quaternary blends, fullerenes, and green fabrication techniques (Wang et al., 2021). A greater emphasis is made on the future prospects of fullerene-based PV materials, and PCMs, and energy modelling for smart greenhouse covers, considering their practical benefits and promising applications in offsetting the drawbacks of existing materials. The cost benefits of PCMs, fullerenes and other materials are listed in Table 5 (Mukherjee et al., 2021). Cost, sensitivity to ambient conditions, and environmental degradation were identified as critical barriers to the adoption of novel materials in the comparative analysis.
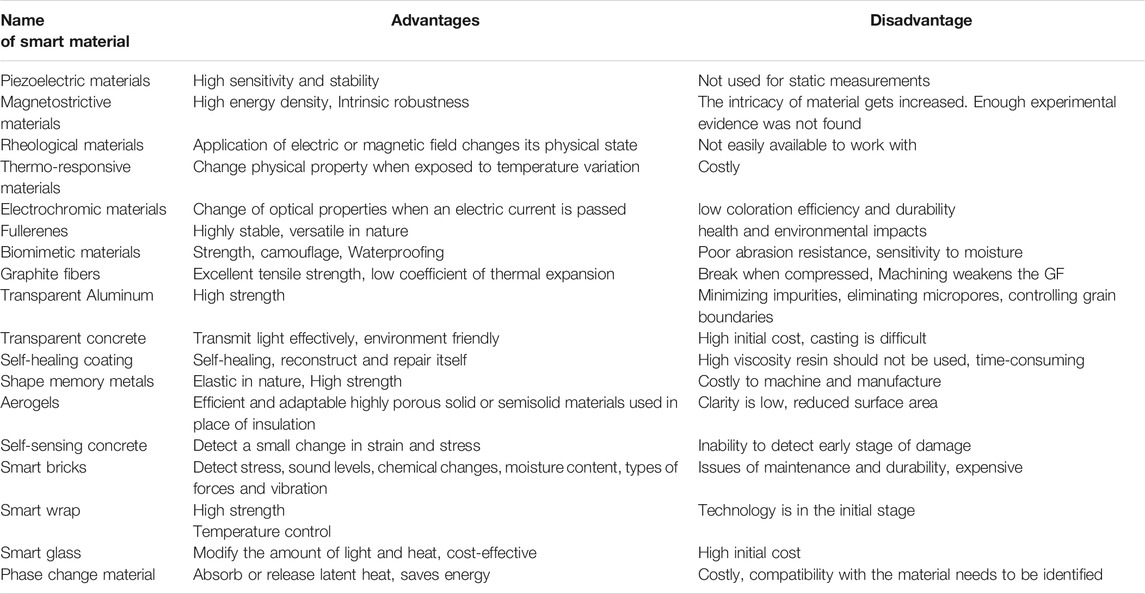
TABLE 5. Cost benefits of materials for smart greenhouse covers (Mukherjee et al., 2021).
6.1 Fullerene-Based, and Graphene-Based PV Materials for Smart Greenhouse Covers
The next generation smart greenhouse and PV modules would be defined by innovations in carbon allotrope-based materials such as fullerenes (Emmott et al., 2015), carbon nanotubes (CNTs) (Ong et al., 2010; Blackburn, 2017; Celik et al., 2017; Ghalandari et al., 2020), and graphene (Saeidi et al., 2020). The proposal is validated by the limits of Pb-based QDs, which had critical shortcomings owing to surface oxidation, ambient degradation, inadequate stability, and loss of performance. The problem was exacerbated by frequent exposure to UV radiation.
Graphene-based QDs had a photoluminescence (PL) quantum yield of 65% due to a significant reduction in reabsorption losses (Saeidi et al., 2020). Apart from the low reabsorption losses reported by Saeidi et al. (2020) synthesized thermotropic hydrogel-graphene oxide molecules with auto-adjust transparency—a desirable characteristic for dual-controlled smart glasses (Chou et al., 2017). The graphene oxide screened sunlight irradiation entering a building and converted photoenergy into thermal energy. The conversion process triggered reversible changes in the optical properties, as the smart glass became opaque to reduce the incident solar radiation. Although graphene-based PV materials possess desirable optical and electrical characteristics, the scalable synthesis of graphene remains a challenge (Ren and Cheng, 2014; Zhong et al., 2015; Deng et al., 2019; Lin et al., 2019). The challenges are anticipated to impact the production of graphene-based PVs.
Similar to graphene, carbon allotrope fullerene is an ideal material for PCE improvement in PV materials Shi et al. (2019), but there are no constraints to the commercial production of fullerenes; this explains the emphasis on fullerene-based PV materials for smart greenhouse covers. In addition to scalable synthesis, the suitability of fullerenes is grounded on the narrow HOMO-LUMO band gap, low reorganization energy, and chemical conjugation (Zhan and Jiang, 2008). The appropriate ratios for fullerene that translate to optimal open-circuit voltage, charge extraction and trap-assisted recombination should be investigated. Alternatively, the case for fullerene-based solar cells can be contested, given that minimal progress had been made over time in translating R&D innovations to the commercial sphere. The poor translation of innovations could be linked to the existing shortcomings.
Initial research into polymer-fullerene solar cells can be traced back to Thompson and Fréchet (2008) research on polymer-fullerene materials. Within that period, Zhan and Jiang (2008) investigated phospholipid-assembled fullerenes for deployment in modular photocurrent generation systems. The latter study demonstrated that the most feasible approach for integrating fullerenes into PV modules was through the development of amphiphilic fullerene C60 derivatives in phospholipids; this approach facilitates the exploitation of the photo-induced charge conversion, HOMO-LUMO charge conversion, and electron/energy transfer.
The outcomes drawn from Thompson and Fréchet (2008), Zhan and Jiang (2008) and Shi et al. (2019) research suggested that fullerenes were appropriate materials due to their charge transfer properties and electron affinity. According to the study, the most effective material at the time was made of polymer-fullerene materials. Despite the promising outcomes in 2008, little progress has been made in the commercialization of these innovations. Recent studies are still exploring practical synthetic routes for fullerene-based solar materials (Kar et al., 2016). On the downside, the material is hydrophobic. The challenges documented in the commercialization of fullerene-based innovations in PV modules was not specific to the material.
The current constraints associated with the existing materials could be addressed through spectral engineering of the absorbance spectrum of the PV modules to attain optimal light-harvesting (PAR transmittance) and matching of the absorption spectra of the photoreceptors (chlorophyll in plants) with the transmission spectra of the ST devices. The optimization of PAR can help improve crop quality and yield (Allardyce et al., 2017). The optimization of the spectra properties would translate to better solar harvesting and photosynthesis. In the meantime, greenhouse cladding materials made of PV materials continue to record mixed efficiency in terms of quantum efficiency, PCE, and the plant growth factor (Trypanagnostopoulos et al., 2017), considering PV modules on greenhouse filters out solar radiation. Until the PAR issue is resolved, the commercial uptake of the novel and smart materials for greenhouse structures will remain limited.
6.2 Phase Change Materials
Greenhouse structures are designed to maximize solar energy absorption for photosynthesis; this creates a new challenge in terms of the maximization of the excess heat stored. Thermal energy storage using heat-storage and heat release systems, phase change materials, solar collectors, and geothermal energy in greenhouse provides a practical approach to address the problem associated with excess heat (Huang et al., 2020). Solar greenhouse have marginal heat resistance, and horticultural plants are significantly sensitive to outdoor fluctuations in temperature and humidity. Phase change materials, which have a demonstrated effectiveness in thermal energy storage, are also widely adopted in greenhouse technology to recover stored waste heat (Yan et al., 2021). In the research, Yan et al. (2021) reported that use of PCM plate containers reduced consumption of gas in the greenhouse by 23.7% and a return on investment was also made in a period of 4 months. In another study, Chen et al. (2020) reported similar findings as Yan et al. (2021) and revealed that where PCM material was not adopted in a greenhouse case study, temperatures dropped to as low as 3.7°C while adopting the PCM led to maintenance of indoor temperature at 10°C. Chen et al. (2018) also highlighted that use of PCM in the walls of a greenhouse further increased the indoor temperature, soil temperature and accumulative temperature. The different studies therefore underscore the role of PCM in improving indoor temperature within greenhouses. As such, the future of smart greenhouse covering materials would be predicted by the pace of adoption of phase change materials and energy modelling for optimal regulation of the microclimate (Han et al., 2021; Mishra et al., 2020) to address temperature fluctuations (25–52°C) (Nayak and Tiwari, 2021). The role of phase change materials such as shape memory alloys, shape memory hybrids, and shape memory polymers were demonstrated by Han et al. (2021), Fiorito et al. (2016) and Maraveas et al. (2021). In the latter case, the utility of PCMs in the regulation of the ambient environment in greenhouse could be augmented by incident solar radiation-triggered shape memory effects. Further shape changes could be achieved by exploiting the hydrophilic aspects of hydrogels and water desorption.
