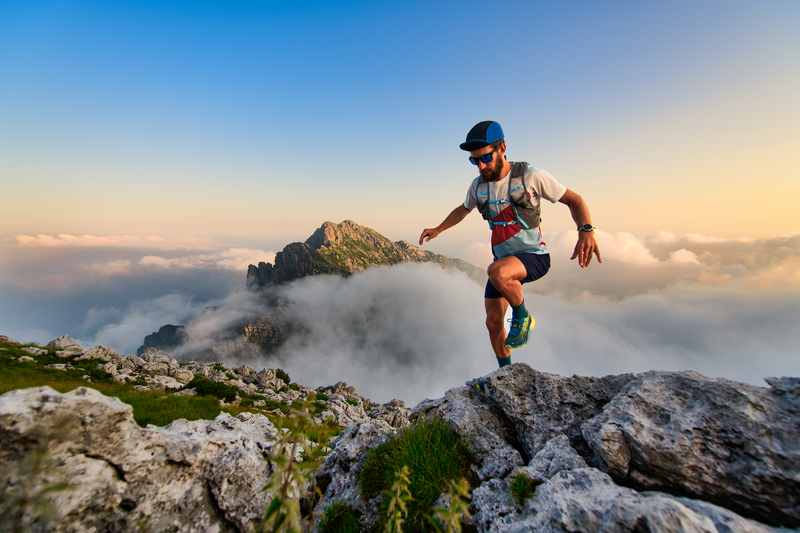
95% of researchers rate our articles as excellent or good
Learn more about the work of our research integrity team to safeguard the quality of each article we publish.
Find out more
ORIGINAL RESEARCH article
Front. Energy Res. , 23 November 2021
Sec. Solar Energy
Volume 9 - 2021 | https://doi.org/10.3389/fenrg.2021.776821
This article is part of the Research Topic Advances in Solar Central Receiver Technology View all 4 articles
Concentrated solar power (CSP) systems are regarded as a renewable energy source technology that can contribute to decoupling the energy mix from fossil fuel combustion and related environmental impacts. However, current small-scale CSP technologies (e.g., Dish-Stirling) have not entered the market yet due to high costs, complexity, and poor reliability. The EU-funded OMSoP (Optimised Microturbine Solar Power) project aimed at solving the small-scale CSP shortcomings by coupling a solar dish with the consolidated and relatively cheap technology of the micro gas turbine (MGT). In this study, an environmental life cycle assessment analysis of the production and operation of a CSP-MGT system is performed following an eco-design approach, thus identifying the environmental hotspots and how the system can be improved in terms of environmental impacts. The results of the analysis, per unit of electricity produced, were compared to other renewable technologies with the same level of dispatchability to better evaluate strengths and weaknesses of the system under exam. With regard to climate change, the greenhouse gas (GHG) emissions of the CSP-MGT system resulted in the same range as those generated by photovoltaic systems. However, the system can substantially be optimized and the GHG emissions per kWh can be reduced up to 73% with respect to the built prototype. The GHG emissions are much lower than the current Italian energy mix (by up to 94%). To reduce the environmental burden of CSP-MGT plants, the system design here considered should be revised by improving the component’s performance and significantly reducing the reflective surface and therefore the structural materials for the dish foundation and frame. The replacement of steel in the dish frame with aluminum increases all the environmental impact parameters and primary energy demand (17%–27% depending on the environmental category considered) but slightly reduces abiotic element depletion (by 9%).
The surging of the global concern on anthropogenic climate impacts has resulted in the prioritization of renewable energy systems that can support the decarbonization of our economies (IPCC, 2014). This transition has fundamentally modified the traditional decision-making approach in the energy sector. While, in the past, this approach was mostly based on socioeconomic aspects, nowadays, one of the main priorities relies on greenhouse gas (GHG) emission reduction (European Union, 2009; European Commission, 2015).
The 2009 Renewable Energy Directive (RED) was revised in December 2018 (REDII) and adopted as part of the Clean energy for all European package (European Union, 2018). It includes a new binding renewable energy target for 2030 of at least 32%, with a clause for a possible upward revision by 2023. Furthermore, the European Green Deal (European Union, 2019) 1, which sets the new European Climate and Energy Strategy, outlines a number of initiatives across all policy sectors, aimed at making EU climate neutral by 2050. In particular, compared to previous actions [COM (2018)773], the European Green Deal has raised the goal of reducing CO2 emissions by 2030 from 40% to 55% and has updated the programs already launched by the previous Commissions to make renewable energy, synergistically with energy efficiency, as the fundamental pillars to reach the ambitious goal of climate neutrality by 2050.
Renewable electricity is playing a pivotal role in reaching these targets. However, renewable power plants do not come free of GHG emissions, and often trade-offs with other environmental impacts are overlooked, especially for bioenergy (Agostini et al., 2020). Even though the environmental impacts of renewables, other than bioenergy, are normally much lower than fossil fuels, the assessment of the environmental impacts associated with electricity production from renewables plays a key role in supporting the development of a sustainable future energy system (Intergovernmental Panel on Climate Change, 2014).
