- Research and Development Centre, Dubai Electricity and Water Authority (DEWA), Dubai, United Arab Emirates
Ionic liquids present an opportunity to design efficient electrolytes for supercapacitors, which are among the most extensively studied electrochemical energy storage systems. Ionic liquids are promising candidates for supercapacitor electrolytes because they can eliminate issues associated with aqueous and organic solvent-based electrolytes, such as narrow operating potential windows, safety, and performance. The full potential of ionic liquids as electrolytes in supercapacitors need to be further explored due to promising previous efforts invested in ionic liquid-based electrolyte systems for supercapacitor. This review aims to provide an outlook on neat (pure) ionic liquids applied as supercapacitor electrolytes to isolate the prospects and influences of ionic liquids in supercapacitor electrolyte systems. This work primarily focuses on ionic liquid chemistry links to their performance in supercapacitor electrolytes. Deduced features of importance to supercapacitor performance include the presence of functional groups in the ionic liquids, the ionic liquids physicochemical and electrochemical properties. With the different classes of ionic liquids evaluated, ion size-pore size matching of ionic liquid electrolytes and electrode materials, respectively, affect resulting capacitances and energy densities. Several design strategies to enhance supercapacitor performance by improving ionic liquid transport and electrochemical properties are proposed. The proposed strategies and obtained insights consequently informed further discussions on challenges associated with the commercialization of ionic liquids electrolytes.
Introduction
Supercapacitors (SC) are electrochemical energy storage systems that play a significant role in addressing the global challenge of integrating renewable energy sources into the energy mix. Electrolytes are major components of electrochemical energy storage systems like SC. The functions of electrolytes include; transporting reactants or supporting species in bulk and providing electronic insulation between the electrodes/terminals (Dühnen et al., 2020). Currently applied electrolytes in electrochemical energy storage devices include aqueous (Susantyoko et al., 2019; Huang et al., 2019; Sharma et al., 2020; Sundaram and Appadoo, 2020; Minakshi Sundaram et al., 2016), organic solvents (Xia et al., 2017), deep eutectic solvents (Dinh et al., 2020; Jaumaux et al., 2020), and ionic liquids (ILs) (Gunday et al., 2019; Gunday et al., 2020; Martins and Torresi, 2018; Chellappan et al., 2020). In SC, organic solvent-based electrolytes particularly suffer from various drawbacks like; high cost, safety issues, cumbersome synthesis and purification procedures and, low ionic conductivity (Bamgbopa et al., 2017). Hence, there is a need to develop electrolyte systems, which are free from these drawbacks.
SC can be divided into three classes according to the charge storage mechanism: 1) electric double-layer capacitors (EDLC), 2) pseudocapacitor and 3) hybrid supercapacitors (Pal et al., 2019). The EDLC stores charge by the formation of an electric double layer on the electrode-electrolyte interface. The electric double layer formation is due to the adsorption of ions (cations and anions) on the respective electrodes. The fast diffusion of the ions in the electrolyte contributes to the high-power density of EDLC. In pseudocapacitor, the energy is stored by the reversible redox reactions with rapid intercalation of electrolyte ions on the electrodes surface and the electric double layer formation. Hybrid SC is a combination of EDLC and pseudocapacitor, and it displayed superior performance because it combines the charge storage mechanism of both EDLC and pseudocapacitor (Muzaffar et al., 2019). A more detailed description of the supercapacitor types and their charge storage mechanism can be found elsewhere (Raza et al., 2018) (Pal et al., 2019).
Rapid storage and release of energy are peculiar characteristics of SC because of their faster charge-discharge process, which is limited by diffusion. Equivalent specific capacitance (
where
ILs are organic salts made up of cations and anions with a melting point preferably below 100°C (Hallett and Welton, 2011; Abbas et al., 2015; Lethesh et al., 2012). If they remain liquids at ambient conditions, they are termed room temperature ionic liquids (Tokuda et al., 2006; Welton, 2018; Venkatraman et al., 2019). The most used anions and cations for the synthesis of ILs are given in Figure 1. ILs are mainly classified into four groups: aprotic (Esperança et al., 2010), protic (Greaves and Drummond, 2008), zwitterionic (Wu et al., 2018; Grøssereid et al., 2019), and functionalized (task-specific) (Davis, 2004; Butt et al., 2020) ILs (Figure 1).
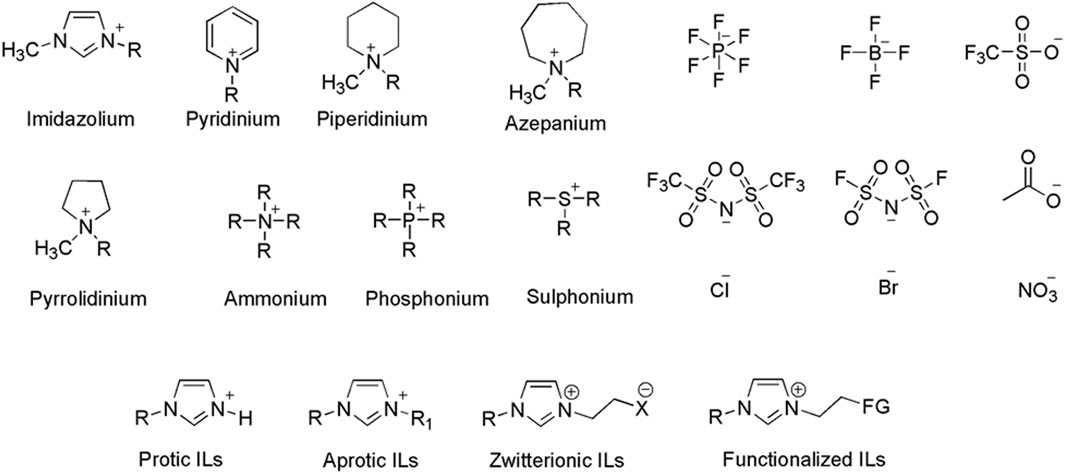
FIGURE 1. Commonly used cations and anions in ILs and the classification of ILs (H- proton, R1-Alkyl chain, X- electronegative atom, FG-Functional group).
In general, the cations are large and bulky, which prevents the efficient packing of ions in the crystal lattice and results in a low melting point. The anions can be organic or inorganic, and their properties largely determine the overall characteristics of the ILs (Marsh et al., 2004; Chellappan et al., 2011; Lethesh et al., 2014b). Physicochemical and electrochemical properties of ILs can also be tuned by a meticulous selection of cation/anion pairs and by introducing desired functional groups (Davis, 2004) (Giernoth, 2010) on the ions according to requirements (Li et al., 2006; Tang et al., 2012). This flexibility makes ILs be regarded as designer solvents.
Given significant effort has been devoted to the development of ILs electrolyte for SCs (Varzi et al., 2014; Aradilla et al., 2019; Ma et al., 2018). Figure 2 summarizes the applications of SCs with IL-based electrolytes, charge storage mechanism of the pseudocapacitor and EDLC (Wang et al., 2016) and graphical representation of cyclic voltammetry (CV) curves of pseudocapacitor and EDLC (Lin Z. et al., 2011). Notwithstanding, some challenges still need to be overcome for increased/large-scale application of ILs as electrolytes for SC. Although many exciting works have reviewed the application of IL electrolyte in SC (Zhong et al., 2015; Van Aken et al., 2015; Shahzad et al., 2019; Lian C. et al., 2019), (Pan et al., 2020; Xu et al., 2021; Miao et al., 2021), a specialized review explaining the relation between IL chemistry and performance of ILs as electrolytes in SC has not received much attention. In addition, there is also a lack of clear explanations regarding the design strategies to improve transport properties and electrochemical performance of the IL electrolytes for SC. Herein, we attempt to bridge this gap with a detailed evaluation of ILs physicochemical properties and their contributions to SC performance, in addition to design considerations and challenges of applying neat ILs as electrolytes in SC. We specifically focus on neat ILs used as SC electrolytes to exclude the influence of other solvents. In neat ILs, the cation nature, the alkyl spacer length on the cation, and the type of anions playing major roles in deciding their electrochemical properties and electrolyte performance. Hence, they provide the opportunity to tune the electrolyte properties by the proper selection of cation-anion combinations. In addition, the effect of solvation can be avoided using ILs because they are made up of cations and anions. Previous studies on ILs electrolytes published from 2010 were only considered to establish a concise review. The abbreviations used in this review are given in Table 1.
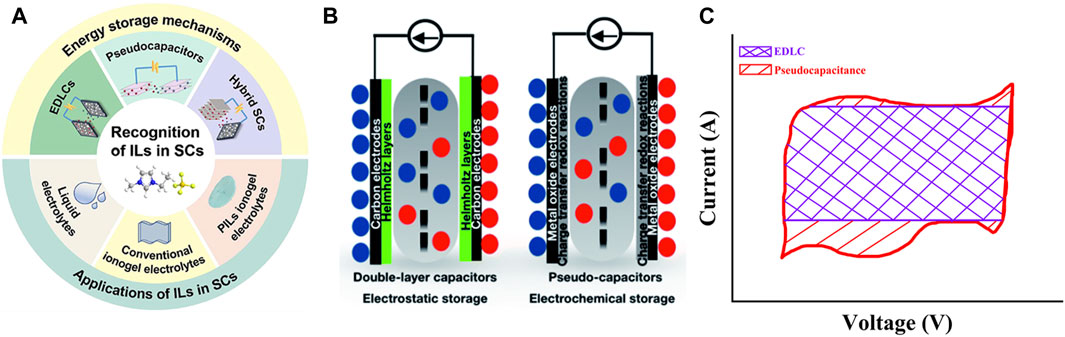
FIGURE 2. (A) ILs application in SCs. Reprinted with permission from (Pan et al., 2020), Frontiers in chemistry, (B) Charge storage mechanism in electric double layer capacitor (EDLC) and pseudocapacitor Reprinted from (Panda et al., 2020), Royal Society of Chemistry. (C) CV of electric double layer capacitor (EDLC) and pseudocapacitor. Reprinted with the permission from (Lin Z. et al., 2011) American Chemical Society.
Supercapacitor Performance with Neat Ionic Liquids Electrolytes
Imidazolium Based Ionic Liquids
Imidazolium-based ILs are among the most extensively studied ILs and electrolytes in SC because of their low viscosity and high conductivity, especially when paired with a range of carbon electrodes, as seen in a recent study (Ortega et al., 2020). Where imidazolium ILs [(EMIM)(Tf2N)] and ([(EMIM)(BF4)] were paired with different carbon electrode materials such as; activated carbon (AC), mesoporous carbon (MES), multi-walled carbon nanotubes (MWCNT), and reduced graphene oxide (RGO) in SC.
