- School of Energy Science and Engineering, Harbin Institute of Technology, Harbin, China
Replacing the oxygen evolution reaction (OER), which is of high energy consumption and slow kinetics, with the more thermodynamically favorable reaction at the anode can reduce the electricity consumption for hydrogen production. Here we developed a lignin-assisted water electrolysis (LAWE) process by using Ti/PbO2 with high OER overpotential as the anode aimed at decreasing the energy consumption for hydrogen production. The influence of key operating parameters such as temperature and lignin concentration on hydrogen production was analyzed. Compared with alkaline water electrolysis (AWE), the anode potential can be decreased from 0.773 to 0.303 (V vs. Hg/HgO) at 10 mA/cm2 in LAWE, and the corresponding cell voltage can be reduced by 546 mV. With increasing the temperature and lignin concentration, current density and H2 production rate were efficiently promoted. Furthermore, the anode deactivation was investigated by analyzing the linear sweep voltammetry (LSV) and cyclic voltammetry (CV) tests. Results showed that the anode deactivation was affected by the temperature.
Introduction
Hydrogen is an ideal secondary energy and energy carrier (Yang et al., 2015; Lu et al., 2019; Nairan et al., 2021). The calorific value of hydrogen is 142.3 MJ/kg, which is 3 times higher than that of oil, and the theoretical combustion production of hydrogen is only water. Hydrogen is regarded as one of the cleanest fuels without carbon emissions (Wang et al., 2021a; Wang et al., 2021b; Wang and Astruc, 2021). Compared with hydrogen production by using fossil fuels, hydrogen production by water electrolysis (WE) has various advantages such as high hydrogen production purity, simple and clean process (Hosseini et al., 2015; Vandyshev and Kulikov, 2017; Wang et al., 2020). Moreover, WE has capability to couple with renewable electricity to transform surplus and fluctuating electricity into stable hydrogen energy (Liu et al., 2020a; Zhao et al., 2021). Therefore, hydrogen is the most promising energy carrier to replace fossil fuels and is an important part of the future energy structure (Hu et al., 2018; Wang and Astruc, 2021).
The high electricity consumption of hydrogen production is the main obstacle limiting the large-scale application of water electrolysis (Yang et al., 2017; Sun et al., 2021; Qian et al., 2022). The theoretical voltage of hydrogen production by water electrolysis is 1.23 V at 25°C, and the power consumption is 2.94 kWh/Nm3 (H2) (Badwal et al., 2014). However, due to the existence of overpotential and internal resistance, and the actual operation must ensure a certain hydrogen production rate, so that the actual cell voltage reaches 2.0–2.2 V, and the actual power consumption is 4.5–5 kWh/Nm3, which is 50–70% higher than the theoretical value (Wang et al., 2014; Li et al., 2018). Anodic oxygen evolution reaction (OER) is a four-electron transfer process with slow reaction kinetics and high overpotential (Reier et al., 2017; Li et al., 2021; Ren et al., 2021; Yu et al., 2021). 90% of the electric consumption of water electrolysis comes from the OER reaction (Ju et al., 2018; Shan et al., 2019; Verma et al., 2019).
In the biomass-assisted water electrolysis system, the OER is replaced by the oxidation reaction of biomass, which can occur at a lower potential to achieve the purpose of reducing the electricity consumption of hydrogen production (Chen et al., 2014; Zhao et al., 2021). Ideally, organic molecules are oxidized to CO2, as shown in Eq. 1. The standard electrode potential of this reaction is 0.21 V, which is much lower than the anode potential required for the OER reaction (Yu et al., 2019). At present, a variety of substances have been used to reduce the electricity consumption for hydrogen production by assisting water electrolysis (Guo et al., 2011; Yu et al., 2018; Liu et al., 2020b). Among them, lignin is abundant and cost-effective, which has attracted the attention of researchers (Rinaldi et al., 2016). As the most abundant natural aromatic polymer, lignin is produced by plant growth up to 150 billion tons per year (Li et al., 2015; Du et al., 2020). However, lignin has not been efficiently used, which is mostly seen as low-rank fuel and pollutes the environment (Holade et al., 2020). In lignin-assisted water electrolysis, a new electrooxidation path was proposed. Lignin can be oxidized to low molecular weight aromatics (LMWA) which are high-value chemicals, such as vanillin, benzoic acid, and so on (Shao et al., 2014; Wang et al., 2015). Thus, apart from reducing the electricity consumption of hydrogen production, LAWE can also achieve the synergistic production of high-value chemicals (Song et al., 2019; Ghahremani et al., 2020). Mahtab et al. used NiCo/TiO2 as the anode to oxidize lignin in an anion-exchange membrane electrolytic cell (NaderiNasrabadi et al., 2019). It was found that the electrochemical oxidation of lignin was more favorable than the OER reaction at the lower cell voltage when the cell voltage was 1.4–1.6 V. Once OER occurred, the oxidation reaction of lignin is in direct competition with OER. Deng et al. used polyoxometalate and FeCl3 as the catalyst and charge-transfer agent to oxidize lignin (Du et al., 2017). With the LAWE, the electricity consumption could be 40% lower than the AWE.
In addition to precious metal or non-noble metal NiCo catalysts, cheap metal oxides such as PbO2 were used to electrooxidize lignin (Caravaca et al., 2019; NaderiNasrabadi et al., 2019). PbO2 has high conductivity, high catalytic oxidation capacity, and high chemical stability (Chai et al., 2014; Shao et al., 2014; Zhao et al., 2016). For example, Liang et al. used Ti/PbO2 as the anode to achieve electrochemical oxidation of lignin (Shao et al., 2014). By analyzing the CV curves, the Ti/PbO2 is a kind of active electrode, which means lignin degradation and OER on the anode share the same active sites. Besides, the Ti/PbO2 could efficiently degrade lignin into low molecular weight aromatic and the mechanism was investigated. Bateni et al. used β-PbO2/MWNT (multi-walled carbon nanotubes) as the anode, which improved the conversion rate of lignin and the rate of hydrogen evolution in the cathode, saving 20% energy compared with AWE for hydrogen production (Bateni et al., 2019). Then the mechanism of lignin oxidation with this electrode was analyzed (Bateni et al., 2021). When lignin was added to the solution, the oxidation peak was slightly shifted to the cathodic directions in the CV curves. Vanillin and methyl salicylate were found to be the main oxidation products.