The underpinning concept is that, generally, the emission associated with fossil energy sources occurs all along the production chain, with the most contributing process being the operation phase, while renewable systems based on solar and wind cause very limited impacts during operation. Bioenergy, for many aspects, has similar impacts as fossil fuel at the point of combustion and, in addition, often cause higher impacts in the production phase (Agostini et al., 2015; Giuntoli et al., 2015; Czyrnek-Delêtre et al., 2017; Serra et al., 2017). In wind and solar facilities, the most significant environmental impact is associated with the construction, transportation, and dismantling phases of power stations and equipment (Bravo et al., 2012).
Concentrated solar power (CSP) technologies, which provide a key supporting technology to the penetration of renewables into the energy mix, are suitable for power generation and cogeneration both at large scale (linear/Fresnel or solar tower systems) and small scale (solar dish systems usually coupled to Stirling engines). Large-scale concentrating solar systems, which are known to benefit from significant economies of scale (Sanchez et al., 2011), are currently an established technology with a total installed capacity of over 5.5 GWe globally (CSP Project Around the World, 2021). On the other hand, small-scale concentrating solar systems, with capacities ranging from a few kW to a few MW, still represent a niche technology in the distributed electricity generation market, as they have to compete with the low cost, ease of operation, and reliability of the photovoltaic technology (Sánchez et al., 2016), despite their capacity of producing heat that can enable the on-demand electricity generation through thermal storage. Even in the case of parabolic technology coupled to Stirling engines, the high operating and maintenance costs of the engine and the low efficiency lead to a final cost of electricity still higher than 0.30–0.35 USD/kWh (Sánchez et al., 2016).
In this regard, the EU-funded OMSoP (Optimised Microturbine Solar Power) project (Lanchi et al., 2015), aimed at overcoming the limitations of the solar dish-Stirling technology by replacing the Stirling engine with a micro gas turbine (MGT), which is a simple, mature, and relatively cheap component.
The OMSoP demonstrative plant, located in Rome, at the ENEA Casaccia Research Centre (Lanchi et al., 2015), is composed of a circular parabolic concentrator (solar dish, Figure 1, point 1) that reflects the parallel solar rays and converge them to the focal point, where a solar receiver is located (Figure 1 point 2 and Figure 2). The solar dish consists of a reflecting surface supported by a metallic frame that moves throughout the day following the Sun on two rotation axes, thanks to an automatic tracking system (Figure 1 points 4 and 5). The solar dish and frame are supported by a pole made of steel, anchored on the ground through a concrete platform (foundation) (Figure 1 point 3); the reflecting surface is shaped to redirect the sunlight toward a solar receiver.
FIGURE 1. Scheme of the solar dish, 1) reflective surface, 2) supporting arm, 3) supporting pole, 4) centering slats, 5) central slats support box.
The solar receiver absorbs the concentrated radiation and converts it into the sensible heat that is transferred to the working fluid (air), which is then sent to the MGT system to produce electricity. The MGT transforms the high pressure of the working fluid into rotational energy and then into electrical power through a generator directly connected to it (OMSoP: D1.5—Optimized dish design, 2015).
Figure 3 shows a schematic of the plant configuration. The main feature of the OMSoP system is the replacement of the Stirling engine with the MGT technology. The purpose of this configuration is to increase the system reliability and operability in relation to short-term fluctuations of the solar energy, as MGTs are characterized by a very low mechanical inertia (Iaria et al., 2017). The replacement of the Stirling engine with the MGTs, which is largely deployed in the automotive sector, is also aimed at obtaining a reliable, economical, and efficient system, eliminating the presence of flammable and expensive process fluids (e.g., H2) and high pressure levels in the circuit.
Regarding the environmental performances of CSP systems, a limited number of studies are available in literature. A short summary of the available data, concerning GHG emissions, is reported in Table 1.
TABLE 1. Results of other studies on greenhouse gas (GHG) emissions from concentrated solar power (CSP) plants.
In a review carried out by NREL (Burkhardt et al., 2012), the authors found that, of the 125 references reviewed, 10 produced 36 independent GHG emission estimates passing screens for quality and relevance: 19 for parabolic trough technology and 17 for power tower technology. The median estimates were 26 and 38 g CO2-eq/kWh for trough and tower CSP, respectively. However, the interquartile range (IQR) of published estimates for troughs and towers was 83 and 20 g CO2-eq/kWh, respectively. Kommalapati et al. (2017) performed an extensive review of life cycle assessment (LCA) studies on GHG emissions from different types of photovoltaics (PV) and mechanism-based CSP electricity generation systems. They identified five different categories for CSP systems: parabolic trough, central receiver, paraboloidal dish, solar chimney, and solar pond. The average GHG emission estimates resulted as 73 ± 61, 94 ± 75, 41 ± 17, 36 ± 22, 6 ± 1 g CO2-eq/kWh, respectively.