The study aimed to obtain insights on; compatibility of the ions with the electrodes, factors affecting the charge accumulation and, its influences on the SC performance. Interestingly, although the ILs investigated contain the same cation, their properties were entirely different. For instance, (EMIM)(Tf2N) is hydrophobic, while (EMIM)(BF4) is hydrophilic. In addition, (EMIM)(BF4) showed higher ionic conductivity (14 mS cm−1) despite its higher viscosity (42 cP). The observations reference the role of the paired anion with imidazolium plays in the physical properties. Figure 3A,B shows; the specific capacitance, energy density and, power density constructed with (EMIM)(Tf2N) and (EMIM)(BF4 ) with the selected electrode material.
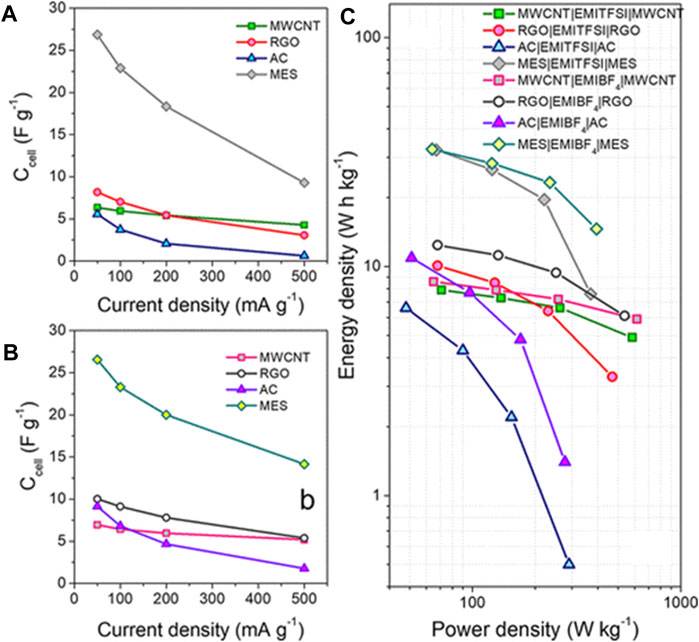
FIGURE 3. Specific capacitance as a function of current density for the SCs with different porous carbon electrodes and with (A) (EMIM)(Tf2N) and (B) (EMIM)(BF4) as electrolytes. Reprinted with permission from (Ortega et al., 2020), American Chemical Society.
We can conclude from Figure 3 that imidazolium-based ILs make better SC with electrode materials containing a high volume of mesopores. The increased power density was achieved with open and accessible electrode material surfaces like mesoporous carbon (Lian Y.-M. et al., 2019) and multi-walled carbon nanotubes (Zhang et al., 2018). These observations are generally valid for a wide range of SC with IL electrolytes. In carbon-based electrode materials with high pore volumes and many micropores (like activated and mesoporous carbons), the interactions between the imidazolium cations and the desorption of co-ions present in the pores govern the specific capacitance and resistance of the electrochemical double-layer capacitance. A summary of the performance of different SC containing neat imidazolium ILs electrolyte is showed in Table 2.
The performance (specific capacity and energy density) of the SC in Table 2 emphasizes that matching the pore size of the electrode with the ionic size of the ILs electrolyte is not the only essential criteria in selecting carbon electrode materials for SC. Other interactions of the ILs with electrode materials, including ion-ion interaction within the ILs, functional groups on the carbon electrode surface, are among other considerations, as will be seen in subsequent sections of this work. A feature observation that qualitatively defines a SC as an electrochemical double layer supercapacitor is the near box-like shape of the cyclic voltammetry response, which also depicts the influence of ion diffusion limitation on limiting current density. Ion diffusion limitations are often defined by electrical conductivity and prevalent electrode mesopore (Hu et al., 2019). The key is that the pore structure of the electrode creates an easy path for the electrolyte ions to access the electrode surface, as shown by Tamailarasan et al. (Tamailarasan and Ramaprabhu, 2012).
Ion diffusion limitations can also be solely a result of the IL property, the physicochemical properties of two imidazolium containing tris(pentafluoroethyl)trifluorophosphate (FAP) and tetracyanoborate {[B(CN)4]−} anions were evaluated, and their performance as the electrolyte in SC was studied (Seki et al., 2012) (Table 2). Due to the larger size of the (FAP)− anion, the viscosity of (FAP)- anion-based IL (43 mPa.s) was higher in comparison with [B(CN)4]− anion-based counterpart (15 mPa.s). At the same time, the ionic conductivity of (EMIM)(FAP) was almost three times lower (6.9 mS cm−1) than (EMIM) [B(CN)4] (19.0 mS cm−1) at 30°C. In other words, the nature of the anions playing a significant role in determining the properties of ILs and the SC performance.
In addition to single/isolated charge-discharge response, an important performance metric for SC is capacity retention and cycling stability (Kurig et al., 2012). In investigations of the chemical reversibility of IL SC systems with (EMIM)+ cation and different anions such as (BF4)−, (Tf2N)−, (FSI)−, (FPA)−, [B(CN)4]− and (SCN)− (Kurig et al., 2011). The oxidation of the (SCN)− to thiocyanogen caused deviation from the ideal capacitive behaviour. Cycling stability in SC can also be linked to the wettability of the electrolyte on the electrode pore walls due to the presence of functional groups on the pore walls (Thangavel et al., 2018).
Generally, we observed from these earlier studies that the relatively good performance of imidazolium cation based ILs as SC electrolytes might be related to small ionic size, appreciable electrochemical stability (depending on the paired anion), low viscosity, and high conductivity.
Redox-Active Ionic Liquids Electrolytes
To further boost the energy density of SC, research efforts have been dedicated to redox-active ILs. An additional charge storage mechanism (pseudocapacitance) is offered from the redox species inherent in the ILs rather than applying pseudocapacitive solid electrode materials. SC assembled with redox-active ILs further blur the lines between batteries and SC, given the galvanostatic charge-discharge response of these SC begins to look more “battery-like”. Fic et al. (2019) took advantage of pseudo halide anion property and excellent transport properties of selenocyanate (SeCN) anion based ILs to develop a redox-active IL for SC. These ILs proved promising candidates as they were previously extensively studied in dye-sensitized solar cells (Wang et al., 2004).
An (EMIM)(SeCN) was tested as a SC electrolyte adopting an activated carbon electrode (Fic et al., 2019). Due to low viscosity (17 mPs) and relatively high conductivity (25 mScm−1), (EMIM)(SeCN) showed promising capacitance (Table 3), especially at higher current density (42 Fg−1 at 2.5 Ag−1). The appreciable performance of the (SeCN)− anion based ILs might be related to their reasonable electrochemical stability, low viscosity, small ionic volumes, and, therefore, high ionic conductivity. Other previous research efforts applied; Cu (II) metal-containing ILs (Sun et al., 2010), bromide ion species (Br2/Br3−) (Yamazaki et al., 2012), and hydroquinone (Sathyamoorthi et al., 2015).
The higher capacitance value of copper containing ILs is associated with the pseudocapacitance contribution from Cu (II)/Cu redox reaction (Table 3). Imidazolium cation can coordinate with the metals through its acidic proton on the C2 carbon atom and form the Cu (II) complex of the ILs (Blue et al., 2006). It is worth noting that thermal stability and the effect of halide content on transport properties of ILs with the metal complexes are often unreported. It is known that even a minimal concentration of halide impurities can detrimentally affect the viscosity of ILs (Seddon et al., 2000). Given these concerns and SC performance targets, the optimal position and polarity of the redox-active component/molecule in the IL also need to be evaluated.
Bi-redox ILs with Anthraquinone, on anion [(AQ-PFS)−] and 2,2,6,6-tetramethylpiperidinyl-1-oxyl (TEMPO) on imidazolium cation [(MIM-TEMPO)+] have also been demonstrated as electrolyte for SC (Mourad et al., 2017). The bi-redox IL introduced had to be dissolved in (BMIM)(Tf2N) (because of high viscosity) to enable testing at room temperature. In contrast, neat bi-redox ILs electrolytes were tested at elevated temperatures (60°C) because of the significant decrease in viscosity with increasing temperature.
Applying only (BMIM)(Tf2N) electrolyte, the double-layer charge storage mechanism is seen without any Faradaic reactions. In the case of bi-redox ILs dissolved in (BMIM)(Tf2N), the redox-active ions, (AQ-PFS)− and (MIM-TEMPO)+ are electro adsorbed on the surface of the carbon electrode and also undergo Faradaic reactions. The introduction of the reducible moiety on the anion and oxidizable species on the cation helped generate bi-anions and bi-cations at the anode and cathode. From the above discussion, we can conclude that the introduction of the redox active materials can improve the performance of the SC. However, it can detrimentally affect the transport properties and electrochemical stability of the ILs. The summary of the performance of the redox-active ILs is summarized in Table 3.
Protic Ionic Liquids-Based Electrolytes
The unique feature of the protic ILs (PILs) is the presence of an acidic and liable proton (hydrogen) on the cationic core, which can involve coordinating with metals (Menne et al., 2014). However, this exchangeable hydrogen on the cationic core results in low thermal stability of PILs. Due to the relatively easy synthesis procedure and non-requirement of purification steps, protic ILs receive much attention in various applications such as; electrolyte for lithium-ion rechargeable batteries (Menne et al., 2013; Vogl et al., 2014), extraction processes (Wang et al., 2020; Rodrigues et al., 2018; Janssen et al., 2016).
In a recent study (Mayrand-Provencher and Rochefort, 2009), PILs were prepared from aromatic heterocyclic compounds (base) containing two nitrogen atoms and trifluoroacetic acid. The effect of multiple proton exchange sites and the impact of the acid strength (pKa) on the physicochemical properties of these PILs and the capacitance of SC containing RuO2 electrodes were evaluated. At the same time, specific conductivities recorded varied from 0.71 to 9.07 mScm−1 at 27°C. In all the PILs studied, conductivity improved with the amount of acid used, and the highest value was obtained in a base and acid ratio of 1:2. This increase in conductivity is related to the decrease in the viscosity obtained at that ratio.