Furthermore, the electrolysis temperature is a key factor for LAWE. At low temperatures, the conversion rate of lignin and the rate of hydrogen evolution reaction (HER) is generally low (Caravaca et al., 2019; Li et al., 2020). But both of them can be promoted evidently when the temperature is increased from ambient temperature to about 100°C (Zirbes and Quadri, 2020). Temperature can change the intrinsic reaction rate of the chemical reaction and the mass transfer rate of reactants/products, so it can have a great influence on the LAWE. Caravaca et al. used Pt/C as the cathode and Pt-Ru as the anode for LAWE (Caravaca et al., 2019). When the temperature increased from 30°C to 90°C, the current density generated at the same potential increased significantly, the hydrogen production rate increased, and the onset potential decreased significantly from 0.75 to 0.45 V. Hibino et al. investigated the cellulose-assisted water electrolysis to produce hydrogen at 75–150°C (Hibino et al., 2017). It was also found that with the increase of temperature, the onset potential of HER decreased and the current density increased. Higher temperature reduced the polarization resistance of the electrode and the ohm resistance of the system. The resulting electrolysis cell could realize the onset voltage at 0.25 V and the current density of 290 mA/cm2 at the cell voltage of only 1 V with the 100% current efficiency of HER.
This study aims to analyze the key operating parameters of the LAWE, such as the electrolysis temperature and the concentration of lignin. In this study, we constructed the anion exchange membrane H-type electrolysis cell to compare the LAWE with the AWE. Ti/PbO2 and Pt were used as anode and cathode, respectively. First, the electrocatalysis performance of the Ti/PbO2 anode was measured using the linear sweep voltammetry (LSV) and cyclic voltammetry (CV) techniques. The current density could be promoted at low cell voltage with the addition of lignin. With increasing the electrolysis temperature and lignin concentration, current density and H2 production rate can be efficiently promoted. At last, this work analyzed the anode deactivation mechanism by electrochemical tests.
Experimental
Chemicals and Materials
Reagents used in this paper: sodium hydroxide (Aladdin chemical reagent Co., Ltd., 99%), sodium lignosulfonate (Aladdin chemical reagent Co., Ltd., 98%). The water used in all experiments was deionized water purified by a Millipore Milli-Q device. The cathode was Pt electrode (1.5 cm × 1.5 cm). Ti/PbO2 electrode with the size of 3 cm × 2 cm was used as the anode, which was purchased from Xinfeng Technology Co., Ltd., and the area of a single titanium mesh hole was 0.25 cm2. The interval between the two electrodes was 6 cm. The anion exchange membrane was Fumapem FAA-3-50. Before use, the anion exchange membrane was soaked in 1 mol/L NaOH at room temperature for 24 h and then washed with deionized water. The electrochemical reaction device is an H-type electrolysis cell, and the volumes of the anode and cathode chamber were both 100 ml, shown in Figure 1.
Electrochemical Measurements
In all experiments, 85 ml of 1 mol/L NaOH solution was added to the anode and cathode chambers. The stirring rate was controlled at 800 r/min when carrying out chronoamperometry (CA) experiments. The linear sweep voltammetry (LSV) and cyclic voltammetry (CV) tests were carried out without stirring. Hg/HgO was used as the reference electrode. The electrochemical characteristics of the reaction were investigated by CA, LSV, and CV in a three-electrode or two-electrode system by electrochemical Workstation (Bio-Logic, VMP3). LSV was measured from 0 V vs. Hg/HgO to 1.0 V (control group 1.2 V) vs. Hg/HgO with a scan rate of 5 mV/s. The two-electrode system LSV has a measuring range from 0.6 to 1.8 V and a scan rate of 5 mV/s. CV is measured from 0 V vs. Hg/HgO to 1.0 V vs. Hg/HgO with a scan rate of 20 mV/s.
To reveal the deactivation mechanism of Ti/PbO2 anode, LSV curves were tested at 60°C under different conditions 6 times. 1) First, 1 mol/L NaOH solution was added to the anode chamber to complete the LSV test. 2) Then, 850 mg sodium lignosulfonate was added to complete the LSV test. 3) After the CA test at 1.6 V cell voltage for 6 h, the rest remained unchanged to complete the LSV test. 4) The LSV test was completed by only replacing the deactivated Ti/PbO2 with fresh Ti/PbO2 which is of the same size. 5) Using deactivated Ti/PbO2, the solution in the anode chamber was replaced with fresh 1 mol/L NaOH solution to complete the LSV test. 6) At last, the LSV test was completed by adding 850 mg sodium lignosulfonate into fresh 1 mol/L NaOH solution using deactivated Ti/PbO2.
Results and Discussion
Electrochemical Properties of Ti/PbO2
The oxidation reaction of lignin at the anode occurs at the anode-electrolyte heterogeneous interface, and the oxidation efficiency of lignin is closely related to the electrode materials. This section focuses on the electrochemical properties of Ti/PbO2 as the anode to oxidize water or lignin. The oxidation reaction of lignin and OER are competitive, and the oxygen evolution potential (OEP) of the anode is also important to further understand the mechanism of the anodic reaction. The CV test of Ti/PbO2 in 1 mol/L NaOH solution was performed to analyze its OEP, and the results are shown in Figure 2A. At 30°C without lignin, the OEP of Ti/PbO2 was 0.73 V vs. Hg/HgO (1.65 V vs. RHE), which showed a high oxygen evolution overpotential. After adding 10 g/L lignin, no new oxidation peak appeared, the OEP increased and OER reaction kinetics decreased. But at lower anode potential (0.5–0.7 V vs. Hg/HgO), the CV curves had a higher current response, which indicated that the oxidation reaction of lignin was thermodynamically more favorable than OER. The addition of lignin inhibits the occurrence of OER because the oxidation reaction of lignin occupies the active site of oxygen evolution reaction, and the reaction kinetics of lignin oxidation is worse than that of OER, resulting in a reduced current response at higher potential (Shao et al., 2014). In consideration of this effect, the experiments involved in the following experiments should try to ensure a low anode potential to avoid the occurrence of competitive OER. Figure 2C shows the CV curves of lignin at different temperatures of 30°C, 40°C, and 60°C. With the increase of temperature, the oxidation peak around 0.4 V vs. Hg/HgO gradually moved to the opposite direction to the negative potential, and the peak current gradually increased, and the anode-lignin reaction activity gradually increased.