The environmental performances are fundamental to define the priorities of future energy systems based on renewables. In particular, in the case of small CSP systems, the comparison should be carried out with other sources of power providing the same function, i.e., non dispatchable power such as PV. It is noticeable that the Dish-MGT system, thanks to the thermal inertia of the receiver, is intrinsically provided with a thermal buffer compensating short-term solar radiation fluctuations and stabilizing the electrical output. Table 2 reports further technical details on the solar dish.
TABLE 2. Dish characteristics, from OMSoP: D1.5–Optimized dish design, 2015.
There is a wide consensus in the scientific community on the application of the LCA approach for the evaluation of the environmental impacts of products and services (European Commission—Joint Research Centre-Institute for Environment and Sustainability, 2010).
According to the International Standardization Organization (ISO), LCA is a structured and internationally standardized method to quantify all relevant emissions and resources consumed, the related environmental and health impacts, and the resource depletion issues that are associated with the entire life cycle of goods or services (“products”) (ISO 14040:2006, 2006; ISO 14044:2006, 2006).
In the present work, the LCA methodology was applied to assess the environmental performances of the OMSoP demonstrative plant installed at the ENEA Casaccia Research Centre in Rome (Italy).
The work is a comparative attributional LCA study aimed at identifying the environmental burden associated with the electricity generation through a small-scale CSP system based on the coupling of a solar dish with a commercial MGT. Through an eco-design approach, we aim at identifying the environmental hotspots and how the system can be improved in order to reduce environmental impacts.
The results are then compared to a PV system, and to the current electricity mix, to provide the reader with a clearer understanding of the results.
This work applies the LCA methodology to assess the environmental impacts of the Dish-MGT technology developed within the EU OMSoP project. LCA, according to the international standard (ISO 14040:2006, 2006; ISO 14044:2006, 2006), is structured in four phases: goal and scope definition; Life Cycle Inventory (LCI); Life Cycle Impact Assessment (LCIA), and interpretation. The first three phases constitute the subsections of this section, while the interpretation is reported in the Discussion and Conclusions section.
The main goal of this study is to identify the potential environmental impacts associated with the Dish-MGT technology. Particularly, the aim is 3-fold: to quantify the environmental impacts associated with the actual system built within the project framework, to calculate the impacts associated with an optimized version of the current technology, and to propose possible improvements with alternative materials. The approach is attributional and comparative. The results also will be compared with a system providing the same function, also in terms of dispatchability (PV), and with the Italian electricity mix to facilitate the understanding of the results.
The lifetime of the system is assumed to be 25 years.
The system function is the production of electrical energy, and the functional unit is 1 kWh.
In addition to the OMSoP system actually built at the ENEA Casaccia site, four additional scenarios have been modeled to evaluate the environmental performances of optimized systems and the opportunity of using alternative materials.
The five systems modeled are the following:
• OMSoP 1: the actual demonstrative plant as it was designed and built with a dish surface oversized to ensure a margin of flexibility for future follow-up of the project.
• OMSoP 2: optimized system in terms of component size; in particular, a turbine of 10 kW in place of a 5 kW is adopted to fully exploit the dish capacity; the turbine size was increased by 20% in terms of mass.
• OMSoP 3: materials, design, and manufacturing optimized: the starting reference is OMSoP 2, but the materials and the infrastructures are improved, leading to a 30% reduction in the reflective surface, which allows for a mass reduction of the whole system (up to 30%, depending on the specific subsystem).
• OMSoP 4: further engineering improvement and material optimization: the starting reference is OMSoP 3 but further improvements led to a mass reduction for the whole system up to 50%, depending on the specific subsystem.
• OMSoP 5: the starting reference is OMSoP 4, but the steel in the dish frame is considered to be replaced with aluminum.
The LCA study was carried out using Gabi software (GaBi Software 10.5, 2021), and the results were analyzed according to international standards (ISO 14040:2006, 2006; ISO 14044:2006, 2006) and recommendations (European Commission-Joint Research Centre, 2010; Fazio et al., 2018).
The boundaries of the assessment were set at cradle to gate. The phases of transportation, construction, and operation of the plant were analyzed. The end of life of the plant and the use of the electricity produced were not considered. However, it should be noted that most of the materials can be recycled or reused.
Following an eco-design approach, the work was aimed at supporting the system optimization and the assessment of the environmental burden associated with the Dish-MGT plant. By carrying out a contribution analysis on the most important aspects impacting the environmental performances of the OMSoP system, we aim at identifying the environmental hotspots and the best materials and components. All the input and flows have been modeled as accurately as possible (i.e., we have not applied a predefined cutoff rule).
In the LCIA phase, the following impact categories were considered relevant and therefore have been analyzed: global warming potential (GWP), ozone depletion potential (ODP), acidification potential (AP), eutrophication potential (EP), photochemical ozone creation potential (POCP), and abiotic depletion potential (elements) (ADP-E).