As expected with PILs, the pH (potential of hydrogen) of the resulting SC electrolyte has a significant influence on the specific capacitance value, as demonstrated by Demarconnay and co-workers (Demarconnay et al., 2013). PILs such as pyrrolidinium nitrate [(Pyr)(NO3)] and triethylammonium bis(trifluoromethylsufonyl)imide [(N222H)(Tf2N)] with high conductivity, varying pH, and water content were employed as electrolytes with carbon-based electrodes. Varying pH of the electrolyte from 7 to 11 increased the specific capacitance from 121 to 208 Fg−1 for (Pyr)(NO3). The higher capacitance value at pH11 was attributed to the pseudocapacitive contribution arisen due to the increased mobility of PILs proton by the presence of multiple amine sites on the electrode material. The water content in the electrolyte detrimentally affected the operating voltage and specific capacitance value. Considering the difficulty in drying the PILs, care must be taken when selecting the anions of the PILs. The PILs containing hydrophobic anions tend to reduce the water content in the PILs and can contribute to enhanced performance.
In summary, PILs demonstrated so far in the literature are promising candidates for carbon electrode SCs because of the pseudo-faradaic reaction between the oxygen-containing functional groups on the activated carbon and the proton on the PILs. The low operating temperature some PILs offer (Montes-Morán et al., 2004) might be valuable in various applications like aerospace.
Sulfonium Based Ionic Liquids Electrolyte
Like PILs, sulfonium-based ILs are also relatively easy to synthesize for application as SC electrolytes, as shown by Anouti et al. (2012). They synthesized trimethylsulphonium bis(trifluoromethylsufonyl)imide (Me3S) (Tf2N) by the simple alkylation of dimethylsulfide with corresponding alkyl halides, followed by a metathesis reaction of the resulting halide salt with Li (Tf2N). Although the (Me3S)(Tf2N) was obtained as solid at room temperature (Tm = 45.5°C), the IL reported high conductivity (20.42 mS cm−1) at 80°C, which is significantly higher than the ammonium-based ILs used in SC applications. (Me3S) (Tf2N) also showed very low viscosity (3 mPas) at similar experimental conditions. The smaller ionic size and high fluidity at 80°C promoted the high conductivity in (Me3S) (Tf2N) ILs, typical for the ILs containing sulfonium cation. When neat (Me3S) (Tf2N) was applied as SC electrolyte (Maton et al., 2013), a potential window of 5.3V (vs. Ag/Ag+) was reported at 50°C with a capacitance value of 130 Fg-1 and 140 Fg−1 at 50°C and 80°C, respectively. These values are almost double that of (Pyr 1,4) (Tf2N) at similar conditions with the same activated carbon electrodes.
The observed results with (Me3S) (Tf2N) reinforce the influence of high conductivity, lower viscosity, and small size of the (Me3S)+(0.6 nm) cation compared with the (Pyr1,4)+(1.1 nm) cation. The higher energy and power densities recorded for the sulfonium ILs make them a promising electrolyte class for SCs. However, the thermal stability of sulfonium-based ILs is still a concern at elevated temperatures (Zhang et al., 2009; Coadou et al., 2016).
Sampaio and co-workers also (Sampaio et al., 2019) conducted molecular dynamics studies to evaluate the electrical, dynamical and structural properties of three sulfonium based ILs diethylmethylsulfonium bis(trifluromethylsulfonyl)imide [(S221)(Tf2N)], triethylsulfonium bis(trifluromethylsulfonyl)imide [(S222)(Tf2N)] and diethylpropylsulfonium bis(trifluromethylsulfonyl)imide[(S223)(Tf2N)] at atomic level. It was observed that both viscosity and ionic conductivity of these ILs varied according to cation size. However, the differences in the properties had a negligible effect on the performance of the SC, especially on the specific capacitance value, which is contradictory to the earlier reports (Li S. et al., 2016) and our previously highlighted relationships. We suspect the near uniformity of their SC-specific capacitance is due to microporous activated carbon as the electrode material, which has a smaller pore size than the ionic size of the ions in the ILs. Although the easy synthesis and favourable transport properties of sulphonium-based ILs make them suitable candidates for SC electrolytes, design protocols to improve their thermal stability are necessary for their use in high-temperature supercapacitors.
Azepanium Based Ionic Liquids
Most ILs used as electrolytes for SCs are based on aromatic or non-aromatic five/six -membered rings (imidazolium, pyrrolidinium or piperidinium). ILs with seven-membered ring (Azepanium) are also applicable and were synthesized, with their physicochemical and electrolyte performance in SC was compared with the established (Pyr1,4)(Tf2N) electrolyte in a previous study (Pohlmann et al., 2015). The presented azepanium based ILs (N-butyl-N-methylazepanium bis(trifluoromethanesulfonyl)imide [(Azp1,4)(Tf2N)] and N-methyl-N-hexylazepanium bis(trifluoromethanesulfonyl)imide [(Azp1,6)(Tf2N)] displayed higher viscosity and lower conductivity compared to their pyrrolidinium analogues. This observation is possibly due to the larger ring size of the azepanium cation. However, the azepanium based ILs showed comparable thermal stability (>350°C) as pyrrolidinium ILs. The determination of the maximum operating voltage showed that the positive potential limit was not affected by the ILs structure. On the other hand, the negative potential limit was influenced by the size of the cation. The inferior performance of the azepanium based ILs as an electrolyte compared with the pyrrolidinium analogue was related to their high viscosity and lower conductivity. Nevertheless, considering the lower cost of azepanium ILs, modifications to reduce their viscosity and improve the ionic conductivity can transform them into electrolytes of choice in SCs.
Functionalized Ionic Liquids
The performance of ILs based electrolytes in SC can be improved by the cautious design of ILs, including introducing functional groups to conventional ILs. Modifying the IL cations by grafting the alkyl side chain with heteroatoms (Oxygen, Nitrogen, Sulphur, etc.) can dramatically improve the ionic transport properties. The heteroatom on the alkyl chain can alter the electronic distribution of the cationic core, which will improve ionic transport properties and enhance electrochemical performance. Rennie et al. (2013), tethered oxygen atom on the alkyl side chain (ether functionalization) of the imidazolium cation [1-(2-ethoxyethyl)-2,3-dimethylimidazolium bis(trifluromethylsulfonyl)imide], (MMIM 202) [(Tf2N) and phosphonium cation (2-methoxyethyl)trimethylphosphonium bis(trifluromethylsulfonyl)imide, [(P 222,201) (Tf2N))], which significantly improved the specific capacitance and reduced the resistance of a SC containing mesoporous carbon electrode. The presence of the ether group reduced the viscosity of the IL. A similar trend was observed for ILs containing other cationic cores under investigation for viscosity and thermal stability. Electrochemical impedance spectroscopy results of ILs showed significant deviation from the ideal behaviour due to the inhomogeneity on the electric double layer formed, which is typically prominent in viscous ILs with low conductivity. The improved performance of the ether group-containing ILs might be related to the presence of an electronegative oxygen atom, which might create a remote electronegative region on the cationic core and facilitated the creation of a denser double layer on the electrode surface and resulted in the displacement of a large amount of charge on the electrode.
A comparative study of electrochemical performance of ILs based on two weakly coordinating anions aluminum hexafluroisopropoxilate {[Al(hfip)4]−} and (Tf2N)− was also performed in a SC (Roznyatovskaya et al., 2015). The [Al(hfip)4]– anion showed less electrode interaction and improved transport properties compared to (Tf2N)− anion, when both were evaluated with carbon electrodes possessing micro to macropores—to study the effect of pore size-ion size matching (sieving effect) on capacitance behaviour. Both areal and specific capacitance values with cells combining electrodes with different pore sizes and ILs are shown in Figure 4. The sieving effect is clear in the case of electrodes with micropores. ILs with larger cation [(N1444)+] and anions {[Al(hfip)4] −} displayed lower capacitance value. The larger size of the {[Al(hfip)4]–} anion (1.166 nm) is not able to pass through the microporous carbon electrode with an average pore size of 0.86 nm - resulting in lower capacitance than {Tf2N}− anion. Conversely, there was no significant difference in the capacitance for ILs with mesoporous carbon electrodes with larger pores as expected.
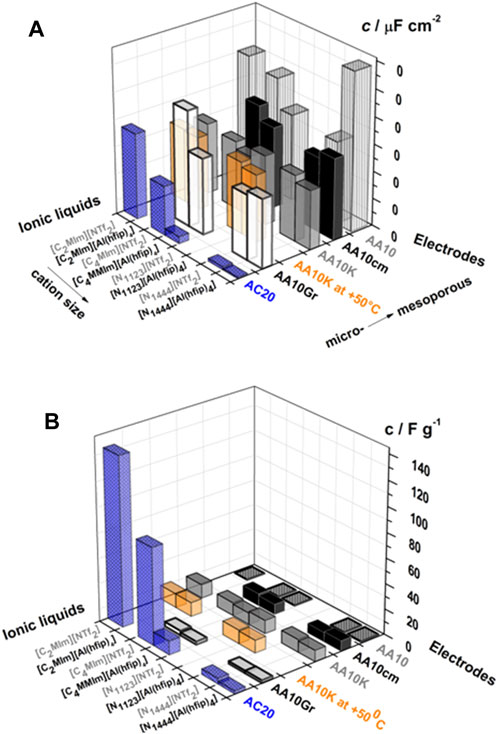
FIGURE 4. (A) Specific capacitances of various carbon electrodes in ILs with [Al(hfip)4]− and (Tf2N)− anions. (B) Gravimetric capacitances of various carbon electrodes in ILs with [Al(hfip)4]− and (Tf2N)− anions. Reprinted with permission from (Roznyatovskaya et al., 2015), Wiley Online Library.
Pyrrolidinium and Piperidinium Ionic Liquids
ILs with pyrrolidinium cations having simple alkyl group [(Pyr 1,3)+, (Pyr 1,4)+] and ether groups [(Pyr 1, 201)+] paired with dicyanamide (DCA) anion have been reported as electrolytes in SCs with an activated carbon-based composite electrode (Wolff et al., 2015). It was previously observed that dicyanamide anion based ILs are typically electrochemically less stable than their (Tf2N)− analogues (Zarrougui et al., 2018). Nevertheless, their high conductivity makes them a good candidate for SC electrolytes. Table 4 shows the SC performance of some reported Pyrrolidinium and Piperidinium ILs.
Both pyrrolidinium and piperidinium cationic cores have also been investigated for SC electrolytes in combination with tricyanomethanide {[C(CN)3]–} and Tetracyanoborate [B(CN)4]– anions (Martins et al., 2017; Martins et al., 2018). It was observed that the ILs with pyrrolidinium and tricyanomethanide combinations showed similar viscosity at 25°C, while (Pip 1,4)[C(CN)3] and (Pip 1,4)[B(CN)4] had significantly higher viscosities due to relatively larger cation size. Consequently, pyrrolidinium-based ILs show better performance as SC electrolytes than the piperidinium counterpart (Table 3), which showed a significant iR drop during discharge. The effect of pyrrolidinium ion size on resulting higher viscosity electrolytes like (Pyr 1,4)(FAP) was also demonstrated (Sillars et al., 2012) on the capacitance of SC applying carbon electrodes. It was observed that the higher viscosity leads to the weakening of the interaction between the electrode and electrolyte ions. From the above discussion, it is clear that the viscosity of ILs playing a significant role in determining the supercapacitor performance, which must be considered while designing new ILs electrolytes for SC.