The constraints associated with surface inadequacies must be resolved before the materials are employed in widespread applications. Additionally, there is an issue of cost, given that PCMs are expensive compared to standard materials (Mukherjee et al., 2021). Salerno et al. (2021) research reinforce earlier claims made by Mukherjee et al. (2021). In the latter case, the exorbitant cost of the PCMs was linked to the combination with other components, particularly the HVACs. In reality, it would be impractical to integrate HVACs into greenhouse structures from a cost perspective.
The HVAC-related cost constraints have been partly resolved through the creation of shape morphing building skins for buildings and greenhouse structures (Fiorito et al., 2016). The structures could be optimized through the integration of photovoltaic (PV) systems to provide power to control or activate the shape morphing materials. The cost-savings that accrue from PV-shape morphing materials for greenhouse structures do not provide a sufficient incentive for the transition from HVAC systems. According to Fiorito et al. (2016), the operation of shape morphing materials is contingent on a broad array of physical (peak/horizontal global solar radiation, orientation, weather conditions, solar penetration depth, workplace and vertical illuminance, window luminous, time of day, time of day, and conditioning) and physiological factors (solar radiation, view, and privacy). In real-life commercial applications, it might be challenging to optimize each of the listed parameters for optimal performance of shape morphing greenhouse skins.
Considering that there are no practical solutions to offset these challenges, the practicality of integrating self-aligning PV modules should be investigated. The pilot study investigations by Dahlqvist and Nilsson-Hedman (2015) confirmed that the idea was practical and feasible, except for the search algorithm requirements. The integration of search algorithms translates to higher capital costs, which are barriers to the widespread utilization of shape morphing skins in commercial greenhouse. In line with Dahlqvist and Nilsson-Hedman (2015), Hoogenboom (2019) notes the benefits of PCMs could be enhanced by increasing their level of responsiveness to sugars and other biomolecules CO2, mechanical stress, pH, and temperature. Alternatively, the challenge could be offset by transparent PV modules installed in a checkboard configuration to maximize the capture of incident radiation (Srinivasan and Muthukumar, 2021). The unique requirements of PCMs demonstrated the need for complementary materials such as LSCs.
6.3 Luminescent Solar Concentrators
Although commercially viable LSC materials have multiple limitations, improvements in technology, absorption and photoluminescence, could translate to greater commercial suitability of dyes (El-bashir and Al-jaghwani, 2020) lanthanide complexes, colloid nanocrystals, and stokes shift engineered nanocrystals (see Figure 6). Previously, it was challenging to synthesize large quantities of LSC materials/chromophores with customized material properties for efficient solar collection and concentration (Meinardi et al., 2017); this explains the low PCE and EQE values (3.4 and 5.9%) of copper-doped InP/ZnSe quantum dots for luminescent solar concentrators (Eren et al., 2021). Different approaches must be employed for type A and type B chromophores to customize the absorption coefficient and the optical absorption spectrum. The comparison of the different LSC materials clearly demonstrates that PbS has greater spectral coverage compared to Eu (TTA)3 (TTPO)2], and 4-dicyanomethyl-6-dimethylaminostiryl-4H-pyran (DCM) (Meinardi et al., 2017). In brief, recent successes and innovations have contributed to a renaissance in the field of LSCs, given that most of the pressing design and synthesis challenges have been resolved.
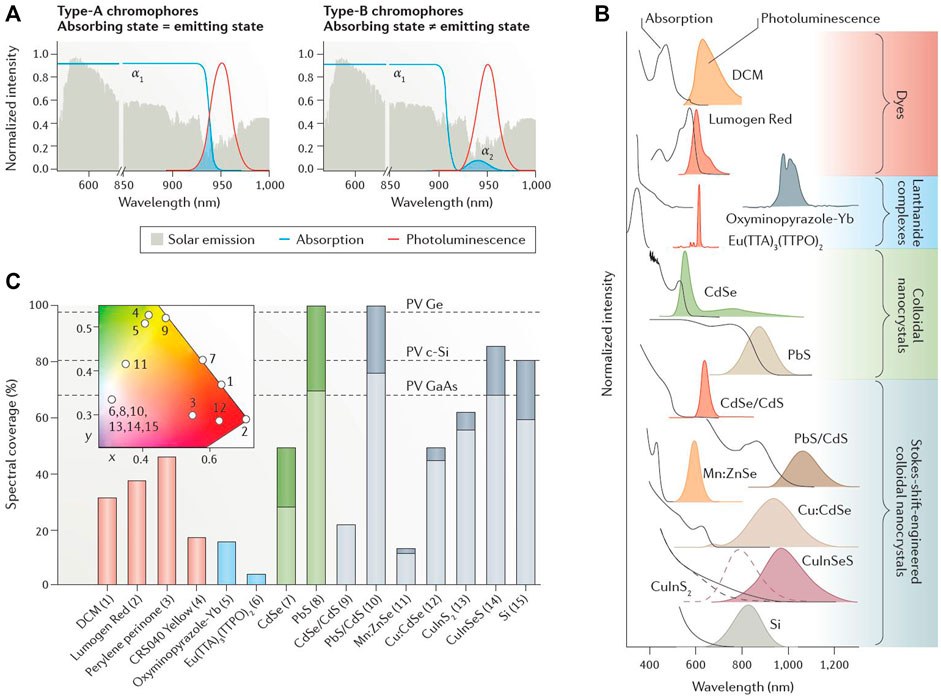
FIGURE 6. (A) Type-a and Type-b chromophores absorbance and emission state (B) normalized intensity (C) spectral coverage % of PbS, CdSe, Lumogen red, and Eu (TTA)3 (TTPO)2], and 4-dicyanomethyl-6-dimethylaminostiryl-4H-pyran (DCM) (Meinardi et al., 2017).
6.4 Barriers to the Future Adoption of PV Modules in Greenhouse
Even though fullerenes. LSCs, and PCMs would resolve the inadequacy of existing materials, the LCOE (cost and ecological impact), variability of solar radiation, and ecological degradation.
6.4.1 LCOE of PV Transparent Modules
Beyond seasonal light variability, the cost of retrofitting must be taken into account before the integration of photovoltaic panels. The costs are not defined, given they depend on the existing greenhouse structures, and the energy requirements, and climate control systems (Notte et al., 2020). However, the high initial setup costs would be offset by the projected energy savings in the long term; this is equivalent to about 40–60% of the solar energy bill (Renewable Energy Hub, 2020). The actual value of the savings was higher after taking into account the notable reduction in carbon emissions (Cibi and Manikandan, 2021). Recent studies on the levelised cost of energy for solar photovoltaic panels and environmental impact noted that the energy benefits (LCOE) were not guaranteed unless certain parameters were met and satisfied. First, the cradle to grave LCOE showed that investment return and reduction in the carbon footprint was dependent on a low degradation rate of the PV system, which is critical in large-scale installations. Second, polycrystalline materials were capable of generating electricity priced at 0491–0.0605 USD/kWh (Ludin et al., 2021) over a payback period of 7–10 years. The long duration exceeded the lifespan of polymer covers for greenhouse structures. The extended payback time might not be an appealing proposition for investors; this challenge could be resolved using emerging technologies for PV systems. On a positive note, recent inventions have translated to greater energy self-sufficiency. Current greenhouse configurations can generate up to 31 kWh/m2 using PV modules annually (Wu et al., 2020). Such energy is sufficient to satisfy the power needs.
The case for intelligent PV materials in farms is also supported by recent pilot studies (Rao et al., 2018), which noted that glass-like solar panels made of organic materials could help greenhouse become energy neutral (Newton, 2020). Traditional solar cells were unable to serve the dual function considering they were opaque; hence light could not reach the plants to trigger photosynthesis. In contrast, the novel intelligent PV materials were able to demonstrate superior performance through optimal energy harvesting and storage and utilization. The optimization of energy use could be enhanced through the incorporation of IoT systems, such as the AT89S52 microcontroller and temperature radars (Rao et al., 2018). The integration of sensors was vital given the daytime variations in the solar radiation intensity. The smart IoT-based sensors were able to differentiate between different ecological conditions, light, dampness, and temperature for optimal plant growth.
In addition to smart IoT-based systems in PV modules, biomimetic design has been employed in the development of shape morphing solar shadings (Fiorito et al., 2016) that mimic natural plants through the use of robotics and electronics. The climate adaptive building skins have been proven reliable and cost-effective, given that there is no energy expenditure in the adjustment of the façade. In the current case, the intelligent building skins would be most appropriate for the smart greenhouse, given their adaptability is not confined to environmental conditions in isolation. In contrast, the adaptive climate skins and climate adaptive building shells were responsive to weather patterns.