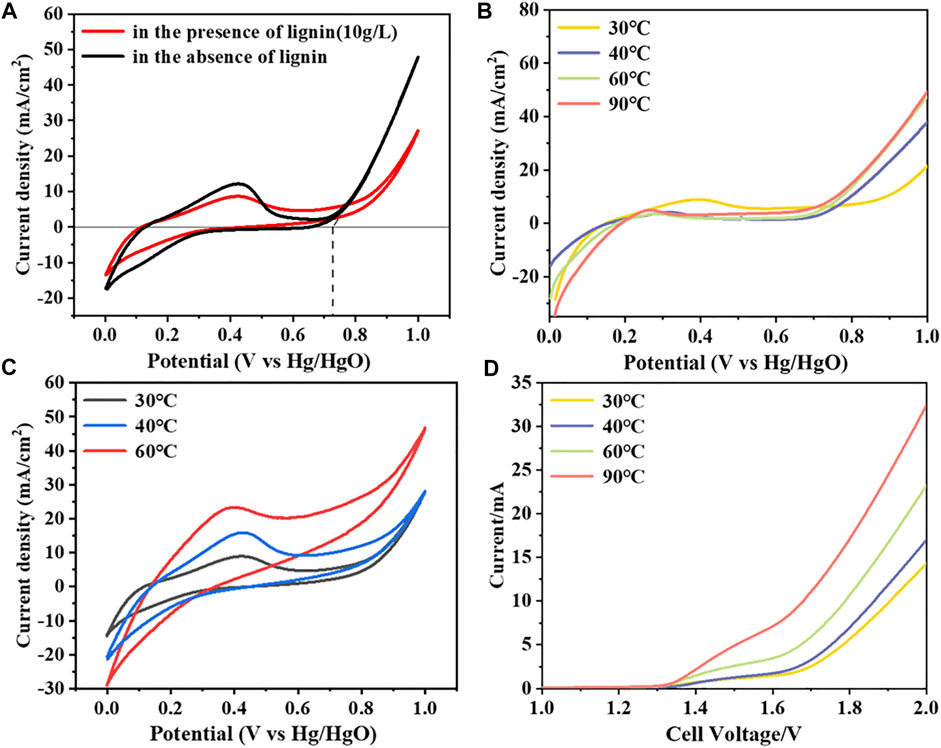
FIGURE 2. The electrochemical performance of Ti/PbO2 as the anode in AWE and LAWE: (A) CV curves of Ti/PbO2 at 30°C, with a scan rate of 20 mV/s; (B) LSV curves at different temperatures in AWE, with a scan rate of 5 mV/s; (C) CV curves of lignin added at different temperatures, with a scan rate of 20 mV/s; (D) Two-electrode LSV curves at different temperatures, with a scan rate of 5 mV/s. Solution: 1 mol/L NaOH.
This study mainly focuses on the influence of temperature on hydrogen production rate, lignin oxidation efficiency, etc. The temperature also affects the occurrence of anodic OER. Figures 2B,D show the LSV curves of water electrolysis at different temperatures and the polarization curves of the two-electrode system respectively. With the increase of temperature, the OEP of Ti/PbO2 decreased, and the current density of the same potential increased, indicating that the increase of temperature could reduce the cell voltage and improve the rate of hydrogen production. The cell voltage could decrease from 2.04 to 1.77 V at 5 mA/cm2 as the temperature increased from 30°C to 90°C.
Effect of the Temperature and Lignin Concentration on the LAWE
In this study, some tests were carried out in the two-electrode system. In the LAWE, the influence of temperature and lignin concentration on hydrogen production rate was emphatically investigated. Figure 3 shows the LSV curves at different temperatures of 30, 40, 60, and 90°C, in the meantime, to compare the LAWE and AWE. All the experiments were operated with the addition of 10 g/L lignin. Under all temperature conditions, the current density of LAWE was obviously higher than that of AWE at the same cell voltage. For example, when the current was 10 mA at 60°C, the cell voltage of water electrolysis for hydrogen production was 1.79 V, while it was only 1.23 V for LAWE. And when the current is 5 mA, compared the LAWE and AWE, the difference of cell voltage was 509 mV, 489 mV, 546 mV, 339 mV under the temperature of 30, 40, 60, and 90°C, respectively. The maximum difference occurred at 60°C, so the later CA tests were almost under the condition of 60°C. Therefore, it can be concluded that replacing the OER reaction with anodic lignin oxidation reaction can reduce the energy consumption of hydrogen production.
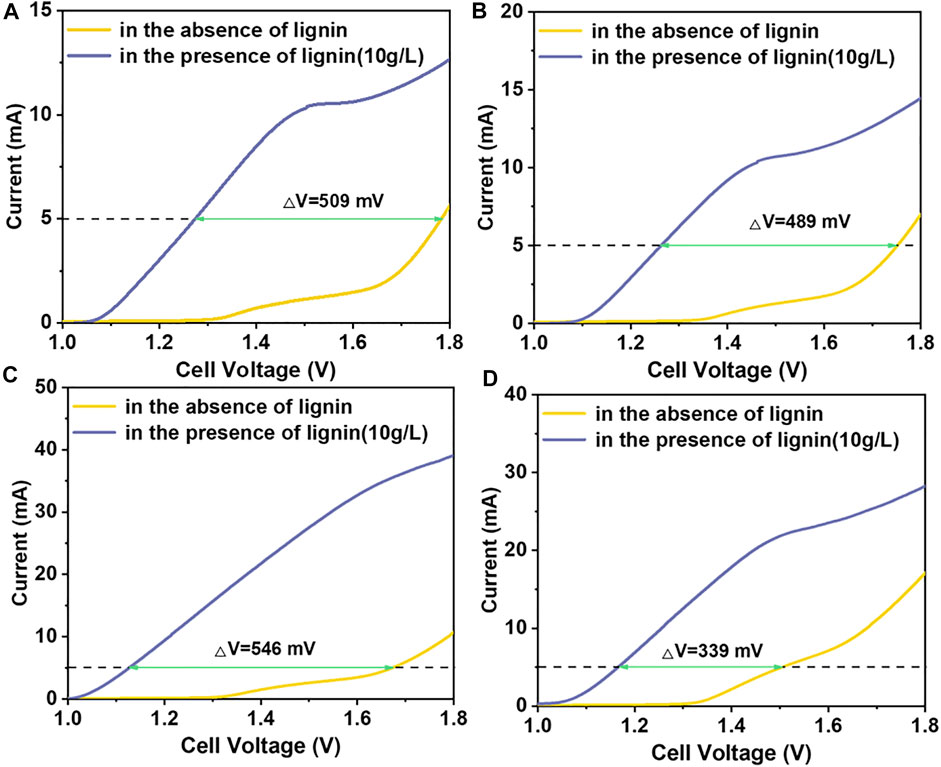
FIGURE 3. Comparison of LAWE and AWE at different temperatures: LSV curves of (A) 30°C, (B) 40°C, (C) 60°C and (D) 90°C, with a scan rate of 5 mV/s. Solution: 1 mol/L NaOH.