Other environmental impact categories were not analyzed because they were considered less significant or because the impact assessment method is considered immature and, therefore, not recommended (European Commission—Joint Research Centre, 2010; Fazio et al., 2018). The impact assessment methods used are those recommended by the ILCD handbook or, for consistency, the methods used in the environmental product declaration (EPD) of the components (in this case ALMIRR®).
Moreover, to better understand the performances of this energy system, the primary energy demand from non-renewables (PED) is also analyzed.
LCI involves a systematic inventory of the input and output of energy and material flows during the entire life cycle. All data have been gathered in a spreadsheet in order to build a comprehensive list of all processes and materials/components included in the construction of the system. We then coupled our data inventory with the most similar technology/process/material datasets available in the commercially available background data database Ecoinvent (Ecoinvent, 2020) and built the model of the five systems analyzed.
The systems were split in subsystems to allow for an improved understanding of the parameters most impacting the environmental performances of the OMSoP system: the receiver and the MGT (power system), the frame, the reflective surface (Almirr®), and the foundation of the solar dish.
The subdivision in subsystems of the infrastructure is represented in Figure 4, including a representation of the input and output considered for the evaluation of environmental performance of the system.
FIGURE 4. Scheme of the subsystems mass contribution in the demonstrative plant including the types of input and output of the environmental life cycle assessment (LCA) analysis performed.
In the actual CSP demo plant (OMSoP 1), the solar dish, also called concentrator, is composed of two circular crowns of steel sections; it supports and shapes the parabolic reflective surface. The reflective surface (Almirr®) consists of a wafer of two aluminum sheets and a plastic core polymeric resin (OMSoP: D1.5 – Optimized dish design, 2015). The dish is supported by a metallic structure, the frame, made of steel sections connected to a central steel pole, fixed to the ground through a concrete platform, the foundation, to provide the system with the necessary mechanical stability. The total area of the dish is 108.1 m2. The supporting pole is about 6 m high. The concrete base is approximately 15.54 tons. The MGT nominal power is 5 kW.
The 5-kW MGT coupled with the OMSoP solar dish is estimated to produce 7.72 MWh electric energy per year, referring to the geographical location of the ENEA Casaccia site and to the real operating condition reported in the first column of Table 3. The nominal operating conditions initially assumed for the design of the system are reported in the second column of Table 3. In the real system, the optical efficiency of the dish was quite low, about 40% (OMSoP: D2.2–Technical Report on Steady State simulations, 2015) mainly due to a limited quality of the dish manufacturing and assembling (OMSoP: D1.6 Report on Solar Dish Performance, 2015), and the calculated thermodynamic cycle efficiency (intended as the ratio of the electrical power produced to the net power absorbed by the receiver) is about 20%, considering a turbine inlet temperature (TIT) of 750°C and a compressor pressure ratio of about 2.6 (Table 3) (OMSoP: D1.4—Report on solar dish selected materials, 2015). The Total Weight is 21,566 kg.
TABLE 3. Cycle operating conditions (OMSoP: D2.2–Technical Report on Steady State Simulations, 2015).
Given the design and the structure of the system, most of the materials used in the demonstrative plant were available in the LCA database. The system is mostly made by concrete, steel, and other metals. For the Almirr®, which is not included in commercial databases, the producer, Larson, has provided the EPD according to ISO 14025:2006, 2006. The transport of all components has been included as well. Concrete was assumed to be transported for 50 km; all the other components are assumed to be transported for 100 km.
Some of the assembly processes, e.g., mounting on-site, and maintenance are not considered in the analysis. Their quantification, besides being very difficult and inaccurate, can be considered negligible for energy systems.
The main bottlenecks of the demonstrative plant (OMSoP 1) are related to the oversizing of the dish in relation to the size of the MGT and its limited optical efficiency. Assuming for the OMSoP dish a state-of-the-art overall optical efficiency of 0.8, and a thermodynamic cycle efficiency of 0.2, the system could power an MGT of about 10 kW, increasing the annual electrical production from 7.72 MWh up to 18.3 MWh (OMSoP 2). In this scenario, it is assumed a reduction of the dish shape errors related to the manufacturing and assembly operations and the use of a bigger MGT (with a rated output of 10 kW and 20% heavier) with the same design. The total weight is 21,582 g, and the total annual electricity production is 18.3 MWh.
In the OMSoP 3 scenario, an optimization of the materials, design, and manufacturing allows for a decrease of the reflecting surface of 30%, with a consequent reduction of materials for the foundation and frame (20% for the arm supporting the power system and the tracking system). This system relies on high-performance materials and nominal operating conditions (turbine pressure ratio: 3, TIT: 800°C; Table 3) that leads to a thermodynamic cycle efficiency of 24%. Its total weight is 14,981 kg. The turbine and power production are the same as those of OMSoP 2.
OMSoP 4 is similar to OMSoP 3, but the materials, design, and manufacturing of the frame and foundation are further optimized, increasing the overall optical efficiency to 85% and the energy efficiency conversion to 30% (upgraded cycle with TIT of 900°C and recuperator effectiveness of 90% in place of 85%, allowing for a mass reduction of 50% of the materials for the foundation, the dish-supporting pole, and structure, while all the remaining parts are as in OMSoP 3 scenario. The total weight is 11,874 kg.