Other Ionic Liquids Electrolytes Systems
More ILs have been recently developed and studied in electrochemical energy storage applications like SC. An example is ILs derived from dimethylformamide (DMF) type cation, and tetrafluoroborate anion, (EDMF)(BF4) prepared and studied as a potential high-capacitance electrolyte for SC by Chen and co-workers (Chen et al., 2019). The moderate size and quasi-linear nature of the (EDMF)+ cation increased ion mobility and helped the formation of a compact electric double layer resulting in better performance of the SC with (DMF)(BF4) electrolyte.
Most ILs applied as electrolytes in SC contain fluorine in the anions. A recent study (Khan and Shah, 2020)s synthesized an IL with a non-fluorinated Furoate anion, in combination with tetrabutylphosphonium furoate [(P4444)(FuA)], and tested it as an electrolyte for a SCs. The advantage with some of these developed ‘non-conventional’ ILs is that high ionic conductivities and operating potential windows in SC applications can be reported, comparable to the most applied high performing ILs like (EMIM)(BF4). In addition, their synthesis can be simplified. However, there are still setbacks with the newly developed ILs in thermal stability. For example; the Furoate anion based ILs like previously mentioned (P4444)(FuA), are thermally less stable (<250 °C) in comparison to their fluorinated analogues, which is not surprising because carboxyl groups can easily undergo decarboxylation at elevated temperatures (Clough et al., 2013; Cao and Mu, 2014). Nevertheless, this report opened the possibility of developing high performing ILs electrolytes by avoiding fluorinated anions.
The mixing of two neat ILs created a new electrolyte that behaved differently than the constituent ILs as seen in the study highlighted in Figure 5 (Van Aken et al., 2015). The use of such IL mixtures can potentially result in electrolytes with balanced charge storage, high potential window, and high energy density (Van Aken et al., 2015).
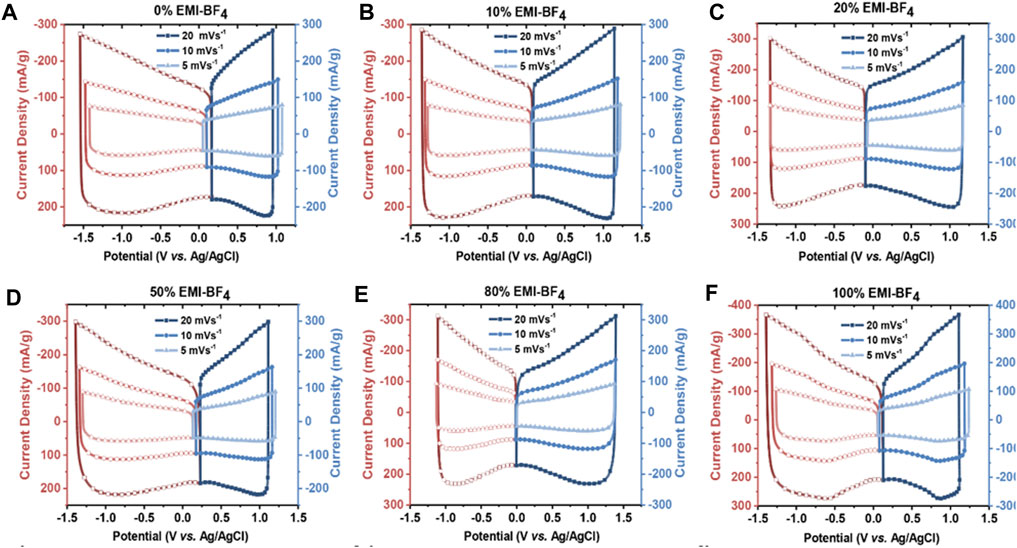
FIGURE 5. Cyclic voltammograms for cells assembled with (EMIM)(Tf2N) and (EMIM)(BF4) mixture with different volumetric percentages of (EMIM)(BF4), including (A) 0%, (B) 10%, (C) 20%, (D) 50%, (E) 80%, and (F) 100%. Operating potential window in all cases is 2.5 V and rates are 5, 10, and 20 mVs−1. Reprinted with the permission from (VanAken et al., 2015), Wiley Online Library.
Another study (Lin R. et al., 2011) explored IL mixtures by mixing two ILs with the same anion bis(fluorosulfonyl)imide [(FSI]−], with similar cations [(Pyr 1,3)+ and (Pip 1,3)+, which prevented ordered arrangement of the ions in the crystal lattice to avoid crystallization. The result was a new IL with a broader liquid range compared to the constituent ILs. Mixing of the two ILs extended the liquid range of the newly formed mixture to as low as −50°C, resulting in the capability to operate a SC with extended operating temperature from −50 to 100°C.
As suggested so far, the electrochemical performance of these non-conventional ILs is defined by their molecular structure. Therefore, the structure–performance influence is always worthy of investigation. Mousavi et al. (2016) reported the effect of ILs structure on the physical properties, electrochemical stability, capacitance, and specific energy when applied as electrolytes in SC using carbon electrodes with uniform and highly interconnected mesopores. ILs containing different anions (tetrafluoroborate, trifluoromethanesulfonate, trifluoromethanesulfonimide and most used cations (imidazolium, ammonium, pyridinium, piperidinium, and pyrrolidinium) were selected for the study seen in Figure 6.
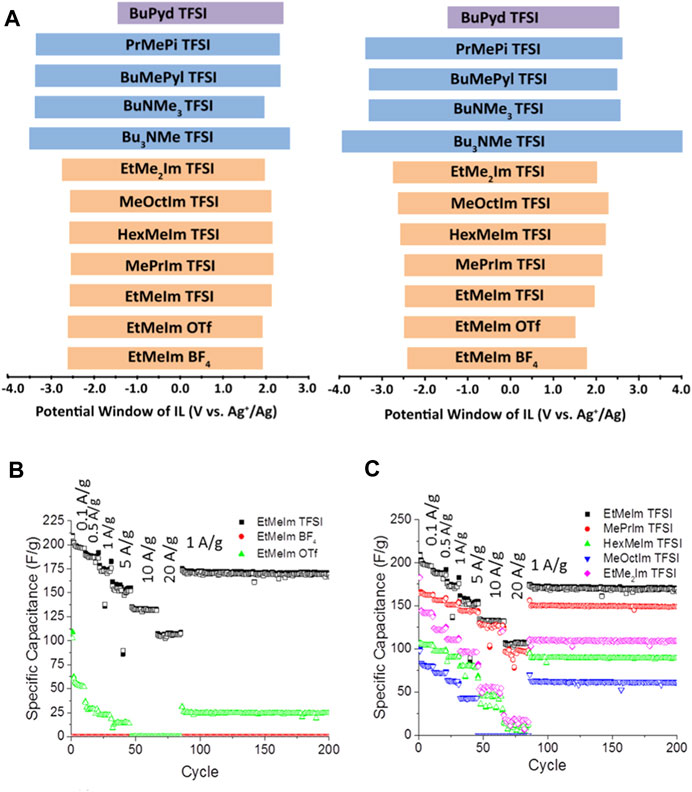
FIGURE 6. effect of; (A) alkyl chain length on the electrochemical stability window, (B) anion, (C) alkyl spacer length on the specific capacitance values at various rates for mesoporous carbon electrodes using the IL electrolytes. Reprinted with permission from (Mousavi et al., 2016), American Chemical society.
The electrochemical stability measurements of the ILs showed that anions have little effect on the cathodic stability of these ILs. On the other hand, the cation has a significant influence on the anodic limit of the ILs. Quaternary ammonium ILs showed the highest anodic stability compared to their aromatic counterparts. Given cation structure substantially impacts the cathodic stability of the ILs, non-aromatic quaternary ammonium cationic cores displayed better stability than their aromatic analogues. The reason is that cations with lower LUMO (lowest unoccupied molecular orbit) energy levels are prone to reduction. The investigators observed that alkyl spacer lengths on the cations had no significant influence on the electrochemical stability of the ILs. Cation size also had a considerable effect on the viscosity and conductivity of the electrolyte and the capacitance of assembled SC. In the study (Mousavi et al., 2016), imidazolium and pyridinium-based ILs provided the highest cell capacitance, and ammonium-based ILs offer potential windows much larger than imidazolium and pyridinium ILs. Although alkyl chain lengths on the imidazolium cation did not influence their electrochemical potential window, it significantly influenced the specific capacitance of SCs with imidazolium ILs (Figure 6).
The increase in the alkyl chain length on the imidazolium cation (larger cation) decreased the gravimetric specific capacitance due to the decrease in the concentration of the counterions in the electrode pores due to the sieving effect of the electrolyte. In addition, the increased ionic size also reduces the accessible surface area. (EMIM)(Tf2N) displayed the highest capacitance among the imidazolium ILs studied (Mousavi et al., 2016). This study clearly shows that the size of the ILs ions should be considered when selecting electrode materials for ILs based supercapacitors.
Design Strategies for Ionic Liquids Electrolyte for Supercapacitors
ILs have some advantages over conventional organic and aqueous electrolytes for SC. However, ILs suffer some setbacks such as high viscosity, low ionic conductivity, and low degree of ion dissociation—resulting in SC with lower power than other electrolytes. From our discussions on different IL structures, resulting IL physicochemical properties, and their IL–electrode interactions in SCs, we propose different design strategies applied to IL development to improve their SC performance.
One of the methods to increase ionic conductivity by decreasing viscosity of the ILs is through the introduction of functional groups such as ether groups on the cationic core of the ILs (Chen et al., 2012; Raj et al., 2018; Raj et al., 2017; Chen et al., 2016; Jin et al., 2012). The introduction of these functional groups on the cation can modify the electronic environment of the ILs due to their highly flexible nature, which increases their free volume and results in a lower viscosity (Chen et al., 2012). Another strategy to reduce the viscosity of the ILs is creating asymmetry in the cation by attaching alkyl substitution in the relevant positions of the cationic core. For instance, the viscosity of the pyridinium-based ILs, as previously discussed, can be significantly improved by attaching alkyl groups on the meta (3) position of the pyridine ring (Lethesh et al., 2019; Chellappan et al., 2011).