Figure 4A shows the I-t curves of LAWE under different conditions at 60°C. Except for the control test, 10 g/L lignin was added to the anode chamber. Compared with AWE, LAWE for hydrogen production had a higher current response, but with the extension of electrolysis time, there was a trend of a slow decline of current, and the anodic oxidation reaction of lignin is a diffusion control process. Possible reasons include the anode deactivation and the consumption of lignin. Detailed analysis of related reasons is given in hereafter this text. Figure 4B shows the total amount of charge transferred by CA tests for 5 h under different working conditions, where 60°C-1.6-repeat refers to the repeated experiment. As the temperature or the cell voltage increased, the total amount of transfer charge increased. The cell voltage or anode potential had a greater effect on the total transfer charge. When the cell voltage increased from 1.6 to 1.8 V, the total transfer charge could be more than doubled. With the increase of cell voltage, more adsorbed hydroxyl radicals could be generated on the surface of the anode, which improves the oxidation efficiency of lignin macromolecules and the rate of hydrogen production.
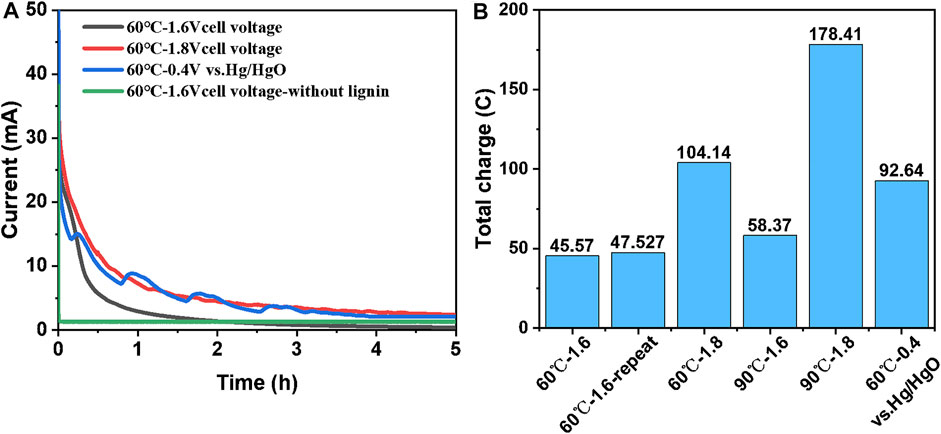
FIGURE 4. CA tests for LAWE at 60°C: (A) I-t curves of LAWE; (B) total transfer charge for CA tests 5 h. Solution: 1 mol/L NaOH and 10 g/L lignin.
To investigate the effect of lignin concentration on hydrogen production rate, LSV and CA (60°C-1.6 V) tests with different lignin concentrations were carried out. The experimental results are shown in Figure 5. With the increase of lignin concentration, the current response gradually increased, and the total charge transferred gradually increased. When the concentration of lignin is 1 g/L, the LSV curve is almost the same as that of water electrolysis, and the concentration of lignin is too low for the anode to effectively oxidize lignin. When the lignin concentration increased from 10 to 20 g/L, the LSV curve had little difference relative to the condition of lignin concentration increased from 1 to 10 g/L. In the absence of stirring, the anodic reaction is controlled by diffusion, which increases the overall solution concentration and fails to replenish the anodically oxidized lignin effectively. In addition, the total transferred charges of the two were 47.53 and 185.45°C, respectively. When the concentration of lignin doubled, the total transferred charges increased about four times, which did not show a proportional relationship, which may be related to the low oxidation degree of lignin at a low concentration.
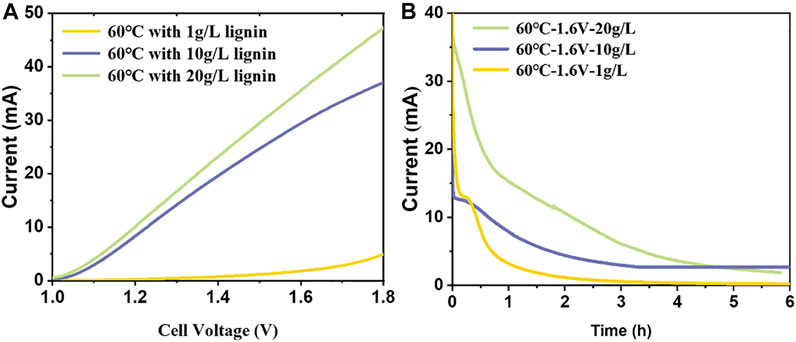
FIGURE 5. The influence of lignin concentration on LAWE: (A) LSV curves, with a scan rate of 5 mV/s; (B) I-t curves. 60°C with different lignin concentrations. Solution: 1 mol/L NaOH.
Anode Deactivation
Anode deactivation occurs in most heterophase lignin oxidation systems. Some studies suggested that small generated molecules, after lignin was oxidized and depolymerized, would adhere to the electrode surface (Du et al., 2020), while others suggested that the recondensation of small molecules would hinder the further occurrence of the reaction (Ezerskis and Jusys, 2001; Shiraishi et al., 2012). Some studies also used the lignin model substrate to investigate the oxidation mechanism of lignin on the anode, and gradual deactivation of the anode was also found (Beliaeva et al., 2020). Figure 6A shows the CV curves of the anode after the addition of lignin at 40°C, with a scan rate of 20 mV/s, which was accompanied by stirring. With the increase of CV cycle number, the current density decreased significantly and the anode deactivation occurred. Among them, CV had a high scan rate, so the current drop caused by lignin consumption could be ignored. Moreover, anode deactivation was also found in the above experiments. In the CA tests at a low temperature (30, 40°C), the current eventually dropped to 0, and the anode material was attached to red substances, as shown in Figure 6B. But under the temperature of 60°C and 90°C, the formation of red substance could not be observed. However, if the electrode is not cleaned in time and the temperature is naturally cooled, the surface of the electrode will also be attached with the red substance. Among them, part of the red material dissolved in water, could be cleaned by deionized water, but part of the red material would be firmly attached to the surface of the anode, even using ultrasonic cleaning could not be removed. Compared with the normal anode, the SEM image of deactived Ti/PbO2 showed that the film was mostly covered and originai PbO2 film could not be observed (Figures 6C,D).