OMSoP 5 scenario is similar to OMSoP 4 configuration, but the steel of the solar dish frame is replaced with aluminum sandwich panels. The resulting total weight is 10,708 kg.
In the Supplementary Material, Tables with a detailed inventory of all the systems analyzed are reported.
The materials and energy flows identified in the LCI phase were categorized and assigned to the relevant impact categories using the commercial software GaBi. The evaluation is at mid-point (i.e., the emission to the environment is quantified, not the impact on the end point). The assessment methods adopted are, where possible, the ILCD (European Commission - Joint Research Centre - Institute for Environment and Sustainability, 2011)-recommended methods.
For some environmental impact categories (ADP-E and EP), the same EPD method of Almirr mirror was adopted to have consistent LCI data.
Seven impact categories were considered, as listed below (in brackets if the method differs from the ILCD recommendation):
• Global warming potential-GWP100 IPCC AR5
• Primary energy demand (technical quantity) from non-renewables (PED)
• Ozone depletion potential (ODP)—Environmental Footprint 3.0
• Acidification potential (AP)—Environmental Footprint 3.0
• Eutrophication potential (EP) (CML2001—January 2016)
• Photochemical ozone creation potential (POCP)—Environmental Footprint 3.0
• Abiotic depletion potential: elements (ADP-E) (CML2001—January 2016)
The metric used to measure climate change is GWP with 100 years’ time frame. The reference substance for this impact category is CO2, and the reference unit is kg CO2–equivalent (kg CO2eq). All emissions of well-mixed GHGs that contribute to global warming are converted to mass of CO2eq according with the characterization factors deriving from the IPCC AR5 (Intergovernmental Panel on Climate Change, 2014).
Figure 5 shows the GHG emissions of the modeled systems. The GHG emissions of OMSoP 1 are already lower by 86% than the Italian electricity mix. They are, however, similar to the competing technology, i.e., PV. By replacing the MGT with a turbine correctly sized and increasing the dish optical efficiency, the emissions become lower than those of PV and through the optimized design of the system, the emissions can become 75% lower with respect to the built prototype. The substitution of steel with aluminum in the frame slightly increases GHG emissions: the GHG emissions associated with the frame increase from 0.0175 to 0.019 kg CO2eq in OMSoP 4 and OMSoP 5, respectively.
FIGURE 5. Greenhouse gas (GHG) emissions (A) of the modeled systems and photovoltaics (PV) and electricity grid mix systems shown for comparison; contribution analysis (B).
As shown by the contributional analysis (Figure 5), the subsystem most contributing to the emissions is, in OMSoP 1, the metallic structure (frame) sustaining the reflective surface (47%), followed by the foundations (24%), the mirrors (16%), and the receiver and the power system, both contributing about 12% to the total GHG emissions. For the other systems, the contribution of the subsystems is similar with the contribution of the foundation and frame decreasing with the increase of the total efficiency, which allows for a smaller dish.
The contribution of the subsystems is directly related to their mass, in particular to the amount of steel and concrete used for the construction of the plant.
Since the function of the OMSoP technology is to produce electricity, besides the ADP-E, we have investigated the PED. This approach also allows using the data from the EPD of the Larson products (Almirr), which were reported with the same method. With regard to the PED, Figure 7 shows that all the renewables clearly perform by far much better than the Italian electricity mix, although the mix includes an important share of renewables.
Figure 6 shows also that, obviously, the electricity mix requires more primary energy from fossils than the electricity produced, since the conversion efficiencies and the upstream emissions to produce the fuel have to be considered. It is noticeable that the use of aluminum (in OMSoP 5) for the dish in place of steel increases the PED by 26% (OMSoP 4 vs. OMSoP 5) due to the high energy demand of aluminum production. The PED of PV systems is higher than that of the OMSoP systems; this is likely due to either the high energy requirements of silicon and aluminum processing or the more complete inventory of the PV commercial products, or a combination of the two factors. OMSoP 1 presents a PED more than 3-fold with respect to the other OMSoP systems on average, exhibiting it is far from being optimized.
FIGURE 6. Primary energy demand from non-renewables (A) of the modeled systems and contribution analysis (B).
The PED contribution analysis (Figure 6) confirms that most of the environmental burden of the OMSoP system lays on the large use of steel and concrete for the frame and foundation.
The remaining environmental impacts are reported in Figure 7.
The POCP for the systems with the correctly sized MGT (all but OMSoP 1) is in the range 5%–16% of the impacts of the Italian electricity mix and less than half of the PV. Again, as shown by the contribution analysis, it is the large quantity of steel and concrete used for the supporting structure that impacts the most on the ozone precursor emissions.
The OMSoP system with a properly sized turbine results in emissions impacting the nutrient cycle (eutrophication potential) of about 10% of those of the electricity mix, with the steel frame contributing the most to the emissions. However, the emissions of the PV system are significantly higher than those of the OMSoP systems, being about half than the Italian electricity mix for this specific impact category. The replacement of steel with aluminum in OMSoP 5 does not reduce the emission, since they are, on the contrary, slightly increased.