6.4.2 Solar Energy-Related Constraints and Circular Energy Use
Even though solar energy remains the most viable option for self-sustaining energy independent greenhouse structures, certain issues must be resolved. The most pressing concern relates to the variable solar irradiation linked to weather patterns and the local geographical conditions (Shankar et al., 2021). Solar energy production is inadequate during winter; this means dessert and tropical areas are suitable for optimal solar energy generation. In another study, Ben Amara et al. (2021) evaluated the climate behaviour during winter and summer days inside a greenhouse that had PV panels integrated on its roof. From the research, findings showed that farmers in Mediterrenean climates benefited from shading that was induced by south-oriented PV panels. As such, due to the constraints of solar irradiation in Mediterrenean climates, insights suggest that southward orientation of the panels is beneficial for the growth of plants as they provide shading and decrease temperature through the reduction in thermal load within the greenhouse.
However, the energy-related benefits associated with solar energy production are offset by dust and sand accumulation, which is facilitated by the strong Van der Waal adhesive forces between the dust and the PV panels (Darwish et al., 2013; Chen et al., 2019). Extended sand and dust accumulation translated to a significant reduction in conversion efficiency and performance. The current innovations do not provide a practical solution given that existing electrostatic, electro-mechanical, mechanical and natural cleaning options available are expensive, unsustainable or unable to overcome the strong Van der Waal forces. In light of the constraints associated with existing materials, more research is required to mitigate the effects of environmental variables on solar energy generation. In the meantime, the unique benefits associated with the development of novel and smart materials and IoT systems for greenhouse would be offset by the negative effects of the environment on solar energy harvesting from greenhouse.
6.4.3 Potential Solutions: Polymer Electrolyte Membrane Fuel Cell Stacks, Double Skin Facades and ANNs
From another dimension, the constraints associated with solar energy demonstrate the need for combined intervention to reduce the energy demands in greenhouse. Modelling analyses have confirmed the practical benefits associated with integrating Polymer Electrolyte Membrane (PEM) fuel cell stacks, electrolyzer bank and solar photovoltaic panels (Ganguly et al., 2010). PV modules generate electricity, some of which is channeled to the electrolyzer bank for H2 gas production. The H2 gas is consumed by PEM fuel cell stacks during periods of low sunshine or high grid demand. Alternatively, the excess PV energy could be channeled to the micro-grids (Ouammi et al., 2020). The progress made with double skin facades (DSF) in commercial and residential structures could be transferred to greenhouse structures to facilitate economic use of energy in commercial agriculture (Salerno et al., 2021). The DSF proposal is grounded on the system’s proven energy-related savings (Salerno et al., 2021). Beyond DSF, the integration of IoT materials could facilitate energy savings through the optimization of the performance of PV modules. The potential cost savings would be significant in absolute terms, given the cost of electricity for greenhouse heat pumps, circulation pumps, and ventilation units were about 900 Euros in 2007 (7.34 MW h/a) (Korecko et al., 2010). At present, the costs might surpass 1,200 Euros after taking into account the 2% annual rate of inflation in Europe (Eurostat, 2021). The cost does not include other indirect expenses that might be incurred in the regulation of the microclimate. In addition to PV-PEM-electrolyzer modules and DSF, PV solar cells can be paired with solar air collectors, drying units, and rotary desiccant wheel to optimize energy use (Saini et al., 2017). The energy savings that accrue from the circular use of solar energy might offset the periodical variations in solar radiation intensity.
Based on the recent progress made in the development of smart sensors and IoT devices for greenhouse, the merits of semitransparent PV modules and transparent greenhouse covering materials outweighed the risks given that sensors and neural networks in greenhouse could help regulate the microclimate’s temperature and humidity. The practical utilization of FES and ANN models and sensors was demonstrated by Castañeda-Miranda and Castaño-Meneses (2020b) in black-frost prediction. A similar principle could be employed to regulate the internal greenhouse temperature through the automatic activation of the water system by the fuzzy expert system. Alternatively, the sensors and IoT devices could mitigate excessive temperature fluctuations through the control system of medium moisture control and irrigation timing intelligent systems (Sagheer et al., 2020). The self-sustaining and autonomous technologies could gradually phase out traditional manual systems that were time and resource-intensive. In contrast to the previous model, the latter system was self-sustaining and integrated with internet services, GSM mobile systems, and solar panels for energy harvesting.
The weather-related changes in solar radiation were amplified by material-related factors such as the heat transfer coefficients and poor thermal inertia. The tradeoff between optimal utilization of solar energy using semitransparent PV modules and the compromised greenhouse microclimate due to the high heat transfer coefficient and poor thermal inertia of transparent greenhouse cladding materials raises fundamental questions about the role of research and development in offsetting these challenges.
From a futuristic perspective, the role of ANN and other neural networks would not be confined to one aspect of commercial agriculture. In addition to facilitating the control of the greenhouse microstructure, Recurrent LSTM Neural Network (R-LSTM-NN) and Deep Belief Network (DBN) (Batov, 2015; Raza and Khosravi, 2015; Lokshina et al., 2019; Zhang K. et al., 2021) have been proven useful in real-life fire hazard prevention and hazard warning (Bu and Gharajeh, 2019). genetic algorithm (GA), and adaptive neuro-fuzzy-inference-system (ANFIS), and artificial neural network (ANN), have been proven useful in similar applications (Arabasadi et al., 2013; Naser, 2019; Ronchi et al., 2019) and ecological monitoring (Molinara et al., 2021) using vision-based sensors (Bu and Gharajeh, 2019). The real-life applications would be augmented by robust R&D.
6.5 Limitations
The scope of this discussion was directed towards smart materials for greenhouse covering; this resulted in a lesser emphasis on the advanced applications of smart materials, including fire hazard prevention in agricultural structures, structural health monitoring, life cycle assessment, commercial production, and plant health monitoring. Beyond the scope-related limitations, the body of knowledge relating to smart materials for greenhouse was limited by varied sentiments about the cost, level of consumer acceptance of new technologies, and inadequate transfer of R&D innovations from the lab to real-world applications (Mukherjee et al., 2021). Other issues that should be resolved in upcoming studies relate to the implications of smart-greenhouse covering materials on crop physiology (Aguilar-santana et al., 2020). The resolution should be informed by experimental data rather than simulations. The simulations of smart material’s ability to selectively filter certain ranges of wavelength (PAR, NIR, TIR) provides estimates that might deviate from the actual values after accounting for external environmental/meteorological parameters (Baeza et al., 2020). In addition, it is challenging to accurately estimate the domino effects on fruit quality and risk of diseases following the incorporation of smart greenhouse covering materials.
Smart glass films block about 9% of PAR and more than half of the entire short-wave radiation. The cumulative effect was impaired plant growth characterized by Ca2+ flux, Cl− and K+ flux, leaf water loss, pronounced sensitivity to abscisic acid (ABA-stress hormone) and suppressed stomatal pore sizes (Aguilar-santana et al., 2020), and the stomatal opening and closing rates; thus impairing the rate of photosynthesis. The observed variations in crop physiology in thin glass film greenhouse illustrate the need for exhaustive experimental trials prior to the commercial deployment of the smart materials. At present, it is challenging to ascertain how copper-doped InP/ZnSe QDs and Pb-free materials, Pb Halide Perovskites, 2D Ruddlesden–Popper perovskite-based solar cells (PVSCs), smart photovoltaic (PV) Pb-quantum dots, and amorphous tungsten oxide (WO3) films impact crop physiology.
7 Conclusion
The in-depth review of smart materials for covering greenhouse structures demonstrated there were unique benefits associated with the installation of greenhouse covering materials made of semitransparent solar PV modules. The case for shared structure and sunlight systems, shared structures is suitable compared to the adjacent materials is based on cost considerations. Recent R&D projects have resulted in the development of hundreds of PV materials for greenhouse ranging from Building Integrated Photovoltaic (BIPV) and Electrochromic Glazing (EG) and smart photovoltaic (PV) materials comprising of Pb-quantum dots, amorphous tungsten oxide (WO3) films, copper-doped InP/ZnSe QDs and Pb-free materials, Pb Halide Perovskites, copper-doped InP/ZnSe QDs, semitransparent (ST) organic solar cells (OSCs), and 2D Ruddlesden–Popper perovskite-based solar cells (PVSCs). Following the assessment of the different classes of materials, it was deduced that luminescent solar concentrators (LSCs) featuring copper-doped InP/ZnSe QDs and other LSC configurations were less appropriate for greenhouse covering due to the low PCE (<10%). The contrary is true for semitransparent (ST) organic solar cells (OSCs), which had a PCE of 17%. The variations in PCE, plant growth factor, ecological impact, cost, durability, and performance, PAR and crop yield, levelised cost of energy (LCOE) in copper-doped InP/ZnSe QDs and Pb-free materials, Pb Halide Perovskites, 2D Ruddlesden–Popper perovskite-based solar cells (PVSCs), smart photovoltaic (PV) Pb-quantum dots, and amorphous tungsten oxide (WO3) and other materials explained the need to narrow down the focus on most promising materials for future applications. Even though there are practical constraints to the adoption of LSCs, progress has been made in the customization of type A and type B chromophore’s absorption coefficient and the optical absorption spectrum; this explains why LSCs were considered integral to the future of smart covering materials under Section 4. Beyond LSCs, fullerene-based materials and PCMs possess the desired properties.