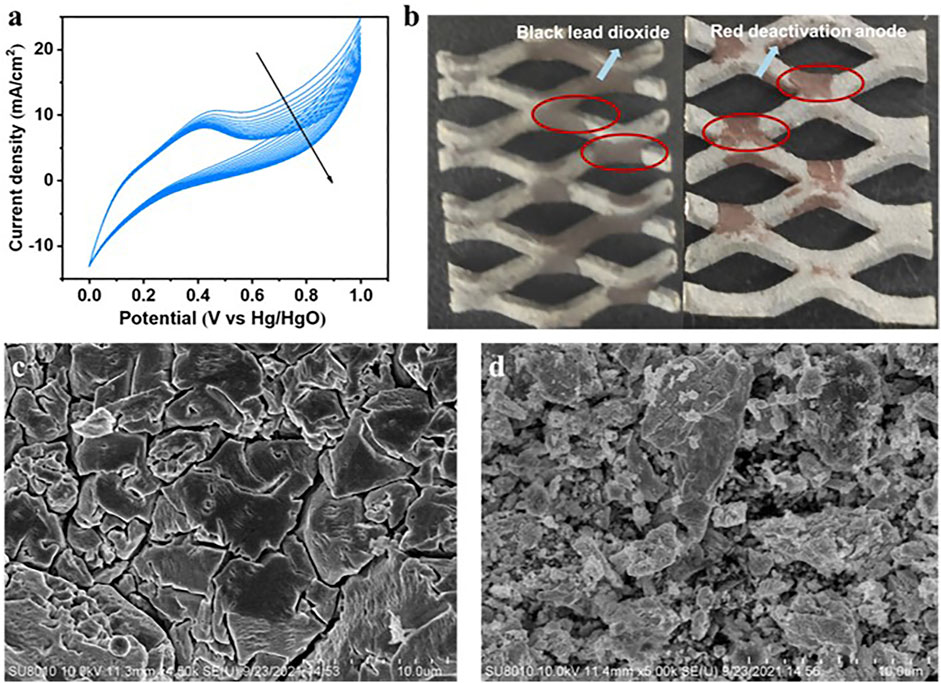
FIGURE 6. Anode deactivation: (A) CV curves of the anode with the addition of lignin at 40°C, with a scan rate of 5 mV/s, 15 cycles; (B) deactivation anode with the red substance on the electrode surface; (C) SEM image of normal Ti/PbO2 anode; (D) SEM image of deactivated Ti/PbO2 anode.
In this paper, the mechanism of anode deactivation was investigated by electrochemical tests, as shown in Figure 7. LSV tests were carried out at 60°C with a scan rate of 5 mV/s. The six curves represent: 1) Ti/PbO2 - NaOH solution; 2) Ti/PbO2-lignin-NaOH solution; 3) Deactivated Ti/PbO2- lignin-NaOH solution after CA test; 4) new Ti/PbO2- lignin-NaOH solution after CA test; 5) new Ti/PbO2-new NaOH solution; 6) Deactivated Ti/PbO2 -new lignin-NaOH solution. Compared tests 3) with 1) and 2), the current density of curve 3) which involved the deactivation anode and lignin-NaOH solution after CA test was even lower than that of water electrolysis. By only changing the electrode, comparing curve 4) with curve 1) and curve 2), it can be concluded that there is still a certain current density under the condition of low potential, and the lignin in the solution still has the potential to be oxidized, so that the lignin cannot be completely oxidized by one time CA test. According to the comparison of curves 1) and 6), 2) and 5), the activity of the deactivated electrode could be regenerated after replacing it in fresh NaOH or lignin-NaOH solution. No red substance was observed on the surface of the electrode during CA tests at 60°C, and before test 5) and 6) the deactivated electrode was not cleaned.
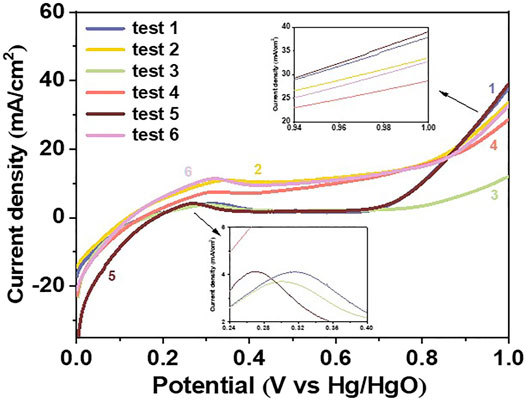
FIGURE 7. LSV curves of Ti/PbO2 anode under different conditions to analyze the anode deactivation mechanism. Test (1) Ti/PbO2 - NaOH solution; (2) Ti/PbO2-lignin-NaOH solution; (3) Deactivated Ti/PbO2- lignin-NaOH solution after CA test; (4) new Ti/PbO2- lignin-NaOH solution after CA test; (5) new Ti/PbO2-new NaOH solution; (6) Deactivated Ti/PbO2 -new lignin-NaOH solution. Experimental conditions: temperature 60°C, 1 mol/L NaOH, counter electrode Pt. Initial concentration of lignin is 10 g/L.
To sum up, 1) Lignin cannot be completely oxidized by a one-time CA test at 60°C due to anode deactivation; 2) The small molecules generated by lignin oxygenation depolymerization will deactivate the anode, whether or not they are polycondensation. 3) The material that can deactivate the anode is attached to the surface of the anode; 4) The material attached to the anode surface is water-soluble to a certain extent, so the activity of the anode can be regenerated by replacing the anolyte. The composition and formation path of anode products need to be further studied.