The two impact categories ODP and AP mimic the EP emissions.
About the ODP, this impact category is related to the emission of chemical compounds that can alter the chemistry of the stratosphere and reduce the ozone layer that protects the Earth from the damaging UV light. The compounds are mostly chloro-fluoro-carbons, which, when used as solvent or refrigerant in the manufacturing of a product, may be released in the atmosphere and reach the stratosphere. For this impact category, the subsystem foundation is responsible for higher impacts. We identified a particular type of steel (Stainless steel Quarto plate 304) as responsible for the emissions most contributing to this impact parameter.
In the case of the ADP-E, the resource consumption of the OMSoP systems is of the same order of magnitude as the electricity mix, while the PV resource depletion is by far much higher due primarily to the use of silver and selenium for multicrystalline silicon PV. With regard to the OMSoP system, basically, the material responsible for this impact is steel, partly the steel in the foundation but mostly the steel in the frame. This depends on the fact that iron, but especially the other metals added to iron, which gives the needed strength and resistance to outdoor conditions, is rarer than the minerals used for concrete, aluminum, or silicon.
The scope of this study was to assess the environmental impact of the OMSoP Dish-MGT technology and to compare it with the current electricity mix and the main competing technology (PV) and to identify possible approaches to reduce it.
The LCA study has been carried out using GaBi software, and the results have been analyzed following the ISO and EC–ILCD recommendations.
The data quality can be considered good, since the most recent datasets from the commercial database Ecoinvent 3.6 (2020) were used for the background systems. However, the representativeness of the data is limited, as some materials used for the OMSoP plant were not present in the database and were replaced, in the analysis, by the most similar dataset available. We could however select proxy materials for almost the entire mass of the system.
A weakness of the whole analysis lies in the missed modeling of many processes needed for assembling and refining the systems (e.g., some electronic appliances and assembly of some parts of the plant). Nevertheless, it is quite reasonable to consider that a few kilograms of electronics and other relatively small items do not change dramatically the results, also taking into account that the system is a prototype.
About the comparison with PV, it has to be noted that in Ecoinvent database, the life expectancy of PV systems is set to 20 years.
Even with the limitations reported above, the assessment performed can be considered consistent with the scope of the study and robust enough to draw the following conclusions:
- The CSP technology under exam (solar dish coupled with MGT) performs much better than the Italian electricity mix and about the same of PV in terms of GHG emissions and PED of power production.
- For other environmental impact categories, the OMSoP system may contribute significantly to decreased emissions with respect to the Italian electricity mix and, although less pronounced, also to PV; however, it scores slightly worse than electricity mix for the ADP-E.
With regard to the reduction of the environmental impacts of the OMSoP system, the most important, and obvious, recommendations are to refine the system design, to improve the component’s performance, and to significantly limit the reflective surface and, consequently, the structural materials for the dish foundation and frame.
The replacement of steel in the dish frame with aluminum does not lead to a general environmental improvement, but to trade-offs among impact categories. It increases all the environmental impacts and PED but reduces the ADP-E.
Given the rapid penetration of non-dispatchable energy technologies in the energy market, further research should be aimed at assessing the environmental impacts of solar technologies with energy storage (e.g., PV with batteries; CSP with heat storage) to provide policymakers further information for a science-based energy policy planning.
The original contributions presented in the study are included in the article/Supplementary Material. Further inquiries can be directed to the corresponding author.
AA, ML, and VR: conceptualization and full responsibility over the article. AA: drafted the initial article. AA and CC: data analysis and preparation of tables and figures. AA, CC, ML, AM, MM, and VR: equal contribution to overview/editing of the article.
The research leading to these results has received funding from the European Union’s Seventh Framework Programme (FP7-ENERGY.2012.2.5.1), grant agreement no. 308952.
The authors declare that the research was conducted in the absence of any commercial or financial relationships that could be construed as a potential conflict of interest.
All claims expressed in this article are solely those of the authors and do not necessarily represent those of their affiliated organizations, or those of the publisher, the editors, and the reviewers. Any product that may be evaluated in this article, or claim that may be made by its manufacturer, is not guaranteed or endorsed by the publisher.