Futuristic technological solutions in the synthesis of nanomaterials at the micro and macro scales would help broaden the application of smart covering materials for greenhouse structures. Such innovations are critical to the resolution of the ambient degradation problem in Pb-based QDs, surface oxidation, loss of performance and poor stability while mitigating the adverse environmental effects. The development of intelligent materials embedded with neural networks (Perez-Alonso et al., 2012) and fuzzy logic IoT would help to further reduce the cost of operating greenhouse, given that the transparent nanogenerators are capable of autonomously capturing solar radiation while self-aligning-AI guided PVs capture optimal solar radiation. In the long-run, the nanotechnologies are anticipated to positively impact human life as they enhance management practices in commercial agriculture. As a result, better investments can be made in greenhouse to promote food production. However, the promising applications of technological solutions for greenhouse could be derailed by the lack of consensus among key stakeholders. Certain scholars believe that the integration of AI and machine learning algorithms in greenhouse covering materials was not an effective solution in large scale and modern greenhouse. A contrary view is advanced by the proponents of smart greenhouse solutions. The former worldview was further reinforced by the extremely vulnerability of PVs to external environmental/meteorological parameters.
Despite the lack of consensus, there is irrefutable evidence that smart materials for greenhouse covers would minimize energy consumption (through energy-independent PV modules), improve crop yields through the regulation of the microclimate and reduce CO2 emissions. On the same note, critical examination of the different applications of smart greenhouse technologies indicates that the innovations also lead to economic benefits especially with regard to reduction in costs for heating or cooling the greenhouse. A case in point was the PCMs which led to reduced costs of operating greenhouses as they led to stable temperature conditions in the ambient environment. The insights also indicate that the technologies reduce maintenance costs for the greenhouse as less human resources are required to manage the greenhouses due to automation advantages. Future research should be directed towards advanced applications of smart materials such as pre-hazard warning, independent assessment of structural health, life cycle assessment, large scale production, and cost reduction.
Author Contributions
CM and DL manuscript preparation, TB, KA, and JU review and edit.
Conflict of Interest
The authors declare that the research was conducted in the absence of any commercial or financial relationships that could be construed as a potential conflict of interest.
Publisher’s Note
All claims expressed in this article are solely those of the authors and do not necessarily represent those of their affiliated organizations, or those of the publisher, the editors and the reviewers. Any product that may be evaluated in this article, or claim that may be made by its manufacturer, is not guaranteed or endorsed by the publisher.
Acknowledgments
The authors acknowledge TCB Avgidis Automation SA for paying this publication’s APC.
Abbreviations
ANN, Artificial Neural Network: ANN are part of neural networks used in the automation of greenhouse structures (Castañeda-Miranda and Castaño-Meneses, 2020a); BIPV, Building-integrated photovoltaics. BIPVs contribute to the optimization of daylight savings and generation of clean energy at the site of use (Scognamiglio, 2017; Aguacil et al., 2019; Gholami et al., 2019); CNT, Carbon nanotubes: CNTs are carbon allotropes with single-walled and multi-walled configurations (Wan et al., 2019); DCM, 4-dicyanomethyl-6-dimethylaminostiryl-4H-pyran (Meinardi et al., 2017); DSSC, dye-sensitized solar cells (Notte et al., 2020); DLS, Dynamic light scattering (Lian et al., 2019); ECE, external quantum efficiency (Chalkias et al., 2021); EG, Electrochromic Glazing (Katanbafnasab et al., 2013); IoT, Internet of things (Mishra and Kertesz, 2020); ITC, irrigation timing (Touhami et al., 2020); LCOE, Levelised cost of energy: LCOE compares the cost of solar energy compared to grid-supplied electricity (Ludin et al., 2021; Shankar et al., 2021); LCA, Life cycle analysis: The LCA analysis focuses on ecological impact of materials from cradle to grave (Ludin et al., 2021); MMC, Medium moisture control (Mishra and Kertesz, 2020); MMCT, Metal-to-metal charge transfer (Mishra and Kertesz, 2020); PAR, Photosynthetic active radiation (Chalkias et al., 2021); PV, Photovoltaics (Chalkias et al., 2021); PVSCs, 2D Ruddlesden–Popper perovskite-based solar cells (Mtz-Enriquez et al., 2020); QD, Quantum dots (Pulli et al., 2020); ST, OSC semitransparent organic solar cells (Pulli et al., 2020); ST, PSC semitransparent polymer solar cell (Pulli et al., 2020); MLCT, Transition and metal-to-ligand charge transfer (He and Yao, 2007); WPSS, Water Pumping Storage Systems (He and Yao, 2007).
References
Agathokleous, R. A., Kalogirou, S. A., and Karellas, S. (2018). Exergy Analysis of a Naturally Ventilated Building Integrated Photovoltaic/Thermal (BIPV/T) System. Renew. Energ. 128, 541–552. doi:10.1016/j.renene.2017.06.085
Aguacil, S., Lufkin, S., and Rey, E. (2019). Active Surfaces Selection Method for Building-Integrated Photovoltaics (BIPV) in Renovation Projects Based on Self-Consumption and Self-Sufficiency. Energy and Buildings 193, 15–28. doi:10.1016/j.enbuild.2019.03.035
Aguilar-santana, J. L., Jarimi, H., Velasco-Carrasco, M., and Riffat, S. (2020). Review on Window-Glazing Technologies and Future Prospects. Int. J. Low-Carbon Tech. 15, 112–120. doi:10.1093/ijlct/ctz032
Ahmad Ludin, N., Ahmad Affandi, N. A., Purvis-Roberts, K., Ahmad, A., Ibrahim, M. A., Sopian, K., et al. (2021). Environmental Impact and Levelised Cost of Energy Analysis of Solar Photovoltaic Systems in Selected Asia Pacific Region: A Cradle-To-Grave Approach. Sustainability 13 (1), 396. doi:10.3390/su13010396
Albaladejo‐Siguan, M., Baird, E. C., Becker‐Koch, D., Li, Y., Rogach, A. L., and Vaynzof, Y. (2021). Stability of Quantum Dot Solar Cells: A Matter of (Life)Time. Adv. Energ. Mater. 11 (12), 2003457. doi:10.1002/aenm.202003457
Allardyce, C. S., Fankhauser, C., Zakeeruddin, S. M., Grätzel, M., and Dyson, P. J. (2017). The Influence of Greenhouse-Integrated Photovoltaics on Crop Production. Solar Energy 155, 517–522. doi:10.1016/j.solener.2017.06.044
Arabasadi, Z., Khorasani, M., Akhlaghi, S., Fazilat, H., Gedde, U. W., Hedenqvist, M. S., et al. (2013). Prediction and Optimization of Fireproofing Properties of Intumescent Flame Retardant Coatings Using Artificial Intelligence Techniques. Fire Saf. J. 61, 193–199. doi:10.1016/j.firesaf.2013.09.006
Aroca-delgado, R., Pérez-Alonso, J., Callejón-Ferre, Á.-J., and Díaz-Pérez, M. (2019). Morphology, Yield and Quality of Greenhouse Tomato Cultivation with Flexible Photovoltaic Rooftop Panels (Almería-Spain). Scientia Horticulturae 257 (March), 108768. doi:10.1016/j.scienta.2019.108768
Aspragathos, N., Dogkas, E., Konstantinidis, P., Koutmos, P., Lamprinou, N., Moulianitis, V. C., et al. (2019). From Pillars to AI Technology-Based Forest Fire Protection Systems. Intell. Syst. Comput. 13. Intechopen. Available at: https://www.intechopen.com/books/advanced-biometric-technologies/liveness-detection-in-biometrics.