Conclusion
This work investigated the effects of temperature and lignin concentration on hydrogen production rate in LAWE, and the Ti/PbO2 anode deactivation mechanism was analyzed. The main conclusions are as follows:
1) Ti/PbO2 anode has high OEP, and with the rise of temperature, the OEP shows a trend of decline. The anodic oxidation of lignin occupies the active site of the OER reaction, so the addition of lignin can inhibit the OER reaction. However, by replacing the OER reaction with the oxidation reaction of lignin, the H-type electrolytic cell can produce hydrogen at a lower cell voltage. The hydrogen production rate can be effectively increased by increasing the temperature and lignin concentration.
2) The internal resistance (i.e., anion exchange membrane resistance, electrolyte internal resistance) of the H-type electrolytic cell used in this study is large, and the lignin consumption cannot be replenished in time. The hydrogen production rate under the two-electrode system is low. However, the anode potential of 0.303 V vs. Hg/HgO can achieve a current density of 10 mA/cm2, under the condition of 10 g/L lignin and 60°C, which is far lower than the potential needed for OER reaction (0.773 vs. Hg/HgO). The development of more suitable electrolytic devices, such as the application of flow-through electrolytic cells, reducing the distance between anode and cathode, can potentially improve the rate of hydrogen production.
3) Temperature can affect the deactivation of the electrode, and the substance attached to the anode surface at 60°C was colorless and soluble in alkaline solution. The deactivation mechanism of the Ti/PbO2 anode needs to be quantitatively characterized and further analyzed.
Data Availability Statement
All datasets generated for this study are included in the article.
Author Contributions
JL: Writing-original draft; WZ: Writing-review and editing; YH: review and editing; JG: Supervision, review and editing.
Funding
This work was supported by the National Natural Science Foundation of China (Grant No. 52006049).
Conflict of Interest
The authors declare that the research was conducted in the absence of any commercial or financial relationships that could be construed as a potential conflict of interest.
Publisher’s Note
All claims expressed in this article are solely those of the authors and do not necessarily represent those of their affiliated organizations, or those of the publisher, the editors and the reviewers. Any product that may be evaluated in this article, or claim that may be made by its manufacturer, is not guaranteed or endorsed by the publisher.
References
Badwal, S. P. S., Giddey, S. S., Munnings, C., Bhatt, A. I., and Hollenkamp, A. F. (2014). Emerging Electrochemical Energy Conversion and Storage Technologies. Front. Chem. 2 (79), 1–28. doi:10.3389/fchem.2014.00079
Bateni, F., Ghahremani, R., and Staser, J. A. (2021). Electrochemical Oxidative Valorization of Lignin by the Nanostructured PbO2/MWNTs Electrocatalyst in a Low-Energy Depolymerization Process. J. Appl. Electrochem. 51 (1), 65–78. doi:10.1007/s10800-020-01451-y
Bateni, F., NaderiNasrabadi, M., Ghahremani, R., and Staser, J. A. (2019). Low-Cost Nanostructured Electrocatalysts for Hydrogen Evolution in an Anion Exchange Membrane Lignin Electrolysis Cell. J. Electrochem. Soc. 166 (14), F1037–F1046. doi:10.1149/2.0221914jes
Beliaeva, K., Elsheref, M., Walden, D., Dappozze, F., Nieto-Marquez, A., Gil, S., et al. (2020). Towards Understanding Lignin Electrolysis: Electro-Oxidation of a β-O-4 Linkage Model on PtRu Electrodes. J. Electrochem. Soc. 167 (13), 134511. doi:10.1149/1945-7111/abb8b5
Caravaca, A., Garcia-Lorefice, W. E., Gil, S., de Lucas-Consuegra, A., and Vernoux, P. (2019). Towards a Sustainable Technology for H2 Production: Direct Lignin Electrolysis in a Continuous-Flow Polymer Electrolyte Membrane Reactor. Electrochemistry Commun. 100, 43–47. doi:10.1016/j.elecom.2019.01.016
Chai, S., Zhao, G., Wang, Y., Zhang, Y.-n., Wang, Y., Jin, Y., et al. (2014). Fabrication and Enhanced Electrocatalytic Activity of 3D Highly Ordered Macroporous PbO2 Electrode for Recalcitrant Pollutant Incineration. Appl. Catal. B: Environ. 147, 275–286. doi:10.1016/j.apcatb.2013.08.046
Chen, Y. X., Lavacchi, A., Miller, H. A., Bevilacqua, M., Filippi, J., Innocenti, M., et al. (2014). Nanotechnology Makes Biomass Electrolysis More Energy Efficient Than Water Electrolysis. Nat. Commun. 5, 4036. doi:10.1038/ncomms5036
Du, X., Liu, W., Zhang, Z., Mulyadi, A., Brittain, A., Gong, J., et al. (2017). Low-Energy Catalytic Electrolysis for Simultaneous Hydrogen Evolution and Lignin Depolymerization. Chemsuschem 10 (5), 847–854. doi:10.1002/cssc.201601685
Du, X., Zhang, H., Sullivan, K. P., Gogoi, P., and Deng, Y. (2020). Electrochemical Lignin Conversion. ChemSusChem 13 (17), 4318–4343. doi:10.1002/cssc.202001187
Ežerskis, Z., and Jusys, Z. (2001). Electropolymerization of Chlorinated Phenols on a Pt Electrode in Alkaline Solution Part - I: A Cyclic Voltammetry Study. J. Appl. Electrochemistry 31 (10), 1117–1124. doi:10.1023/a:1012280216273