Anions play a crucial role in determining the overall electrochemical properties of ILs. Viscosity, conductivity, thermal stability, and hydrophobicity of the ILs can be tuned with the selection of relevant anions with delocalized charge or weak coordination (Timofte and Mudring, 2006). It is worth noting that although non-coordinating anions can reduce the viscosity and increase the conductivity of ILs, these anions can also detrimentally affect the operating potential of ILs (Pandey and Hashmi, 2013). Pairing cations with smaller size anions have conflicting effects. Smaller ions can increase the conductivity of ILs because of their higher intrinsic mobility (Tsuzuki et al., 2005; Yoshida et al., 2007). Conversely, smaller ions can also result in increased ion-ion Coulombic interactions resulting in high viscosity. Hence, an optimum ion size pairing is sought to maximize conductivity and minimize the viscosity of resulting IL.
Improving the electrochemical stability of ILs can be done by introducing modifications to the cationic core of the ILs. It is known that quaternary ammonium ILs undergo decomposition primarily through Hoffman elimination by utilizing the hydrogen atoms on their β-position (DeVos et al., 2014; Lethesh et al., 2014a). The replacement of the β-hydrogens can limit the Hoffman elimination reaction rate and significantly improve the electrochemical potential window of ILs. However, it is worth noting that the replacement of the β-hydrogens might affect other physicochemical properties (depending on the nature of the substituents) of the ILs. Hence, care must be taken while selecting the substituents on the β position of the quaternary ammonium ILs. As discussed earlier, phosphonium cations can increase the electrochemical stability and operating potential window of SC (Khan and Shah, 2020). The use of tetralkylphosphonium cations can also increase the electrochemical stability window of ILs because of their unique electrochemical decomposition mechanism (Bradaric et al., 2003). However, higher viscosity of the phosphonium based ILs must be taken into account while employing them as an electrolyte in SC (Del Sesto et al., 2005).
Organic solvent additives or mixtures with ILs can also optimize the ILs electrolytes for SC applications. Although IL-organic solvent mixture electrolytes improve capacitance compared with the neat ILs system (Lian et al., 2016; Siinor et al., 2013), energy density is sometimes compromised when the mixture results in a narrower potential window. Therefore, adding organic solvents with good conductivity and high anodic stability is expected to improve both capacitance and energy density in IL-organic solvent mixture electrolyte systems. Furthermore, even when a high electrochemical potential window ILs are applied in a SC, the IL may not be fully utilized in both electrodes of the SC when there is an asymmetry in the capacitance of the anode and cathode. This imbalance can result in electrolyte decomposition and can be remedied to achieve high cell voltage by mixing two suitable ILs instead of an IL-organic solvent mistune.
Conclusion and Future Perspective
This work sought to provide an outlook on the prospects of neat ionic liquids (ILs) as supercapacitor (SC) electrolytes. We specifically focus on IL—SC electrolyte design considerations from our observations and reported conclusions in previous works towards the performance of neat ILs in SCs.
The chemistry of charge storage reactions involved in different classes of ILs electrolyte was explained in detail before we provided design strategies for new IL electrolyte systems for SCs. The role of ILs physicochemical properties such as viscosity, ionic conductivity, ionic size, thermal stability, electrochemical stability (potential window) on the performance of the SC—in terms of specific capacitance, energy density was discussed. In addition, we also highlighted plausible mechanisms of IL cycling stability when paired with typical carbon-based electrode materials in SC.
The effect of ILs structure and the impact of distinct functional groups on their physicochemical properties were provided in detail. Although notable accomplishments were made in ILs electrolytes for SCs, many challenges are yet to be addressed in the commercialization of SC with ILs electrolytes. A practical method for widening the operating potential window and operating temperature range of the IL-based electrolytes is yet to be established. We found there is a lack of clear understanding about the electrochemical degradation mechanism of the ILs electrolyte in SC, which is a significant roadblock in designing new ILs with a wide operating potential window.
We suggest more effort is required both experimentally and theoretically to establish the relation between ILs transport and thermal properties with respect to its molecular structure, which will help to design promising ILs electrolytes for electrochemical energy storage devices. More studies are also required towards the standardization of the electrochemical characterization methods for IL electrolytes.
Formulation of electrolytes with a wide electrochemical stability window is essential for developing high-performance SC, and ILs displayed massive potential in this regard. Although ILs can widen the operating potential of the SC devices, the design of ILs suitable for different operating conditions is essential to utilize their full potential. For instance, ILs with promising transport properties at sub-zero temperature and ILs with long-term thermal stability of more than 200°C are not available. Hence, it is necessary to focus on developing ILs to operate in different experimental conditions. Another exciting research direction will be creating a suitable method for identifying the best electrode - ILs combination because the literature data confirmed that the electrode structure could influence the arrangement of ILs at the interface. The technique will help to improve the capacitance of the SC without sacrificing the power and energy density. The commonly used ILs as an electrolyte in SC have moderate to high toxicity, and they are not readily biodegradable. The use of such toxic ILs in a commercial scale can cause environmental and health issues. Hence, it is vital to develop non-toxic and readily biodegradable ILs without compromising their electrochemical and transport properties in large-scale electrochemical applications.
Despite the unique advantages of ILs electrolytes, their cost and purification issues are major concerns while using them in commercial applications. The development of a simple post-synthesis purification step (or no purification step) and the use of low-cost raw materials need to be explored to make ILs a cheaper alternative to organic-based electrolytes. The purification of ILs is critical because even a trace amount of impurities (water/halide) decreases the operating potential window and increases self-discharge. Due to the enormous increase in the demand for wearable devices, the “ionogel” electrolyte (neat ILs with polymer skeleton) might be the future of IL-based electrolytes, providing adequate mechanical stability improvements. Ionogels can provide flexible SCs with superior capability because of their high ion conductivity and fast ion diffusion. There are emerging reports of polymerized ILs based solid electrolytes for SC. We believe these electrolytes will continue to be promising because their appreciable conductivity often comes with notable electrochemical stability.
Author Contributions
The idea of this review paper was conceived by KL. KL wrote the manuscript with significant contributions from MB and RS. All authors have read and approved the final version of the manuscript.
Funding
This research (High Voltage Supercapacitor Cell for Grid Applications) was sponsored by Dubai Electricity and Water Authority (DEWA) R&D center, Dubai, United Arab Emirates.
Conflict of Interest
KL, MB, and RS were employed by the company Dubai Electricity and Water Authority (DEWA).
Publisher’s Note
All claims expressed in this article are solely those of the authors and do not necessarily represent those of their affiliated organizations, or those of the publisher, the editors and the reviewers. Any product that may be evaluated in this article, or claim that may be made by its manufacturer, is not guaranteed or endorsed by the publisher.
References
Abbas, T., Kallidanthiyil Chellappan, L., Mutalib, M. I. A., Cheun, K. Y., Nasir Shah, S., Nazir, S., et al. (2015). Stability and Performance of Physically Immobilized Ionic Liquids for Mercury Adsorption from a Gas Stream. Ind. Eng. Chem. Res. 54, 12114–12123. doi:10.1021/acs.iecr.5b01738