Baeza, E. J., Van Breugel, A. J. B., Hemming, S., and Stanghellini, C. (2020). Smart Greenhouse Covers: a Look into the Future. Acta Hortic., 213–224. doi:10.17660/ActaHortic.2020.1268.28
Batov, E. I. (2015). The Distinctive Features of "Smart" Buildings. Proced. Eng. 111 (TFoCE), 103–107. doi:10.1016/j.proeng.2015.07.061
Becker-Koch, D., Albaladejo-Siguan, M., Lami, V., Paulus, F., Xiang, H., Chen, Z., et al. (2019). Ligand Dependent Oxidation Dictates the Performance Evolution of High Efficiency PbS Quantum Dot Solar Cells. Sustain. Energ. Fuels 4 (1), 108–115. doi:10.1039/c9se00602h
Behzadi, A., and Arabkoohsar, A. (2020). Feasibility Study of a Smart Building Energy System Comprising Solar PV/T Panels and a Heat Storage Unit. Energy 210, 118528. doi:10.1016/j.energy.2020.118528
Ben Amara, H., Bouadila, S., Fatnassi, H., Arici, M., and Allah Guizani, A. (2021). Climate Assessment of Greenhouse Equipped with South-Oriented PV Roofs: An Experimental and Computational Fluid Dynamics Study. Sustainable Energ. Tech. Assessments 45, 101100. doi:10.1016/j.seta.2021.101100
Bershadskii, A. (2008). Coherent Phenomena at the Last Scattering in the CMB Light. Phys. Lett. A 372, 2741–2745. doi:10.1016/j.physleta.2007.12.041
Bhattarai, A., and Wilczura-wachnik, H. (2015). Size and Diffusion Phenomena of AOT/alcohol/water System in the Presence of Morin by Dynamic Light Scattering. Int. J. Pharmaceutics 478 (2), 610–616. doi:10.1016/j.ijpharm.2014.11.037
Biyik, E., Araz, M., Hepbasli, A., Shahrestani, M., Yaob, R., and Shaob, L. (2017). A Key Review of Building Integrated Photovoltaic (BIPV) Systems. Eng. Sci. Technol. Int. J. 20, 833. doi:10.1016/j.jestch.2017.01.009
Bjorn, P. J., Breivik, C., and Drolsum, H. (2012). Building Integrated Photovoltaic Products : A State of the Art Review and Future Research Opportunities. Solar Energy Materials and Solar Cells 100, 69–96. doi:10.1016/j.solmat.2011.12.016
Blackburn, J. L. (2017). Semiconducting Single-Walled Carbon Nanotubes in Solar Energy Harvesting. ACS Energ. Lett. 2 (7), 1598–1613. doi:10.1021/acsenergylett.7b00228
Bu, F., and Gharajeh, M. S. (2019). Intelligent and Vision-Based Fire Detection Systems: A Survey. Image Vis. Comput. 91, 103803. doi:10.1016/j.imavis.2019.08.007
Camarinha-Matos, L. M., Falcão, A. J., Vafaei, N., and Najdi, S. (2016). “Technological Innovation for Cyber-Physical Systems,” in DoCEIS 2016 7th IFIP WG 5.5/SOCOLNET Advanced Doctoral Conference on Computing, Electrical and Industrial Systems, Costa de Caparica, Portugal, April 11–13, 2016.
Castañeda-Miranda, A., and Castaño-Meneses, V. M. (2020a). Internet of Things for Smart Farming and Frost Intelligent Control in Greenhouses. Comput. Electron. Agric. 176, 105614. doi:10.1016/j.compag.2020.105614
Castañeda-Miranda, A., and Castaño-Meneses, V. M. (2020b). Smart Frost Measurement for Anti-disaster Intelligent Control in Greenhouses via Embedding IoT and Hybrid AI Methods. Measurement 164, 108043. doi:10.1016/j.measurement.2020.108043
Celik, I., Mason, B. E., PhillipsPhillips, A. B., Heben, M. J., and Apul, D. (2017). Environmental Impacts from Photovoltaic Solar Cells Made with Single Walled Carbon Nanotubes. Environ. Sci. Technol. 51 (8), 4722–4732. doi:10.1021/acs.est.6b06272
Chalkias, D. A., Charalampopoulos, C., Andreopoulou, A. K., Karavioti, A., and Stathatos, E. (2021). Spectral Engineering of Semi-transparent Dye-Sensitized Solar Cells Using New Triphenylamine-Based Dyes and an Iodine-free Electrolyte for Greenhouse-Oriented Applications. J. Power Sourc. 496, 229842. doi:10.1016/j.jpowsour.2021.229842
Chan, S. (2019). Building Integrated Photovoltiacs (BiPV) Can Be Low Cost Crystalline Si Based BiPV. Available at: https://www.kschan.com/building-integrated-photovoltaics-bipv-can-be-low-cost/ (Accessed September 9, 2021).
Chen, C., Ling, H., Zhai, Z., Li, Y., Yang, F., Han, F., et al. (2018). Thermal Performance of an Active-Passive Ventilation wall with Phase Change Material in Solar Greenhouses. Appl. Energ. 216, 602–612. doi:10.1016/j.apenergy.2018.02.130
Chen, S., Zhu, Y., Chen, Y., and Liu, W. (2020). Usage Strategy of Phase Change Materials in Plastic Greenhouses, in Hot Summer and Cold winter Climate. Appl. Energ. 277, 115416. doi:10.1016/j.apenergy.2020.115416
Chen, Y., Liu, Y., Tian, Z., Dong, Y., Zhou, Y., Wang, X., et al. (2019). Experimental Study on the Effect of Dust Deposition on Photovoltaic Panels. Energ. Proced. 158, 483–489. doi:10.1016/j.egypro.2019.01.139
Chiesa, G., Di Vita, D., Ghadirzadeh, A., Muñoz Herrera, A. H., and Leon Rodriguez, J. C. (2020). A Fuzzy-Logic IoT Lighting and Shading Control System for Smart Buildings. Automation in Construction 120 (July), 103397. doi:10.1016/j.autcon.2020.103397
Chou, H.-T., Chen, Y.-C., Lee, C.-Y., Chang, H.-Y., and Tai, N.-H. (2017). Switchable Transparency of Dual-Controlled Smart Glass Prepared with Hydrogel-Containing Graphene Oxide for Energy Efficiency. Solar Energ. Mater. Solar Cell 166 (December 2016), 45–51. doi:10.1016/j.solmat.2017.01.025
Cibi, M. R., and Manikandan, S. (2021). Concise Overview of BIPV Systems and its Future Scope. IOP Conf. Ser. Mater. Sci. Eng. 1130, 012033. doi:10.1088/1757-899X/1130/1/012033
ClearVue (2021). Converting Sunlight to Electricity with Clear Solar Glass. Available at: https://www.archdaily.com/959593/converting-sunlight-to-electricity-with-clear-solar-glass (Accessed August 12, 2021).
Cossu, M., Yano, A., Li, Z., Onoe, M., Nakamura, H., Matsumoto, T., et al. (2016). Advances on the Semi-transparent Modules Based on Micro Solar Cells: First Integration in a Greenhouse System. Appl. Energ. 162, 1042–1051. doi:10.1016/j.apenergy.2015.11.002
Cossu, M., Yano, A., Solinas, S., Deligios, P. A., Tiloca, M. T., Cossu, A., et al. (2020). Agricultural Sustainability Estimation of the European Photovoltaic Greenhouses. Eur. J. Agron. 118 (January), 126074. doi:10.1016/j.eja.2020.126074
Dahlqvist, M., and Nilsson-Hedman, T. (2015). Self-Aligning Solar Panel: Construction of a Self-Aligning Platform for Solar Panels. KTH, Degree Project.
Darwish, Z., Kazem, H., Sopian, K., Alghoul, M. A., and Chaichan, M. (2013). Impact of Some Environmental Variables with Dust on Solar Photovoltaic (PV) Performance: Review and Research Status. Int. J Energ. Environ. 7 (4), 152–159.
Deb, S. K. (2000). Photovoltaic-Integrated Electrochromic Device for Smart-Window Applications Preprint. no. May.
Della Lunga, D., BryeBrye, K. R., Slayden, J. M., Henry, C. G., and Wood, L. S. (2021). Relationships Among Soil Factors and Greenhouse Gas Emissions from Furrow-Irrigated Rice in the Mid-southern, USA. Geoderma Reg. 24, e00365. doi:10.1016/j.geodrs.2021.e00365
Deng, B., Liu, Z., and Peng, H. (2019). Toward Mass Production of CVD Graphene Films. Adv. Mater. 31 (9), 1800996. doi:10.1002/adma.201800996
Dey, T., and Naughton, D. (2019). Nano-Porous Sol-Gel Derived Hydrophobic Glass Coating for Increased Light Transmittance through Greenhouse. Mater. Res. Bull. 116 (April), 126–130. doi:10.1016/j.materresbull.2019.04.027
El-bashir, S. M., and Al-jaghwani, A. A. (2020). Perylene-doped Polycarbonate Coatings for Acrylic Active Greenhouse Luminescent Solar Concentrator Dryers. Results Phys. 16 (December 2019), 102920. doi:10.1016/j.rinp.2019.102920
Elfrink, R., Burghoorn, M., Zevenbergen, M., Hal, R. v., and Schoo, H. (2019). “Integrated Climate and Water Sensors for Greenhouses,” in Smart Systems Integration; 13th International Conference and Exhibition on Integration Issues of Miniaturized Systems, Barcelona, Spain, 1–8.