Ghahremani, R., Farales, F., Bateni, F., and Staser, J. A. (2020). Simultaneous Hydrogen Evolution and Lignin Depolymerization Using NiSn Electrocatalysts in a Biomass-Depolarized Electrolyzer. J. Electrochem. Soc. 167 (4), 043502. doi:10.1149/1945-7111/ab7179
Guo, W. L., Li, L., Li, L. L., Tian, S., Liu, S. L., and Wu, Y. P. (2011). Hydrogen Production via Electrolysis of Aqueous Formic Acid Solutions. Int. J. Hydrogen Energ. 36 (16), 9415–9419. doi:10.1016/j.ijhydene.2011.04.127
Hibino, T., Kobayashi, K., Nagao, M., and Teranishi, S. (2017). Hydrogen Production by Direct Lignin Electrolysis at Intermediate Temperatures. Chemelectrochem 4 (12), 3032–3036. doi:10.1002/celc.201700917
Holade, Y., Tuleushova, N., Tingry, S., Servat, K., Napporn, T. W., Guesmi, H., et al. (2020). Recent Advances in the Electrooxidation of Biomass-Based Organic Molecules for Energy, Chemicals and Hydrogen Production. Catal. Sci. Technol. 10 (10), 3071–3112. doi:10.1039/c9cy02446h
Hosseini, S. E., Abdul Wahid, M., Jamil, M. M., Azli, A. A. M., and Misbah, M. F. (2015). A Review on Biomass-Based Hydrogen Production for Renewable Energy Supply. Int. J. Energ. Res. 39 (12), 1597–1615. doi:10.1002/er.3381
Hu, E., Feng, Y., Nai, J., Zhao, D., Hu, Y., and Lou, X. W. (2018). Construction of Hierarchical Ni-Co-P Hollow Nanobricks with Oriented Nanosheets for Efficient Overall Water Splitting. Energy Environ. Sci. 11 (4), 872–880. doi:10.1039/c8ee00076j
Ju, H., Badwal, S., and Giddey, S. (2018). A Comprehensive Review of Carbon and Hydrocarbon Assisted Water Electrolysis for Hydrogen Production. Appl. Energ. 231, 502–533. doi:10.1016/j.apenergy.2018.09.125
Li, C., Zhao, X., Wang, A., Huber, G. W., and Zhang, T. (2015). Catalytic Transformation of Lignin for the Production of Chemicals and Fuels. Chem. Rev. 115 (21), 11559–11624. doi:10.1021/acs.chemrev.5b00155
Li, H.-C., Zhang, Y.-J., Hu, X., Liu, W.-J., Chen, J.-J., and Yu, H.-Q. (2018). Metal-Organic Framework Templated Pd@PdO-Co3 O4 Nanocubes as an Efficient Bifunctional Oxygen Electrocatalyst. Adv. Energ. Mater. 8 (11), 1702734. doi:10.1002/aenm.201702734
Li, X., Cheng, Z., and Wang, X. (2021). Understanding the Mechanism of the Oxygen Evolution Reaction with Consideration of Spin. Electrochem. Energ. Rev. 4 (1), 136–145. doi:10.1007/s41918-020-00084-1
Li, Y., Nagao, M., Kobayashi, K., Jin, Y., and Hibino, T. (2020). A Cellulose Electrolysis Cell with Metal-free Carbon Electrodes. Catalysts 10 (1), 106. doi:10.3390/catal10010106
Liu, W.-J., Xu, Z., Zhao, D., Pan, X.-Q., Li, H.-C., Hu, X., et al. (2020a). Efficient Electrochemical Production of Glucaric Acid and H2 via Glucose Electrolysis. Nat. Commun. 11 (1). doi:10.1038/s41467-019-14157-3
Liu, X., Cai, P., Wang, G., and Wen, Z. (2020b). Nickel Doped MoS2 Nanoparticles as Precious-Metal Free Bifunctional Electrocatalysts for Glucose Assisted Electrolytic H2 Generation. Int. J. Hydrogen Energ. 45 (58), 32940–32948. doi:10.1016/j.ijhydene.2020.09.007
Lu, J., Yin, S., and Shen, P. K. (2019). Carbon-Encapsulated Electrocatalysts for the Hydrogen Evolution Reaction. Electrochem. Energ. Rev. 2 (1), 105–127. doi:10.1007/s41918-018-0025-9
NaderiNasrabadi, M., Bateni, F., Chen, Z., Harrington, P. B., and Staser, J. A. (2019). Biomass-Depolarized Electrolysis. J. Electrochem. Soc. 166 (10), E317–E322. doi:10.1149/2.1471910jes
Nairan, A., Liang, C., Chiang, S.-W., Wu, Y., Zou, P., Khan, U., et al. (2021). Proton Selective Adsorption on Pt-Ni Nano-Thorn Array Electrodes for superior Hydrogen Evolution Activity. Energ. Environ. Sci. 14 (3), 1594–1601. doi:10.1039/d1ee00106j
Reier, T., Nong, H. N., Teschner, D., Schlögl, R., and Strasser, P. (2017). Electrocatalytic Oxygen Evolution Reaction in Acidic Environments - Reaction Mechanisms and Catalysts. Adv. Energ. Mater. 7 (1), 1601275. doi:10.1002/aenm.201601275
Ren, X., Wu, T., Sun, Y., Li, Y., Xian, G., Liu, X., et al. (2021). Spin-polarized Oxygen Evolution Reaction under Magnetic Field. Nat. Commun. 12 (1), 2608. doi:10.1038/s41467-021-22865-y
Rinaldi, R., Jastrzebski, R., Clough, M. T., Ralph, J., Kennema, M., Bruijnincx, P. C. A., et al. (2016). Paving the Way for Lignin Valorisation: Recent Advances in Bioengineering, Biorefining and Catalysis. Angew. Chem. Int. Ed. 55 (29), 8164–8215. doi:10.1002/anie.201510351
Shan, J., Zheng, Y., Shi, B., Davey, K., and Qiao, S.-Z. (2019). Regulating Electrocatalysts via Surface and Interface Engineering for Acidic Water Electrooxidation. ACS Energ. Lett. 4 (11), 2719–2730. doi:10.1021/acsenergylett.9b01758
Shao, D., Liang, J., Cui, X., Xu, H., and Yan, W. (2014). Electrochemical Oxidation of Lignin by Two Typical Electrodes: Ti/SbSnO2 and Ti/PbO2. Chem. Eng. J. 244, 288–295. doi:10.1016/j.cej.2014.01.074
Shiraishi, T., Takano, T., Kamitakahara, H., and Nakatsubo, F. (2012). Studies on Electrooxidation of Lignin and Lignin Model Compounds. Part 1: Direct Electrooxidation of Non-phenolic Lignin Model Compounds. Holzforschung 66 (3), 303–309. doi:10.1515/hf.2011.069