Anouti, M., Timperman, L., El Hilali, M., Boisset, A., and Galiano, H. (2012). Sulfonium Bis(trifluorosulfonimide) Plastic crystal Ionic Liquid as an Electrolyte at Elevated Temperature for High-Energy Supercapacitors. J. Phys. Chem. C 116, 9412–9418. doi:10.1021/jp3012987
Aradilla, D., Sadki, S., and Bidan, G. (2019). Beyond Conventional Supercapacitors: Hierarchically Conducting Polymer-Coated 3D Nanostructures for Integrated On-Chip Micro-supercapacitors Employing Ionic Liquid Electrolytes. Synth. Met. 247, 131–143. doi:10.1016/j.synthmet.2018.11.022
Bamgbopa, M. O., Shao-Horn, Y., and Almheiri, S. (2017). The Potential of Non-aqueous Redox Flow Batteries as Fast-Charging Capable Energy Storage Solutions: Demonstration with an Iron-Chromium Acetylacetonate Chemistry. J. Mater. Chem. A. 5, 13457–13468. doi:10.1039/c7ta02022h
Blue, E. D., Gunnoe, T. B., Petersen, J. L., and Boyle, P. D. (2006). Protonation of N-Heterocyclic Carbene Ligand Coordinated to Copper(I): Coordination Mode of Imidazolium Cation as a Function of Counterion as Determined by Solid-State Structures. J. Organomet. Chem. 691, 5988–5993. doi:10.1016/j.jorganchem.2006.09.051
Bradaric, C. J., Downard, A., Kennedy, C., Robertson, A. J., and Zhou, Y. (2003). Industrial Preparation of Phosphonium Ionic Liquids. ACS Symp. Ser., 41–56. doi:10.1021/bk-2003-0856.ch004
Butt, H. S., Lethesh, K. C., and Fiksdahl, A. (2020). Fuel Oil Desulfurization with Dual Functionalized Imidazolium Based Ionic Liquids. Separation Purif. Technology 248, 116959. doi:10.1016/j.seppur.2020.116959
Cao, Y., and Mu, T. (2014). Comprehensive Investigation on the thermal Stability of 66 Ionic Liquids by Thermogravimetric Analysis. Ind. Eng. Chem. Res. 53, 8651–8664. doi:10.1021/ie5009597
Chellappan, K. C., Van Hecke, K., Van Meervelt, L., Nockemann, P., Kirchner, B., Zahn, S., et al. (2011). Nitrile-Functionalized Pyridinium, Pyrrolidinium, and Piperidinium Ionic Liquids. J. Phys. Chem. B 115, 8424–8438. doi:10.1021/jp2027675
Chellappan, L. K., Kvello, J., Tolchard, J. R., Dahl, P. I., Hanetho, S. M., Berthelot, R., et al. (2020). Non-nucleophilic Electrolyte Based on Ionic Liquid and Magnesium Bis(diisopropyl)amide for Rechargeable Magnesium-Ion Batteries. ACS Appl. Energ. Mater. 3, 9585–9593. doi:10.1021/acsaem.0c01026
Chen, Y., Liu, Z., Sun, L., Lu, Z., and Zhuo, K. (2018). Nitrogen and Sulfur Co-doped Porous Graphene Aerogel as an Efficient Electrode Material for High Performance Supercapacitor in Ionic Liquid Electrolyte. J. Power Sourc. 390, 215–223. doi:10.1016/j.jpowsour.2018.04.057
Chen, Z., Huo, Y., Cao, J., Xu, L., and Zhang, S. (2016). Physicochemical Properties of Ether-Functionalized Ionic Liquids: Understanding Their Irregular Variations with the Ether Chain Length. Ind. Eng. Chem. Res. 55, 11589–11596. doi:10.1021/acs.iecr.6b02875
Chen, Z. J., Xue, T., and Lee, J.-M. (2012). What Causes the Low Viscosity of Ether-Functionalized Ionic Liquids? its Dependence on the Increase of Free Volume. RSC Adv. 2, 10564–10574. doi:10.1039/c2ra21772d
Chen, Z., Li, Z., Ma, X., Wang, Y., Zhou, Q., and Zhang, S. (2019). A New DMF-Derived Ionic Liquid with Ultra-high Conductivity for High-Capacitance Electrolyte in Electric Double-Layer Capacitor. Electrochimica Acta 319, 843–848. doi:10.1016/j.electacta.2019.07.015
Clough, M. T., Geyer, K., Hunt, P. A., Mertes, J., and Welton, T. (2013). Thermal Decomposition of Carboxylate Ionic Liquids: Trends and Mechanisms. Phys. Chem. Chem. Phys. 15, 20480–20495. doi:10.1039/c3cp53648c
Coadou, E., Goodrich, P., Neale, A. R., Timperman, L., Hardacre, C., Jacquemin, J., et al. (2016). Synthesis and Thermophysical Properties of Ether-Functionalized Sulfonium Ionic Liquids as Potential Electrolytes for Electrochemical Applications. Chem Phys Chem 17, 3992–4002. doi:10.1002/cphc.201600882
Del Sesto, R. E., Corley, C., Robertson, A., and Wilkes, J. S. (2005). Tetraalkylphosphonium-based Ionic Liquids. J. Organomet. Chem. 690, 2536–2542. doi:10.1016/j.jorganchem.2004.09.060
Demarconnay, L., Calvo, E. G., Timperman, L., Anouti, M., Lemordant, D., Raymundo-Piñero, E., et al. (2013). Optimizing the Performance of Supercapacitors Based on Carbon Electrodes and Protic Ionic Liquids as Electrolytes. Electrochimica Acta 108, 361–368. doi:10.1016/j.electacta.2013.07.001
De Vos, N., Maton, C., and Stevens, C. V. (2014). Electrochemical Stability of Ionic Liquids: General Influences and Degradation Mechanisms. Chem Electro Chem 1, 1258–1270. doi:10.1002/celc.201402086
Dinh, T. T. A., Huynh, T. T. K., Le, L. T. M., Truong, T. T. T., Nguyen, O. H., Tran, K. T. T., et al. (2020). Deep Eutectic Solvent Based on Lithium Bis[(trifluoromethyl)sulfonyl] Imide (LiTFSI) and 2,2,2-Trifluoroacetamide (TFA) as a Promising Electrolyte for a High Voltage Lithium-Ion Battery with a LiMn2O4 Cathode. ACS Omega 5, 23843–23853. doi:10.1021/acsomega.0c03099
Dühnen, S., Betz, J., Kolek, M., Schmuch, R., Winter, M., Placke, T., et al. (2020). Toward Green Battery Cells: Perspective on Materials and Technologies. Small Methods 4, 2000039. doi:10.1002/smtd.202000039
Fic, K., Gorska, B., Bujewska, P., Béguin, F., and Frackowiak, E. (2019). Selenocyanate-based Ionic Liquid as Redox-Active Electrolyte for Hybrid Electrochemical Capacitors. Electrochimica Acta 314, 1–8. doi:10.1016/j.electacta.2019.04.161
Giernoth, R. (2010). Task-specific Ionic Liquids. Angew. Chem. Int. Edition 49, 2834–2839. doi:10.1002/anie.200905981
Greaves, T. L., and Drummond, C. J. (2008). Protic Ionic Liquids: Properties and Applications. Chem. Rev. 108, 206–237. doi:10.1021/cr068040u
Grøssereid, I., Lethesh, K. C., Venkatraman, V., and Fiksdahl, A. (2019). New Dual Functionalized Zwitterions and Ionic Liquids; Synthesis and Cellulose Dissolution Studies. J. Mol. Liquids 292, 111353. doi:10.1016/j.molliq.2019.111353
Gunday, S. T., Cevik, E., Yusuf, A., and Bozkurt, A. (2019). Nanocomposites Composed of Sulfonated Polysulfone/hexagonal boron Nitride/ionic Liquid for Supercapacitor Applications. J. Energ. Storage 21, 672–679. doi:10.1016/j.est.2019.01.008
Gunday, S. T., Cevik, E., Yusuf, A., and Bozkurt, A. (2020). Synthesis, Characterization and Supercapacitor Application of Ionic Liquid Incorporated Nanocomposites Based on SPSU/Silicon Dioxide. J. Phys. Chem. Sol. 137, 109209. doi:10.1016/J.JPCS.2019.109209
Hallett, J. P., and Welton, T. (2011). Room-temperature Ionic Liquids: Solvents for Synthesis and Catalysis. 2. Chem. Rev. 111, 3508–3576. doi:10.1021/cr1003248
H. Davis, Jr., J. (2004). Task-specific Ionic Liquids. Chem. Lett. 33, 1072–1077. doi:10.1246/cl.2004.1072
Huang, J., Guo, Z., Ma, Y., Bin, D., Wang, Y., and Xia, Y. (2019). Recent Progress of Rechargeable Batteries Using Mild Aqueous Electrolytes. Small Methods 3, 1800272. doi:10.1002/smtd.201800272
Hurilechaoketu, H., Wang, J., Cui, C., and Qian, W. (2019). Highly Electroconductive Mesoporous Activated Carbon Fibers and Their Performance in the Ionic Liquid-Based Electrical Double-Layer Capacitors. Carbon 154, 1–6. doi:10.1016/j.carbon.2019.07.093
Janssen, C. H. C., Macías-Ruvalcaba, N. A., Aguilar-Martínez, M., and Kobrak, M. N. (2016). Copper Extraction Using Protic Ionic Liquids: Evidence of the Hofmeister Effect. Separation Purif. Technology 168, 275–283. doi:10.1016/j.seppur.2016.05.031
Jaumaux, P., Liu, Q., Zhou, D., Xu, X., Wang, T., Wang, Y., et al. (2020). Deep‐Eutectic‐Solvent‐Based Self‐Healing Polymer Electrolyte for Safe and Long‐Life Lithium‐Metal Batteries. Angew. Chem. Int. Ed. 59, 9134–9142. doi:10.1002/anie.202001793
Jin, Y., Fang, S., Chai, M., Yang, L., and Hirano, S.-i. (2012). Ether-functionalized Trialkylimidazolium Ionic Liquids: Synthesis, Characterization, and Properties. Ind. Eng. Chem. Res. 51, 11011–11020. doi:10.1021/ie300849u
Khan, I. A., and Shah, F. U. (2020). Fluorine-Free Ionic Liquid-Based Electrolyte for Supercapacitors Operating at Elevated Temperatures. ACS Sustainable Chem. Eng. 8, 10212–10221. doi:10.1021/acssuschemeng.0c02568
Kurig, H., Vestli, M., Jänes, A., and Lust, E. (2011). Electrical Double Layer Capacitors Based on Two 1-Ethyl-3-Methylimidazolium Ionic Liquids with Different Anions. Electrochem. Solid-state Lett. 14, A120. doi:10.1149/1.3596722
Kurig, H., Vestli, M., Tõnurist, K., Jänes, A., and Lust, E. (2012). Influence of Room Temperature Ionic Liquid Anion Chemical Composition and Electrical Charge Delocalization on the Supercapacitor Properties. J. Electrochem. Soc. 159, A944–A951. doi:10.1149/2.022207jes
Lei, Z., Liu, Z., Wang, H., Sun, X., Lu, L., and Zhao, X. S. (2013). A High-Energy-Density Supercapacitor with Graphene-CMK-5 as the Electrode and Ionic Liquid as the Electrolyte. J. Mater. Chem. A. 1, 2313–2321. doi:10.1039/c2ta01040b
Lethesh, K. C., Dehaen, W., and Binnemans, K. (2014a). Base Stable Quaternary Ammonium Ionic Liquids. RSC Adv. 4, 4472–4477. doi:10.1039/c3ra45126g