Emmott, C. J. M., Röhr, J. A., Campoy-Quiles, M., Kirchartz, T., Urbina, A., Ekins-Daukes, N. J., et al. (2015). Organic Photovoltaic Greenhouses: A Unique Application for Semi-transparent PV? Energy Environ. Sci. 8 (4), 1317–1328. doi:10.1039/C4EE03132F
Eren, G. O., Sadeghi, S., Shahzad, M., and Nizamoglu, S. (2021). Protocol on Synthesis and Characterization of Copper-Doped InP/ZnSe Quantum Dots as Ecofriendly Luminescent Solar Concentrators with High Performance and Large Area. STAR Protoc. 2 (3), 100664. doi:10.1016/j.xpro.2021.100664
Fiorito, F., Sauchelli, M., Arroyo, D., Pesenti, M., Imperadori, M., Masera, G., et al. (2016). Shape Morphing Solar Shadings: A Review. Renew. Sustain. Energ. Rev. 55, 863–884. doi:10.1016/j.rser.2015.10.086
Friman Peretz, M., Geoola, F., Yehia, I., Ozer, S., Levi, A., Magadley, E., et al. (2019). Testing Organic Photovoltaic Modules for Application as Greenhouse Cover or Shading Element. Biosyst. Eng. 184, 24–36. doi:10.1016/j.biosystemseng.2019.05.003
Friman-peretz, M., Ozer, S., Levi, A., Magadley, E., Yehia, I., Geoola, F., et al. (2021). Energy Partitioning and Spatial Variability of Air Temperature, VPD and Radiation in a Greenhouse Tunnel Shaded by Semitransparent Organic PV Modules. Solar Energy 220 (April), 578–589. doi:10.1016/j.solener.2021.03.050
Ganguly, A., Misra, D., and Ghosh, S. (2010). Modeling and Analysis of Solar Photovoltaic-Electrolyzer-Fuel Cell Hybrid Power System Integrated with a Floriculture Greenhouse. Energy and Buildings 42 (11), 2036–2043. doi:10.1016/j.enbuild.2010.06.012
Gautam, Y. K., Sharma, K., Tyagi, S., Ambedkar, A. K., Chaudhary, M., and Pal Singh, B. (2021). Nanostructured Metal Oxide Semiconductor-Based Sensors for Greenhouse Gas Detection: Progress and Challenges. R. Soc. Open Sci. 8 (3), 201324. doi:10.1098/rsos.201324
Gesheva, K. A., Ivanova, T. M., and Bodurov, G. (2012). Transition Metal Oxide Films: Technology and "Smart Windows" Electrochromic Device Performance. Prog. Org. Coat. 74 (4), 635–639. doi:10.1016/j.porgcoat.2011.07.016
Ghalandari, M., Maleki, A., Haghighi, A., Safdari Shadloo, M., Alhuyi Nazari, M., and Tlili, I. (2020). Applications of Nanofluids Containing Carbon Nanotubes in Solar Energy Systems: A Review. J. Mol. Liquids 313, 113476. doi:10.1016/j.molliq.2020.113476
Gholami, H., Røstvik, H. N., and Müller-Eie, D. (2019). Holistic Economic Analysis of Building Integrated Photovoltaics (BIPV) System: Case Studies Evaluation. Energy and Buildings 203, 109461. doi:10.1016/j.enbuild.2019.109461
Giglio, M., and Vailati, A. (1995). Light Scattering Studies of Aggregation Phenomena. Physica A 213, 148–158.
González-Henríquez, C. M., Sarabia-Vallejos, M. A., Rodriguez-Hernandez, J., and Rodriguez-Hernandez, J. (2019). Polymers for Additive Manufacturing and 4D-Printing: Materials, Methodologies, and Biomedical Applications. Prog. Polym. Sci. 94, 57–116. doi:10.1016/j.progpolymsci.2019.03.001
Han, F., Chen, C., Hu, Q., He, Y., Wei, S., and Li, C. (2021). Modeling Method of an Active-Passive Ventilation wall with Latent Heat Storage for Evaluating its thermal Properties in the Solar Greenhouse. Energy and Buildings 238, 110840. doi:10.1016/j.enbuild.2021.110840
He, T., and Yao, J. (2007). Photochromic Materials Based on Tungsten Oxide. J. Mater. Chem. 17 (43), 4547–4557. doi:10.1039/b709380b
Hoogenboom, R. (2019). “Temperature-Responsive Polymers: Properties, Synthesis, and Applications,” in Smart Polymers and Their Applications. Second Edi (Amsterdam: Elsevier), 13–44. doi:10.1016/B978-0-08-102416-4.00002-8
Huang, H., Bian, H., Zhu, S., and Jin, J. (2011). A Greenhouse Remote Monitoring System Based on GSM. 2011 Int. Conf. Inf. Manag. Innovation Manage. Ind. Eng. 2, 357–360. doi:10.1109/ICIII.2011.231
Huang, L., Deng, L., Li, A., Gao, R., Zhang, L., and Lei, W. (2021). A Novel Approach for Solar Greenhouse Air Temperature and Heating Load Prediction Based on Laplace Transform. J. Building Eng. 44, 102682. doi:10.1016/j.jobe.2021.102682
Huang, L., Deng, L., Li, A., Gao, R., Zhang, L., and Lei, W. (2020). Analytical Model for Solar Radiation Transmitting the Curved Transparent Surface of Solar Greenhouse. J. Building Eng. 32 (August), 101785. doi:10.1016/j.jobe.2020.101785
Ifeanyi, A., Isherwood, P., and Abdul-Lateef, A. O. (2021). A Study of Copper-Tungsten Oxide Materials for Photovoltaic Application. World J. Eng. ahead-of-print. doi:10.1108/WJE-04-2021-0241
Joseph, B., Pogrebnaya, T., and Kichonge, B. (2019). Semitransparent Building-Integrated Photovoltaic: Review on Energy Performance, Challenges, and Future Potential. Int. J. Photoenergy. doi:10.1155/2019/5214150
Kamalisarvestani, M., Saidur, R., Mekhilef, S., and Javadi, F. S. (2020). Performance, Materials and Coating Technologies of Thermochromic Thin Films on Smart Windows. Renew. Sustain. Energ. Rev. 26, 353–364. doi:10.1016/j.rser.2013.05.038
Kar, S., Sizochenko, N., Ahmed, L., Batista, V. S., and Leszczynski, J. (2016). Quantitative Structure-Property Relationship Model Leading to Virtual Screening of Fullerene Derivatives: Exploring Structural Attributes Critical for Photoconversion Efficiency of Polymer Solar Cell Acceptors. Nano Energy 26, 677–691. doi:10.1016/j.nanoen.2016.06.011
Katanbafnasab, M., Abu-hijleh, B., and Al, E. T. (2013). Assessment of the Energy Impact of Using Building Integrated Photovoltaic and Electrochromic Glazing in Office Building in UAE. Eng 05, 56–61. doi:10.4236/eng.2013.51b01010.4236/eng.2013.51b110
Korecko, J., Jirka, V., Sourek, B., and Cerveny, J. (2010). Module Greenhouse with High Efficiency of Transformation of Solar Energy, Utilizing Active and Passive Glass Optical Rasters. Solar Energy 84 (10), 1794–1808. doi:10.1016/j.solener.2010.07.004
Kotsuchibashi, Y. (2020). Recent Advances in Multi-Temperature-Responsive Polymeric Materials. Polym. J. 52, 681–689. doi:10.1038/s41428-020-0330-0
Kwon, H.-K., Lee, K.-T., Hur, K., MoonMoon, S. H., QuasimQuasim, M. M., Wilkinson, T. D., et al. (2015). Optically Switchable Smart Windows with Integrated Photovoltaic Devices. Adv. Energ. Mater. 5, 1401347. doi:10.1002/aenm.201401347
Li, B., Shi, B., Yao, Z., Kumar Shukla, M., and Du, T. (2020). Energy Partitioning and Microclimate of Solar Greenhouse under Drip and Furrow Irrigation Systems. Agric. Water Manage. 234, 106096. doi:10.1016/j.agwat.2020.106096
Li, C., Wang, H., Miao, H., and Ye, B. (2017). The Economic and Social Performance of Integrated Photovoltaic and Agricultural Greenhouses Systems: Case Study in China. Appl. Energ. 190, 204–212. doi:10.1016/j.apenergy.2016.12.121