The Supplementary Material for this article can be found online at: https://www.frontiersin.org/articles/10.3389/fenrg.2021.776821/full#supplementary-material
ADP-E, abiotic depletion potential (elements); AP, acidification potential; CSP, concentrated solar power; EPD, environmental product declaration; EP, eutrophication potential; GHG, greenhouse gas; GWP, global warming potential; IPCC, Intergovernmental Panel on Climate Change; ISO, International Standardization Organization; LCA, life cycle assessment; LCI, Life Cycle Inventory; LCIA, Life Cycle Impact Assessment; MGT, micro gas turbine; ODP, ozone depletion potential; OMSoP, Optimised Microturbine Solar Power; POCP, photochemical ozone creation potential; PED, primary energy demand from non-renewables; PV, photovoltaics; RED, Renewable Energy Directive (2009); REDII, Renewable Energy Directive (2018); TIT, turbine inlet temperature
Agostini, A., Battini, F., Giuntoli, J., Tabaglio, V., Padella, M., Baxter, D., et al. (2015). Environmentally Sustainable Biogas? the Key Role of Manure Co-Digestion with Energy Crops. Energies 8, 5234–5265. doi:10.3390/en8065234
Agostini, A., Giuntoli, J., Marelli, L., and Amaducci, S. (2020). Flaws in the Interpretation Phase of Bioenergy LCA Fuel the Debate and Mislead Policymakers. Int. J. Life Cycle Assess. 25, 17–35. doi:10.1007/s11367-019-01654-2
Bravo, Y., Carvalho, M., Serra, L. M., Monné, C., Alonso, S., Moreno, F., et al. (2012). Environmental Evaluation of Dish-Stirling Technology for Power Generation. Solar Energy 86, 2811–2825. doi:10.1016/j.solener.2012.06.019
Burkhardt, J. J., Heath, G., and Cohen, E. (2012). Life Cycle Greenhouse Gas Emissions of Trough and Tower Concentrating Solar Power Electricity Generation. J. Ind. Ecol. 16, S93–S109. doi:10.1111/j.1530-9290.2012.00474.x
Corona, B., Cerrajero, E., López, D., and San Miguel, G. (2016). Full Environmental Life Cycle Cost Analysis of Concentrating Solar Power Technology: Contribution of Externalities to Overall Energy Costs. Solar Energy 135, 758–768. doi:10.1016/j.solener.2016.06.059
CSP Project Around the World (2021). [WWW Document]. Available at: https://www.solarpaces.org/csp-technologies/csp-projects-around-the-world. (Accessed on: August 01, 2021).
Czyrnek-Delêtre, M. M., Rocca, S., Agostini, A., Giuntoli, J., and Murphy, J. D. (2017). Life Cycle Assessment of Seaweed Biomethane, Generated from Seaweed Sourced from Integrated Multi-Trophic Aquaculture in Temperate Oceanic Climates. Appl. Energ. 196, 34–50. doi:10.1016/j.apenergy.2017.03.129
Desideri, U., Zepparelli, F., Morettini, V., and Garroni, E. (2013). Comparative Analysis of Concentrating Solar Power and Photovoltaic Technologies: Technical and Environmental Evaluations. Appl. Energ. 102, 765–784. doi:10.1016/j.apenergy.2012.08.033
Ecoinvent (2020). Ecoinvent Centre. EcoInvent v.3.3 Database, Available at https://ecoinvent.org/the-ecoinvent-database/. (Accessed on: August 01, 2021).
European Commission - Joint Research Centre - Institute for Environment and Sustainability (2010). International Reference Life Cycle Data System (ILCD) Handbook : Analysing of Existing Environmental Impact Assessment Methodologies for Use in Life Cycle Assessment. First edition. (Publications Office of the European Union). Cycle. doi:10.2788/38719
European Commission - Joint Research Centre - Institute for Environment and Sustainability (2011). International Reference Life Cycle Data System (ILCD) Handbook: Recommendations for Life Cycle Impact Assessment in the European Context - Based on Existing Environmental Impact Assessment Models and Factors. First edition. EUR 24571 EN (Luxemburg: Publications Office of the European Union).
European Commission (2015). COM(2015) 80 -A Framework Strategy for a Resilient Energy Union with a Forward-Looking Climate Change Policy. (Communication from the Commission to the European parliament).
European Union (2009). Directive 2009/28/EC on the Promotion of the Use of Energy from Renewable Sources and Amending and Subsequently Repealing Directives 2001/77/EC and 2003/30/EC. (Directive of the European Parliament).
European Union (2018). Directive (Eu) 2018/2001 of the European Parliament. (recast). (Directive of the European Parliament).
European Union (2019). The New Green Deal. COM, 640. final. (Communication from the Commission to the European parliament).
Fazio, S., Castellani, V., Sala, S., Schau, E. M., Secchi, M., Zampori, L., et al. (2018). Supporting Information to the Characterisation Factors of Recommended EF Life Cycle Impact Assessment Methods. doi:10.2760/671368
GaBi Software 10.5 (2021). [WWW Document]. Available at: https://gabi.sphera.com/italy/index/.
Giuntoli, J., Caserini, S., Marelli, L., Baxter, D., and Agostini, A. (2015). Domestic Heating from forest Logging Residues: Environmental Risks and Benefits. J. Clean. Prod. 99, 206–216. doi:10.1016/j.jclepro.2015.03.025
Iaria, D., Alzaili, J., and Sayma, A. I. (2017). Solar Dish Micro Gas Turbine Technology for Distributed Power GenerationNo Title, Sustainabl. Singapore: Springer.