Song, S., Zhang, J., Gözaydın, G., and Yan, N. (2019). Production of Terephthalic Acid from Corn Stover Lignin. Angew. Chem. Int. Ed. 58 (15), 4934–4937. doi:10.1002/anie.201814284
Sun, F., Qin, J., Wang, Z., Yu, M., Wu, X., Sun, X., et al. (2021). Energy-saving Hydrogen Production by Chlorine-free Hybrid Seawater Splitting Coupling Hydrazine Degradation. Nat. Commun. 12 (1), 4182. doi:10.1038/s41467-021-24529-3
Vandyshev, A. B., and Kulikov, V. A. (2017). Energy and Resource Efficiency in Industrial Systems for Production and Use of High-Purity Hydrogen. Chem. Petrol. Eng. 53 (3-4), 166–170. doi:10.1007/s10556-017-0315-9
Verma, S., Lu, S., and Kenis, P. J. A. (2019). Co-electrolysis of CO2 and Glycerol as a Pathway to Carbon Chemicals with Improved Technoeconomics Due to Low Electricity Consumption. Nat. Energ. 4 (6), 466–474. doi:10.1038/s41560-019-0374-6
Wang, C., and Astruc, D. (2021). Recent Developments of Nanocatalyzed Liquid-phase Hydrogen Generation. Chem. Soc. Rev. 50 (5), 3437–3484. doi:10.1039/d0cs00515k
Wang, J., Yu, Q., Li, H., Li, R., Zeng, S., Yao, Q., et al. (2021a). Natural DNA-Assisted RuP2 on Highly Graphitic N,P-codoped Carbon for pH-wide Hydrogen Evolution. Chem. Commun. 57 (59), 7284–7287. doi:10.1039/d1cc01951a
Wang, M., Wang, Z., Gong, X., and Guo, Z. (2014). The Intensification Technologies to Water Electrolysis for Hydrogen Production - A Review. Renew. Sustain. Energ. Rev. 29, 573–588. doi:10.1016/j.rser.2013.08.090
Wang, Y.-s., Yang, F., Liu, Z.-h., Yuan, L., and Li, G. (2015). Electrocatalytic Degradation of aspen Lignin over Pb/PbO2 Electrode in Alkali Solution. Catal. Commun. 67, 49–53. doi:10.1016/j.catcom.2015.03.033
Wang, Y., Wang, A., Xue, Z., Wang, L., Li, X., and Wang, G. (2021b). Ultrathin Metal-Organic Framework Nanosheet Arrays and Derived Self-Supported Electrodes for Overall Water Splitting. J. Mater. Chem. A. 9 (39), 22597–22602. doi:10.1039/d1ta06360j
Wang, Y., Wang, S., Li, R., Li, H., Guo, Z., Chen, B., et al. (2020). A Simple Strategy for Tridoped Porous Carbon Nanosheet as superior Electrocatalyst for Bifunctional Oxygen Reduction and Hydrogen Evolution Reactions. Carbon 162, 586–594. doi:10.1016/j.carbon.2020.03.011
Yang, L., Liu, W., Zhang, Z., Du, X., Gong, J., Dong, L., et al. (2017). Hydrogen Evolution from Native Biomass with Fe3+/Fe2+ Redox Couple Catalyzed Electrolysis. Electrochimica Acta 246, 1163–1173. doi:10.1016/j.electacta.2017.06.124
Yang, Y., Lun, Z., Xia, G., Zheng, F., He, M., and Chen, Q. (2015). Non-precious alloy Encapsulated in Nitrogen-Doped Graphene Layers Derived from MOFs as an Active and Durable Hydrogen Evolution Reaction Catalyst. Energ. Environ. Sci. 8 (12), 3563–3571. doi:10.1039/c5ee02460a
Yu, M., Budiyanto, E., and Tüysüz, H. (2021). Principles of Water Electrolysis and Recent Progress in Cobalt‐, Nickel‐, and Iron‐Based Oxides for the Oxygen Evolution Reaction. Angew. Chem. Int. Ed. 60, 2–26. doi:10.1002/anie.202103824
Yu, P., Peng, R., Jiang, H., Zhang, R., Ma, J., Zhang, J. Z., et al. (2019). Carbon Fiber Supported Pt-Co Electrocatalyst for Coal Electrolysis for Hydrogen Production. J. Electrochem. Soc. 166 (13), E395–E400. doi:10.1149/2.0521913jes
Yu, Q., Wang, J., Li, H., Li, R., Zeng, S., Li, R., et al. (2022). Natural DNA-Derived Highly-Graphitic N, P, S-Tridoped Carbon Nanosheets for Multiple Electrocatalytic Applications. Chem. Eng. J. 429, 132102. doi:10.1016/j.cej.2021.132102
Yu, Z.-Y., Lang, C.-C., Gao, M.-R., Chen, Y., Fu, Q.-Q., Duan, Y., et al. (2018). Ni-Mo-O Nanorod-Derived Composite Catalysts for Efficient Alkaline Water-To-Hydrogen Conversion via Urea Electrolysis. Energ. Environ. Sci. 11 (7), 1890–1897. doi:10.1039/c8ee00521d
Zhao, H., Lu, D., Wang, J., Tu, W., Wu, D., Koh, S. W., et al. (2021). Raw Biomass Electroreforming Coupled to green Hydrogen Generation. Nat. Commun. 12 (1), 2008. doi:10.1038/s41467-021-22250-9
Zhao, W., Xing, J., Chen, D., Jin, D., and Shen, J. (2016). Electrochemical Degradation of Musk Ketone in Aqueous Solutions Using a Novel Porous Ti/SnO2-Sb2O2/PbO2 Electrodes. J. Electroanalytical Chem. 775, 179–188. doi:10.1016/j.jelechem.2016.05.050
Keywords: hydrogen, water electrolysis, lignin, electrooxidation, lead dioxide
Citation: Li J, Zhou W, Huang Y and Gao J (2021) Lignin-Assisted Water Electrolysis for Energy-Saving Hydrogen Production With Ti/PbO2 as the Anode. Front. Energy Res. 9:762346. doi: 10.3389/fenrg.2021.762346
Received: 21 August 2021; Accepted: 28 October 2021;
Published: 24 November 2021.
Edited by:
Rahul R. Bhosale, Qatar University, QataCopyright © 2021 Li, Zhou, Huang and Gao. This is an open-access article distributed under the terms of the Creative Commons Attribution License (CC BY). The use, distribution or reproduction in other forums is permitted, provided the original author(s) and the copyright owner(s) are credited and that the original publication in this journal is cited, in accordance with accepted academic practice. No use, distribution or reproduction is permitted which does not comply with these terms.
*Correspondence: Wei Zhou, aGl0emhvdXdAaGl0LmVkdS5jbg==; Jihui Gao, Z2FvamhAaGl0LmVkdS5jbg==