Lian, X., Chen, J., Qin, M., Zhang, Y., Tian, S., Lu, X., et al. (2019). The Second Spacer Cation Assisted Growth of a 2D Perovskite Film with Oriented Large Grain for Highly Efficient and Stable Solar Cells. Angew. Chem. 131 (28), 9509–9513. doi:10.1002/ange.201902959
Lin, L., Peng, H., and Liu, Z. (2019). Synthesis Challenges for Graphene Industry. Nat. Mater. 18 (6), 520–524. doi:10.1038/s41563-019-0341-4
Lokshina, I. V., Greguš, M., and Thomas, W. L. (2019). Application of Integrated Building Information Modeling, IoT and Blockchain Technologies in System Design of a Smart Building. Proced. Comput. Sci. 160, 497–502. doi:10.1016/j.procs.2019.11.058
Makableh, Y. F., Daibes, M., and Aljaiuossi, G. (2021). Large Optical and Structural Enhancement by Designing VOx Nanostructures for Smart Windows Applications. Mater. Sci. Eng. B 270, 115216. doi:10.1016/j.mseb.2021.115216
Maraveas, C. (2019). Environmental Sustainability of Greenhouse Covering Materials. Sustainability 11 (6129), 1–24. doi:10.3390/su11216129
Maraveas, C., and Bartzanas, T. (2021). Sensors for Structural Health Monitoring of Agricultural Structures. Sensors 21 (1), 314–332. doi:10.3390/s21010314
Maraveas, C., BayerBayer, I. S., and Bartzanas, T. (2021). 4D Printing: Perspectives for the Production of Sustainable Plastics for Agriculture. Biotechnol. Adv. In Press, 107785. doi:10.1016/j.biotechadv.2021.107785
Maraveas, C. (2020a). Environmental Sustainability of Plastic in Agriculture. Agriculture 10 (8), 310. doi:10.3390/agriculture10080310
Maraveas, C. (2020b). Production of Sustainable and Biodegradable Polymers from Agricultural Waste. Polymers 12 (1127), 1127. doi:10.3390/polym12051127
Mazzaro, R., and Vomiero, A. (2018). The Renaissance of Luminescent Solar Concentrators: The Role of Inorganic Nanomaterials. Adv. Energ. Mater. 8, 1801903–1801919. doi:10.1002/aenm.201801903
Meinardi, F., Bruni, F., and Brovelli, S. (2017). Luminescent Solar Concentrators for Building-Integrated Photovoltaics. Nat. Rev. Mater. 2, 1–9. doi:10.1038/natrevmats.2017.72
Mishra, B., and Kertesz, A. (2020). The Use of MQTT in M2M and IoT Systems: A Survey. IEEE Access 8, 201071–201086. doi:10.1109/ACCESS.2020.3035849
Mishra, P., Stockmal, K., Ardito, G., Tao, M., Van Dessel, S., and Granados-focil, S. (2020). Thermo-Optically Responsive Phase Change Materials for Passive Temperature Regulation. Solar Energy 197 (December 2019), 222–228. doi:10.1016/j.solener.2019.12.064
Molinara, M., Bria, A., De Vito, S., and Marrocco, C. (2021). Artificial Intelligence for Distributed Smart Systems. Pattern Recognition Lett. 142, 48–50. doi:10.1016/j.patrec.2020.12.006
Moretti, S., and Marucci, A. (2019). A Photovoltaic Greenhouse with Variable Shading for the Optimization of Agricultural and Energy Production. Energies 12 (13), 2589. doi:10.3390/en12132589
Mtz-Enriquez, A. I., Padmasree, K. P., Oliva, A. I., Gomez-Solis, C., Coutino-Gonzalez, E., Garcia, C. R., et al. (2020). Tailoring the Detection Sensitivity of Graphene Based Flexible Smoke Sensors by Decorating with Ceramic Microparticles. Sensors Actuators B: Chem. 305 (November 2019), 127466. doi:10.1016/j.snb.2019.127466
Mukherjee, A., Deepmala, P. S., Srivastava, P., and Sandhu, J. K. (2021). Application of Smart Materials in Civil Engineering: A Review. Mater. Today Proc. 5, 665. doi:10.1016/j.matpr.2021.03.304
Naser, M. Z. (2019). Fire Resistance Evaluation through Artificial Intelligence - A Case for Timber Structures. Fire Saf. J. 105, 1–18. doi:10.1016/j.firesaf.2019.02.002
Nayak, S., and Tiwari, G. N. (2021). Energy and Exergy Analysis of Photovoltaic/thermal Integrated with a Solar Greenhouse. Energy and Buildings 40 (2008), 2015–2021. doi:10.1016/j.enbuild.2008.05.007
Nefedov, E., and Vyatkin, V. (2016). Decentralised Coordination of Intelligent Autonomous Batteries. IFIP Adv. Inf. Commun. Technol. 470, 425–433. doi:10.1007/978-3-319-31165-4_40
Newton, M. (2020). Glass-Like Solar Panels Could Help Greenhouses Become Completely Energy Neutral. Available at: https://en.reset.org/blog/glass-solar-panels-could-help-greenhouses-become-completely-energy-neutral-02252020. (Accessed October 5, 2021).
Niu, J., Wang, Y., Zou, X., Tan, Y., Jia, C., Weng, X., et al. (2021). Infrared Electrochromic Materials, Devices and Applications. Appl. Mater. Today 24, 101073. doi:10.1016/j.apmt.2021.101073
Notte, L., Giordano, L., Calabrò, E., Bedini, R., Colla, G., Puglisi, G., et al. (2020). Hybrid and Organic Photovoltaics for Greenhouse Applications. Appl. Energ. 278 (July), 115582. doi:10.1016/j.apenergy.2020.115582
Omar, M. N., Taha, A. T., Samak, A. A., Keshek, M. H., Gomaa, E. M., and Elsisi, S. F. (2021). Simulation and Validation Model of Cooling Greenhouse by Solar Energy (P V) Integrated with Painting its Cover and its Effect on the Cucumber Production. Renew. Energ. 172, 1154–1173. doi:10.1016/j.renene.2021.03.092
Ong, P.-L., Euler, W. B., and Levitsky, I. A. (2010). Hybrid Solar Cells Based on Single-Walled Carbon Nanotubes/Si Heterojunctions. Nanotechnology 21 (10), 105203. doi:10.1088/0957-4484/21/10/105203
Ouammi, A., Achour, Y., Dagdougui, H., and Zejli, D. (2020). Optimal Operation Scheduling for a Smart Greenhouse Integrated Microgrid. Energ. Sustain. Develop. 58, 129–137. doi:10.1016/j.esd.2020.08.001
Pérez-Alonso, J., Pérez-García, M., Pasamontes-Romera, M., and Callejón-Ferre, A. J. (2012). Performance Analysis and Neural Modelling of a Greenhouse Integrated Photovoltaic System. Renew. Sustain. Energ. Rev. 16, 4675–4685. doi:10.1016/j.rser.2012.04.002
Pulli, E., Rozzi, E., and Bella, F. (2020). Transparent Photovoltaic Technologies: Current Trends towards Upscaling. Energ. Convers. Manage. 219 (February), 112982. doi:10.1016/j.enconman.2020.112982
Rao, M. N., Ajit, K. C., and Kumar, G. P. (2018). Smart Green House Based on IOT. Int. J. Eng. Technol. 7, 258–261. doi:10.14419/ijet.v7i2.32.15579
Ravishankar, E., Booth, R. E., Saravitz, C., Sederoff, H., Ade, H. W., and O’Connor, B. T. (2020). Achieving Net Zero Energy Greenhouses by Integrating Semitransparent Organic Solar Cells. Joule 4 (2), 490–506. doi:10.1016/j.joule.2019.12.018
Rayhana, R., Xiao, G., and Liu, Z. (2020). Internet of Things Empowered Smart Greenhouse Farming. IEEE J. Radio Freq. Identif. 4 (3), 195–211. doi:10.1109/jrfid.2020.2984391
Raza, M. Q., and Khosravi, A. (2015). A Review on Artificial Intelligence Based Load Demand Forecasting Techniques for Smart Grid and Buildings. Renew. Sustain. Energ. Rev. 50, 1352–1372. doi:10.1016/j.rser.2015.04.065
Ren, W., and Cheng, H.-M. (2014). The Global Growth of Graphene. Nat. Nanotech 9 (10), 726–730. doi:10.1038/nnano.2014.229
Renewable Energy Hub (2020). Solar Panels for Greenhouses. Available at: https://www.renewableenergyhub.co.uk/main/solar-panels/solar-panels-for-greenhouses/.