Intergovernmental Panel on Climate Change (2014). Mitigation of Climate Change. doi:10.1017/cbo9781107415416
IPCC (2014). Contribution to the Fifth Assessment Report of the Intergovernmental Panel on Climate Change.
ISO 14025:2006 (2006). [WWW Document]. Available at: https://www.iso.org/standard/38131.html. (Accessed on: August 01, 2021).
ISO 14040:2006 (2006). [WWW Document]. Available at: https://www.iso.org/standard/37456.html. (Accessed on: August 01, 2021).
ISO 14044:2006 (2006). [WWW Document]. Available at: https://www.iso.org/standard/38498.html. (Accessed on: August 01, 2021).
Kommalapati, R., Kadiyala, A., Shahriar, M., and Huque, Z. (2017). Review of the Life Cycle Greenhouse Gas Emissions from Different Photovoltaic and Concentrating Solar Power Electricity Generation Systems. Energies 10 (3), 350. doi:10.3390/en10030350
Lanchi, M., Montecchi, M., Crescenzi, T., Mele, D., Miliozzi, A., Russo, V., et al. (2015). Investigation into the Coupling of Micro Gas Turbines with CSP Technology: OMSoP Project. Energ. Proced. 69, 1317–1326. doi:10.1016/j.egypro.2015.03.146
Mahlangu, N., and Thopil, G. A. (2018). Life Cycle Analysis of External Costs of a Parabolic Trough Concentrated Solar Power Plant. J. Clean. Prod. 195, 32–43. doi:10.1016/j.jclepro.2018.05.187
Norwood, Z., and Kammen, D. (2012). Life Cycle Analysis of Distributed Concentrating Solar Combined Heat and Power: Economics, Global Warming Potential and Water. Environ. Res. Lett. 7, 044016. doi:10.1088/1748-9326/7/4/044016
OMSoP D1.4 Report on solar dish selected materials (2015). OMSoP. [WWW Document]. Available at: https://etn.global/research-innovation/projects/omsop/#h2igcc-publications-and-reports. (Accessed on: August 01, 2021).
OMSoP: D1.5 Optimized dish design (2015). OMSoP. [WWW Document]. Available at: https://etn.global/research-innovation/projects/omsop/#h2igccpublications-and-reports. (Accessed on: August 01, 2021).
OMSoP: D1.6 Report on Solar Dish Performance (2015). OMSoP. [WWW Document]. Available at: https://etn.global/research-innovation/projects/omsop/#h2igcc-publications-and-reports. (Accessed on: August 01, 2021).
OMSoP: D2.2 Technical Report on Steady State simulations (2015). OMSoP. [WWW Document]. Available at: https://etn.global/research-innovation/projects/omsop/#h2igcc-publications-and-reports. (Accessed on: August 01, 2021).
Sánchez, D., Bortkiewicz, A., Rodríguez, J. M., Martínez, G. S., Gavagnin, G., and Sánchez, T. (2016). A Methodology to Identify Potential Markets for Small-Scale Solar Thermal Power Generators. Appl. Energ. 169, 287–300. doi:10.1016/j.apenergy.2016.01.114
Sanchez, D., Frej, H., Munoz de Escalona, M., Chacartegui, R., and Sanchez, T. (2011). “Alternative Approach to Determining the Preferred Plant Size of Parabolic Trough CSP Power Plantsitle,” in Turbo Expo: Power Dor Land, Sea, and Air, Vancouver, British Columbia, Canada, June 6–10, 2011, 1053–1062.
Keywords: solar dish, small-scale CSP, micro gas turbine (MGT), environmental impact, life cycle assessment (LCA)
Citation: Agostini A, Carbone C, Lanchi M, Miliozzi A, Misceo M and Russo V (2021) Environmental Impacts of a Solar Dish Coupled With a Micro-Gas Turbine for Power Generation. Front. Energy Res. 9:776821. doi: 10.3389/fenrg.2021.776821
Received: 14 September 2021; Accepted: 22 October 2021;
Published: 23 November 2021.
Edited by:
Loreto Valenzuela, Centro de Investigaciones Energéticas, Medioambientales y Tecnológicas, SpainReviewed by:
Runsheng Tang, Yunnan Normal University, ChinaCopyright © 2021 Agostini, Carbone, Lanchi, Miliozzi, Misceo and Russo. This is an open-access article distributed under the terms of the Creative Commons Attribution License (CC BY). The use, distribution or reproduction in other forums is permitted, provided the original author(s) and the copyright owner(s) are credited and that the original publication in this journal is cited, in accordance with accepted academic practice. No use, distribution or reproduction is permitted which does not comply with these terms.
*Correspondence: C. Carbone, Y2xhdWRpby5jYXJib25lQGVuZWEuaXQ=
Disclaimer: All claims expressed in this article are solely those of the authors and do not necessarily represent those of their affiliated organizations, or those of the publisher, the editors and the reviewers. Any product that may be evaluated in this article or claim that may be made by its manufacturer is not guaranteed or endorsed by the publisher.
Research integrity at Frontiers
Learn more about the work of our research integrity team to safeguard the quality of each article we publish.