Lethesh, K. C., Evjen, S., Raj, J. J., Roux, D. C. D., Venkatraman, V., Jayasayee, K., et al. (2019). Hydroxyl Functionalized Pyridinium Ionic Liquids: Experimental and Theoretical Study on Physicochemical and Electrochemical Properties. Front. Chem. 7. doi:10.3389/fchem.2019.00625
Lethesh, K. C., Parmentier, D., Dehaen, W., and Binnemans, K. (2012). Phenolate Platform for Anion Exchange in Ionic Liquids. RSC Adv. 2, 11936–11943. doi:10.1039/c2ra22304j
Lethesh, K. C., Shah, S. N., and Mutalib, M. I. A. (2014b). Synthesis, Characterization, and Thermophysical Properties of 1,8-diazobicyclo[5.4.0]undec-7-Ene Based Thiocyanate Ionic Liquids. J. Chem. Eng. Data 59, 1788–1795. doi:10.1021/je400991s
Li, J., Tang, J., Yuan, J., Zhang, K., Shao, Q., Sun, Y., et al. (2016a). Interactions between Graphene and Ionic Liquid Electrolyte in Supercapacitors. Electrochimica Acta 197, 84–91. doi:10.1016/j.electacta.2016.03.036
Li, S., Zhu, M., and Feng, G. (2016b). The Effects of Dication Symmetry on Ionic Liquid Electrolytes in Supercapacitors. J. Phys. Condens. Matter 28, 464005. doi:10.1088/0953-8984/28/46/464005
Li, X., Zhao, D., Fei, Z., and Wang, L. (2006). Applications of Functionalized Ionic Liquids. Sci. China Ser. B 49, 385–401. doi:10.1007/s11426-006-2020-y
Lian, C., Liu, H., Li, C., and Wu, J. (2019a). Hunting Ionic Liquids with Large Electrochemical Potential Windows. Aiche J. 65, 804–810. doi:10.1002/aic.16467
Lian, C., Liu, K., Van Aken, K. L., Gogotsi, Y., Wesolowski, D. J., Liu, H. L., et al. (2016). Enhancing the Capacitive Performance of Electric Double-Layer Capacitors with Ionic Liquid Mixtures. ACS Energ. Lett. 1, 21–26. doi:10.1021/acsenergylett.6b00010
Lian, Y.-M., Utetiwabo, W., Zhou, Y., Huang, Z.-H., Zhou, L., Faheem, M., et al. (2019b). From Upcycled Waste Polyethylene Plastic to Graphene/mesoporous Carbon for High-Voltage Supercapacitors. J. Colloid Interf. Sci. 557, 55–64. doi:10.1016/j.jcis.2019.09.003
Lin, R., Taberna, P.-L., Fantini, S., Presser, V., Pérez, C. R., Malbosc, F., et al. (2011a). Capacitive Energy Storage from −50 to 100 °C Using an Ionic Liquid Electrolyte. J. Phys. Chem. Lett. 2, 2396–2401. doi:10.1021/jz201065t
Lin, Z., Liu, Y., Yao, Y., Hildreth, O. J., Li, Z., Moon, K., et al. (2011b). Superior Capacitance of Functionalized Graphene. J. Phys. Chem. C 115, 7120–7125. doi:10.1021/jp2007073
Liu, C., Yu, Z., Neff, D., Zhamu, A., and Jang, B. Z. (2010). Graphene-based Supercapacitor with an Ultrahigh Energy Density. Nano Lett. 10, 4863–4868. doi:10.1021/nl102661q
Ma, K., Zhang, C., Woodward, C. E., and Wang, X. (2018). Bridging the gap between Macroscopic Electrochemical Measurements and Microscopic Molecular Dynamic Simulations: Porous Carbon Supercapacitor with Ionic Liquids. Electrochimica Acta 289, 29–38. doi:10.1016/j.electacta.2018.09.016
Marsh, K. N., Boxall, J. A., and Lichtenthaler, R. (2004). Room Temperature Ionic Liquids and Their Mixtures-A Review. Fluid Phase Equilibria 219, 93–98. doi:10.1016/j.fluid.2004.02.003
Martins, V. L., Rennie, A. J. R., Sanchez-Ramirez, N., Torresi, R. M., and Hall, P. J. (2018). Improved Performance of Ionic Liquid Supercapacitors by Using Tetracyanoborate Anions. ChemElectroChem 5, 598–604. doi:10.1002/celc.201701164
Martins, V. L., Rennie, A. J. R., Torresi, R. M., and Hall, P. J. (2017). Ionic Liquids Containing Tricyanomethanide Anions: Physicochemical Characterisation and Performance as Electrochemical Double-Layer Capacitor Electrolytes. Phys. Chem. Chem. Phys. 19, 16867–16874. doi:10.1039/C7CP03377J
Martins, V. L., and Torresi, R. M. (2018). Ionic Liquids in Electrochemical Energy Storage. Curr. Opin. Electrochemistry 9, 26–32. doi:10.1016/j.coelec.2018.03.005
Maton, C., De Vos, N., and Stevens, C. V. (2013). Ionic Liquid thermal Stabilities: Decomposition Mechanisms and Analysis Tools. Chem. Soc. Rev. 42, 5963–5977. doi:10.1039/c3cs60071h
Mayrand-Provencher, L., and Rochefort, D. (2009). Influence of the Conductivity and Viscosity of Protic Ionic Liquids Electrolytes on the Pseudocapacitance of RuO2 Electrodes. J. Phys. Chem. C 113, 1632–1639. doi:10.1021/jp8084149
Menne, S., Pires, J., Anouti, M., and Balducci, A. (2013). Protic Ionic Liquids as Electrolytes for Lithium-Ion Batteries. Electrochemistry Commun. 31, 39–41. doi:10.1016/j.elecom.2013.02.026
Menne, S., Vogl, T., and Balducci, A. (2014). Lithium Coordination in Protic Ionic Liquids. Phys. Chem. Chem. Phys. 16, 5485–5489. doi:10.1039/c3cp55183k
Miao, L., Song, Z., Zhu, D., Li, L., Gan, L., and Liu, M. (2021). Ionic Liquids for Supercapacitive Energy Storage: A Mini-Review. Energy Fuels 35, 8443–8455. doi:10.1021/acs.energyfuels.1c00321
Minakshi Sundaram, M., Biswal, A., Mitchell, D., Jones, R., and Fernandez, C. (2016). Correlation Among Physical and Electrochemical Behaviour of Nanostructured Electrolytic Manganese Dioxide from Leach Liquor and Synthetic for Aqueous Asymmetric Capacitor. Phys. Chem. Chem. Phys. 18, 4711–4720. doi:10.1039/c5cp07141k
Montes-Morán, M. A., Suárez, D., Menéndez, J. A., and Fuente, E. (2004). On the Nature of Basic Sites on Carbon Surfaces: An Overview. Carbon 42, 1219–1225. doi:10.1016/j.carbon.2004.01.023
Mourad, E., Coustan, L., Lannelongue, P., Zigah, D., Mehdi, A., Vioux, A., et al. (2017). Biredox Ionic Liquids with Solid-like Redox Density in the Liquid State for High-Energy Supercapacitors. Nat. Mater 16, 446–453. doi:10.1038/NMAT4808
Mousavi, M. P. S., Wilson, B. E., Kashefolgheta, S., Anderson, E. L., He, S., Bühlmann, P., et al. (2016). Ionic Liquids as Electrolytes for Electrochemical Double-Layer Capacitors: Structures that Optimize Specific Energy. ACS Appl. Mater. Inter. 8, 3396–3406. doi:10.1021/acsami.5b11353
M. S. S. Esperança, J., Canongia Lopes, J. N., Tariq, M., Santos, L. M. N. B. F., Magee, J. W., and Rebelo, L. P. N. (2010). Volatility of Aprotic Ionic Liquids - A Review. J. Chem. Eng. Data 55, 3–12. doi:10.1021/je900458w
Muzaffar, A., Ahamed, M. B., Deshmukh, K., and Thirumalai, J. (2019). A Review on Recent Advances in Hybrid Supercapacitors: Design, Fabrication and Applications. Renew. Sustainable Energ. Rev. 101, 123–145. doi:10.1016/j.rser.2018.10.026
Ortega, P. F. R., Santos, G. A. D., Trigueiro, J. P. C., Silva, G. G., Quintanal, N., Blanco, C., et al. (2020). Insights on the Behavior of Imidazolium Ionic Liquids as Electrolytes in Carbon-Based Supercapacitors: An Applied Electrochemical Approach. J. Phys. Chem. C 124, 15818–15830. doi:10.1021/acs.jpcc.0c04217
Pal, B., Yang, S., Ramesh, S., Thangadurai, V., and Jose, R. (2019). Electrolyte Selection for Supercapacitive Devices: A Critical Review. Nanoscale Adv. 1, 3807–3835. doi:10.1039/c9na00374f
Pan, S., Yao, M., Zhang, J., Li, B., Xing, C., Song, X., et al. (2020). Recognition of Ionic Liquids as High-Voltage Electrolytes for Supercapacitors. Front. Chem. 8. doi:10.3389/fchem.2020.00261
Panda, P. K., Grigoriev, A., Mishra, Y. K., and Ahuja, R. (2020). Progress in Supercapacitors: Roles of Two Dimensional Nanotubular Materials. Nanoscale Adv. 2, 70–108. doi:10.1039/c9na00307j
Pandey, G. P., and Hashmi, S. A. (2013). Studies on Electrical Double Layer Capacitor with a Low-Viscosity Ionic Liquid 1-Ethyl-3-Methylimidazolium Tetracyanoborate as Electrolyte. Bull. Mater. Sci. 36, 729–733. doi:10.1007/s12034-013-0511-y
Pohlmann, S., Olyschläger, T., Goodrich, P., Alvarez Vicente, J., Jacquemin, J., and Balducci, A. (2015). Azepanium-based Ionic Liquids as green Electrolytes for High Voltage Supercapacitors. J. Power Sourc. 273, 931–936. doi:10.1016/j.jpowsour.2014.09.167
Raj, J. J., Magaret, S., Pranesh, M., Lethesh, K. C., Devi, W. C., and Mutalib, M. I. A. (2018). Extractive Desulfurization of Model Fuel Oil Using Ester Functionalized Imidazolium Ionic Liquids. Separation Purif. Technology 196, 115–123. doi:10.1016/j.seppur.2017.08.050
Raj, J. J., Wilfred, C. D., Shah, S. N., Pranesh, M., Abdul Mutalib, M. I., and Lethesh, K. C. (2017). Physicochemical and Thermodynamic Properties of Imidazolium Ionic Liquids with Nitrile and Ether Dual Functional Groups. J. Mol. Liquids 225, 281–289. doi:10.1016/j.molliq.2016.11.049
Raza, W., Ali, F., Raza, N., Luo, Y., Kim, K.-H., Yang, J., et al. (2018). Recent Advancements in Supercapacitor Technology. Nano Energy 52, 441–473. doi:10.1016/j.nanoen.2018.08.013
Rennie, A. J. R., Sanchez-Ramirez, N., Torresi, R. M., and Hall, P. J. (2013). Ether-bond-containing Ionic Liquids as Supercapacitor Electrolytes. J. Phys. Chem. Lett. 4, 2970–2974. doi:10.1021/jz4016553
Rodrigues, R. D. P., de Castro, F. C., Santiago-Aguiar, R. S. d., and Rocha, M. V. P. (2018). Ultrasound-assisted Extraction of Phycobiliproteins from Spirulina (Arthrospira) Platensis Using Protic Ionic Liquids as Solvent. Algal Res. 31, 454–462. doi:10.1016/j.algal.2018.02.021
Roznyatovskaya, N., Rupp, A. B. A., Tübke, J., and Krossing, I. (2015). Sieving Effects in Electrical Double-Layer Capacitors Based on Neat [Al(hfip)4]−and [NTf2]−Ionic Liquids. Chem Electro Chem 2, 829–836. doi:10.1002/celc.201500024