Ronchi, E., Corbetta, A., Galea, E. R., Kinateder, M., Kuligowski, E., McGrath, D., et al. (2019). New Approaches to Evacuation Modelling for Fire Safety Engineering Applications. Fire Saf. J. 106, 197–209. doi:10.1016/j.firesaf.2019.05.002
Saeidi, S., Rezaei, B., Irannejad, N., and Ensafi, A. A. (2020). Efficiency Improvement of Luminescent Solar Concentrators Using Upconversion Nitrogen-Doped Graphene Quantum Dots. J. Power Sourc. 476 (January), 228647. doi:10.1016/j.jpowsour.2020.228647
Sagheer, A., Mohammed, M., Riad, K., and Alhajhoj, M. (2020). A Cloud-Based IoT Platform for Precision Control of Soilless Greenhouse Cultivation. Sensors 21 (1), 223. doi:10.3390/s21010223
Saini, V., Tiwari, S., and Tiwari, G. N. (2017). Environ Economic Analysis of Various Types of Photovoltaic Technologies Integrated with Greenhouse Solar Drying System. J. Clean. Prod. 156, 30–40. doi:10.1016/j.jclepro.2017.04.044
Salerno, I., Anjos, M. F., McKinnon, K., and Gómez-Herrera, J. A. (2021). Adaptable Energy Management System for Smart Buildings. J. Building Eng. 44, 102748. doi:10.1016/j.jobe.2021.102748
Scognamiglio, A. (2017). “Building-Integrated Photovoltaics (BIPV) for Cost-Effective Energy-Efficient Retrofitting,” in Cost-Effective Energy Efficient Building Retrofitting Kurnitski. Editors F. Pacheco-Torgal, C-G. Granqvist, B. P. Jelle, G. P. Vanoli, N. Bianco, and B. T. Jarek (Sawston, United Kingdom: Woodhead Publishing), 169–197. doi:10.1016/B978-0-08-101128-7.00006-X
Shamshiri, R. R., Hameed, I. A., Thorp, K. R., Balasundram, S. K., Shafian, S., Fatemieh, M., et al. (2020). “Greenhouse Automation Using Wireless Sensors and IoT Instruments Integrated with Artificial Intelligence,” in Next-Generation Greenhouses for Food Security (London, UK: Intechopen), 1–20.
Shankar, A., Vijayakumar, K., and Babu, B. C. (2021). Energy Saving Potential through Artificial Lighting System in PV Integrated Smart Buildings. J. Building Eng. 43, 103080. doi:10.1016/j.jobe.2021.103080
Shi, H., Xia, R., Zhang, G., Yip, H.-L., and Cao, Y. (2019). Spectral Engineering of Semitransparent Polymer Solar Cells for Greenhouse Applications. Adv. Energ. Mater. 9 (5), 1803438. doi:10.1002/aenm.201803438
Srinivasan, G., and Muthukumar, P. (2021). A Review on Solar Greenhouse Dryer: Design, thermal Modelling, Energy, Economic and Environmental Aspects. Solar Energy. doi:10.1016/j.solener.2021.04.058
Su, L.-c., Wu, X., Zhang, X., and Huang, X. (2021). Smart Performance-Based Design for Building Fire Safety: Prediction of Smoke Motion via AI. J. Building Eng. 43 (January), 102529. doi:10.1016/j.jobe.2021.102529
Syafarinda, Y., Akhadin, F., Fitri, Z. E., Widiawan, Y. B., and Rosdiana, E. (2018). The Precision Agriculture Based on Wireless Sensor Network with MQTT Protocol. IOP Conf. Ser. Earth Environ. Sci. 207 (1), 012059–9. doi:10.1088/1755-1315/207/1/012059
Thomopoulos, V., Bitas, D., Papastavros, K.-N., Tsipianitis, D., and Kavga, A. (2021). Development of an Integrated IoT-Based Greenhouse Control Three-Device Robotic System. Agronomy 11 (2), 405–416. doi:10.3390/agronomy11020405
Thompson, B. C., and Fréchet, J. M. J. (2008). Polymer-Fullerene Composite Solar Cells. Angew. Chem. Int. Ed. 47 (1), 58–77. doi:10.1002/anie.200702506
Touhami, A., Benahmed, K., Parra, L., Bounaama, F., and Lloret, J. (2020). An Intelligent Monitoring of Greenhouse Using Wireless Sensor Networks. Smart Structures Syst. 26 (1), 117–134. doi:10.12989/sss.2020.26.1.117
Trypanagnostopoulos, G., Kavga, A., Souliotis, Μ., and Tripanagnostopoulos, Y. (2017). Greenhouse Performance Results for Roof Installed Photovoltaics. Renew. Energ. 111, 724–731. doi:10.1016/j.renene.2017.04.066
Villacorta, E., Haraldseid, I., Mikalsen, R. F., Hagen, B. C., Erland, S., Kleppe, G., et al. (2021). Onset of Smoldering Fires in Storage Silos: Susceptibility to Design, Scenario, and Material Parameters. Fuel 284, 118964. doi:10.1016/j.fuel.2020.118964
Wan, X., Zhang, F., Liu, Y., and Leng, J. (2019). CNT-based Electro-Responsive Shape Memory Functionalized 3D Printed Nanocomposites for Liquid Sensors. Carbon 155, 77–87. doi:10.1016/j.carbon.2019.08.047
Wang, D., Liu, H., Li, Y., Zhou, G., Zhan, L., Zhu, H., et al. (2021). High-Performance and Eco-Friendly Semitransparent Organic Solar Cells for Greenhouse Applications. Joule 5 (4), 945–957. doi:10.1016/j.joule.2021.02.010
Wang, Z., Wang, X., Cong, S., Geng, F., and Zhao, Z. (2020). Fusing Electrochromic Technology with Other Advanced Technologies: A New Roadmap for Future Development. Mater. Sci. Eng. R: Rep. 140, 100524–100526. doi:10.1016/j.mser.2019.100524
Wu, G., Yang, Q., Zhang, Y., Fang, H., Feng, C., and Zheng, H. (2020). Energy and Optical Analysis of Photovoltaic Thermal Integrated with Rotary Linear Curved Fresnel Lens inside a Chinese Solar Greenhouse. Energy 197, 117215. doi:10.1016/j.energy.2020.117215
Wyatt, P. J. (2021). Differential Light Scattering and the Measurement of Molecules and Nanoparticles: A Review. Analytica Chim. Acta X 7-8, 100070. doi:10.1016/j.acax.2021.100070
Xia, Y., Xu, X., and Inganäs, O. (2019). Photovoltage Loss in Semi-transparent Organic Photovoltaic Devices. Org. Electron. 74 (March), 37–40. doi:10.1016/j.orgel.2019.06.051
Yan, S., Fazilati, M. A., Toghraie, D., Khalili, M., and Karimipour, A. (2021). Energy Cost and Efficiency Analysis of Greenhouse Heating System Enhancement Using Phase Change Material: An Experimental Study. Renew. Energ. 170, 81. doi:10.1016/j.renene.2021.01.081
Yang, J., Guo, Y., Jiang, R., Qin, F., Zhang, H., Lu, W., et al. (2018). High-Efficiency "Working-In-Tandem" Nitrogen Photofixation Achieved by Assembling Plasmonic Gold Nanocrystals on Ultrathin Titania Nanosheets. J. Am. Chem. Soc. 140 (27), 8497–8508. doi:10.1021/jacs.8b03537
Yang, L., Entchev, E., Rosato, A., and Sibilio, S. (2017). Smart Thermal Grid with Integration of Distributed and Centralized Solar Energy Systems. Energy 122, 471–481. doi:10.1016/j.energy.2017.01.114
Zhan, W., and Jiang, K. (2008). A Modular Photocurrent Generation System Based on Phospholipid-Assembled Fullerenes. Langmuir 24 (23), 13258–13261. doi:10.1021/la802972p
Zhang, K., Lv, H., Zheng, Y., Yao, Y., Li, X., Yu, J., et al. (2021). Nanofibrous Hydrogels Embedded with Phase-Change Materials: Temperature-Responsive Dressings for Accelerating Skin Wound Healing. Composites Commun. 25 (April), 100752. doi:10.1016/j.coco.2021.100752
Zhang, Y., Geng, P., Sivaparthipan, C. B., and Muthu, B. A. (2021). Big Data and Artificial Intelligence Based Early Risk Warning System of Fire Hazard for Smart Cities. Sustain. Energ. Tech. Assessments 45, 100986. doi:10.1016/j.seta.2020.100986
Keywords: greenhouses, smart and intelligent materials, photovoltaics, solar cells, internet of things
Citation: Maraveas C, Loukatos D, Bartzanas T, Arvanitis KG and Uijterwaal JF (2021) Smart and Solar Greenhouse Covers: Recent Developments and Future Perspectives. Front. Energy Res. 9:783587. doi: 10.3389/fenrg.2021.783587
Received: 26 September 2021; Accepted: 01 November 2021;
Published: 17 November 2021.
Edited by:
Shiva Gorjian, Tarbiat Modares University, IranReviewed by:
Macmanus Ndukwu, Michael Okpara University of Agriculture, NigeriaDaniel Tudor Cotfas, Transilvania University of Brașov, Romania
Muslum Arici, Kocaeli University, Turkey
Copyright © 2021 Maraveas, Loukatos, Bartzanas, Arvanitis and Uijterwaal. This is an open-access article distributed under the terms of the Creative Commons Attribution License (CC BY). The use, distribution or reproduction in other forums is permitted, provided the original author(s) and the copyright owner(s) are credited and that the original publication in this journal is cited, in accordance with accepted academic practice. No use, distribution or reproduction is permitted which does not comply with these terms.
*Correspondence: Konstantinos G. Arvanitis, a2FydmFuQGF1YS5ncg==