Sampaio, A. M., Fileti, E. E., and Siqueira, L. J. A. (2019). Atomistic Study of the Physical Properties of Sulfonium-Based Ionic Liquids as Electrolyte for Supercapacitors. J. Mol. Liquids 296, 112065. doi:10.1016/j.molliq.2019.112065
Sathyamoorthi, S., Suryanarayanan, V., and Velayutham, D. (2015). Organo-redox Shuttle Promoted Protic Ionic Liquid Electrolyte for Supercapacitor. J. Power Sourc. 274, 1135–1139. doi:10.1016/j.jpowsour.2014.10.166
Seddon, K. R., Stark, A., and Torres, M.-J. (2000). Influence of Chloride, Water, and Organic Solvents on the Physical Properties of Ionic Liquids. Pure Appl. Chem. 72, 2275–2287. doi:10.1351/pac200072122275
Seki, S., Serizawa, N., Hayamizu, K., Tsuzuki, S., Umebayashi, Y., Takei, K., et al. (2012). Physicochemical and Electrochemical Properties of 1-Ethyl-3-Methylimidazolium Tris(pentafluoroethyl)trifluorophosphate and 1-Ethyl-3-Methylimidazolium Tetracyanoborate. J. Electrochem. Soc. 159, A967–A971. doi:10.1149/2.032207jes
Shahzad, S., Shah, A., Kowsari, E., Iftikhar, F. J., Nawab, A., Piro, B., et al. (2019). Ionic Liquids as Environmentally Benign Electrolytes for High-Performance Supercapacitors. Glob. Challenges 3, 1800023. doi:10.1002/gch2.201800023
Shao, Q., Tang, J., Lin, Y., Li, J., Qin, F., Yuan, J., et al. (2015). Carbon Nanotube Spaced Graphene Aerogels with Enhanced Capacitance in Aqueous and Ionic Liquid Electrolytes. J. Power Sourc. 278, 751–759. doi:10.1016/j.jpowsour.2014.12.052
Sharma, P., Minakshi Sundaram, M., Singh, D., and Ahuja, R. (2020). Highly Energetic and Stable Gadolinium/Bismuth Molybdate with a Fast Reactive Species, Redox Mechanism of Aqueous Electrolyte. ACS Appl. Energ. Mater. 3, 12385–12399. doi:10.1021/acsaem.0c02380
Siinor, L., Siimenson, C., Lust, K., and Lust, E. (2013). Mixture of 1-Ethyl-3-Methylimidazolium Tetrafluoroborate and 1-Ethyl-3-Methylimidazolium Iodide: A New Potential High Capacitance Electrolyte for EDLCs. Electrochemistry Commun. 35, 5–7. doi:10.1016/j.elecom.2013.07.023
Sillars, F. B., Fletcher, S. I., Mirzaeian, M., and Hall, P. J. (2012). Variation of Electrochemical Capacitor Performance with Room Temperature Ionic Liquid Electrolyte Viscosity and Ion Size. Phys. Chem. Chem. Phys. 14, 6094–6100. doi:10.1039/c2cp40089h
Sun, G., Li, K., and Sun, C. (2010). Electrochemical Performance of Electrochemical Capacitors Using Cu(II)-containing Ionic Liquid as the Electrolyte. Microporous Mesoporous Mater. 128, 56–61. doi:10.1016/j.micromeso.2009.07.027
Sundaram, M. M., and Appadoo, D. (2020). Traditional Salt-In-Water Electrolyte vs. Water-In-Salt Electrolyte with Binary Metal Oxide for Symmetric Supercapacitors: Capacitive vs. Faradaic. Dalton Trans. 49, 11743–11755. doi:10.1039/d0dt01871f
Susantyoko, R. A., Parveen, F., Mustafa, I., and Almheiri, S. (2019). MWCNT/activated-carbon Freestanding Sheets: a Different Approach to Fabricate Flexible Electrodes for Supercapacitors. Ionics 25, 265–273. doi:10.1007/s11581-018-2585-4
Tamailarasan, P., and Ramaprabhu, S. (2012). Carbon Nanotubes-Graphene-Solidlike Ionic Liquid Layer-Based Hybrid Electrode Material for High Performance Supercapacitor. J. Phys. Chem. C 116, 14179–14187. doi:10.1021/jp302785j
Tang, S., Baker, G. A., and Zhao, H. (2012). Ether- and Alcohol-Functionalized Task-specific Ionic Liquids: Attractive Properties and Applications. Chem. Soc. Rev. 41, 4030–4066. doi:10.1039/c2cs15362a
Thangavel, R., Kannan, A. G., Ponraj, R., Thangavel, V., Kim, D.-W., and Lee, Y.-S. (2018). High-energy green Supercapacitor Driven by Ionic Liquid Electrolytes as an Ultra-high Stable Next-Generation Energy Storage Device. J. Power Sourc. 383, 102–109. doi:10.1016/j.jpowsour.2018.02.037
Timofte, T., and Mudring, A.-V. (2006). Ionic Liquids with Weakly Coordinating Anions. Z. Anorg. Allg. Chem. 632, 2164. doi:10.1002/zaac.200670175
Tokuda, H., Tsuzuki, S., Susan, M. A. B. H., Hayamizu, K., and Watanabe, M. (2006). How Ionic Are Room-Temperature Ionic Liquids? an Indicator of the Physicochemical Properties. J. Phys. Chem. B 110, 19593–19600. doi:10.1021/jp064159v
Torrecilla, J. S., Rafione, T., García, J., and Rodríguez, F. (2008). Effect of Relative Humidity of Air on Density, Apparent Molar Volume, Viscosity, Surface Tension, and Water Content of 1-Ethyl-3-Methylimidazolium Ethylsulfate Ionic Liquid. J. Chem. Eng. Data 53, 923–928. doi:10.1021/je700523b
Tran, C., Lawrence, D., Richey, F. W., Dillard, C., Elabd, Y. A., and Kalra, V. (2015). Binder-free Three-Dimensional High Energy Density Electrodes for Ionic-Liquid Supercapacitors. Chem. Commun. 51, 13760–13763. doi:10.1039/c5cc04359j
Tsuzuki, S., Tokuda, H., Hayamizu, K., and Watanabe, M. (2005). Magnitude and Directionality of Interaction in Ion Pairs of Ionic Liquids: Relationship with Ionic Conductivity. J. Phys. Chem. B 109, 16474–16481. doi:10.1021/jp0533628
Vadiyar, M. M., Patil, S. K., Bhise, S. C., Ghule, A. V., Han, S.-H., and Kolekar, S. S. (2015). Improved Electrochemical Performance of a ZnFe2O4Nanoflake-Based Supercapacitor Electrode by Using Thiocyanate-Functionalized Ionic Liquid Electrolytes. Eur. J. Inorg. Chem. 2015, 5832–5838. doi:10.1002/ejic.201500870
Van Aken, K. L., Beidaghi, M., and Gogotsi, Y. (2015). Formulation of Ionic‐Liquid Electrolyte to Expand the Voltage Window of Supercapacitors. Angew. Chem. Int. Ed. 54, 4806–4809. doi:10.1002/anie.201412257
Varzi, A., Balducci, A., and Passerini, S. (2014). Natural Cellulose: A Green Alternative Binder for High Voltage Electrochemical Double Layer Capacitors Containing Ionic Liquid-Based Electrolytes. J. Electrochem. Soc. 161, A368–A375. doi:10.1149/2.063403jes
Venkatraman, V., Evjen, S., Lethesh, K. C., Raj, J. J., Knuutila, H. K., and Fiksdahl, A. (2019). Rapid, Comprehensive Screening of Ionic Liquids towards Sustainable Applications. Sustainable Energ. Fuels 3, 2798–2808. doi:10.1039/c9se00472f
Vogl, T., Menne, S., Kühnel, R.-S., and Balducci, A. (2014). The Beneficial Effect of Protic Ionic Liquids on the Lithium Environment in Electrolytes for Battery Applications. J. Mater. Chem. A. 2, 8258–8265. doi:10.1039/c3ta15224c
Wang, P., Zakeeruddin, S. M., Moser, J.-E., Humphry-Baker, R., and Grätzel, M. (2004). A Solvent-free, SeCN-/(SeCN)3- Based Ionic Liquid Electrolyte for High-Efficiency Dye-Sensitized Nanocrystalline Solar Cells. J. Am. Chem. Soc. 126, 7164–7165. doi:10.1021/ja048472r
Wang, Q., Zhang, T., Zhang, S., Fan, Y., and Chen, B. (2020). Extractive Desulfurization of Fuels Using Trialkylamine-Based Protic Ionic Liquids. Separation Purif. Technology 231, 115923. doi:10.1016/j.seppur.2019.115923
Wang, Y., Song, Y., and Xia, Y. (2016). Electrochemical Capacitors: Mechanism, Materials, Systems, Characterization and Applications. Chem. Soc. Rev. 45, 5925–5950. doi:10.1039/c5cs00580a
Wasserscheid, P., and Welton, T. (2008). Ionic Liquids in Synthesis. Second Edition. doi:10.1002/9783527621194
Welton, T. (2018). Ionic Liquids: a Brief History. Biophys. Rev. 10, 691–706. doi:10.1007/s12551-018-0419-2
Wolff, C., Jeong, S., Paillard, E., Balducci, A., and Passerini, S. (2015). High Power, Solvent-free Electrochemical Double Layer Capacitors Based on Pyrrolidinium Dicyanamide Ionic Liquids. J. Power Sourc. 293, 65–70. doi:10.1016/j.jpowsour.2015.05.065
Wu, B., Kuroda, K., Takahashi, K., and Castner, E. W. (2018). Structural Analysis of Zwitterionic Liquids vs. Homologous Ionic Liquids. J. Chem. Phys. 148, 193807. doi:10.1063/1.5010983
Xia, L., Yu, L., Hu, D., and Chen, G. Z. (2017). Electrolytes for Electrochemical Energy Storage. Mater. Chem. Front. 1, 584–618. doi:10.1039/c6qm00169f
Xu, C., Yang, G., Wu, D., Yao, M., Xing, C., Zhang, J., et al. (2021). Roadmap on Ionic Liquid Electrolytes for Energy Storage Devices. Chem. Asian J. 16, 549–562. doi:10.1002/asia.202001414
Yamazaki, S., Ito, T., Yamagata, M., and Ishikawa, M. (2012). Non-aqueous Electrochemical Capacitor Utilizing Electrolytic Redox Reactions of Bromide Species in Ionic Liquid. Electrochimica Acta 86, 294–297. doi:10.1016/j.electacta.2012.01.031
Yoshida, Y., Baba, O., and Saito, G. (2007). Ionic Liquids Based on Dicyanamide Anion: Influence of Structural Variations in Cationic Structures on Ionic Conductivity†. J. Phys. Chem. B 111, 4742–4749. doi:10.1021/jp067055t
Zarrougui, R., Hachicha, R., Rjab, R., Messaoudi, S., and Ghodbane, O. (2018). Physicochemical Characterizations of Novel Dicyanamide-Based Ionic Liquids Applied as Electrolytes for Supercapacitors. RSC Adv. 8, 31213–31223. doi:10.1039/C8RA05820B
Zhang, Q., Liu, S., Li, Z., Li, J., Chen, Z., Wang, R., et al. (2009). Novel Cyclic Sulfonium-Based Ionic Liquids: Synthesis, Characterization, and Physicochemical Properties. Chem. Eur. J. 15, 765–778. doi:10.1002/chem.200800610
Zhang, S., Brahim, S., and Maat, S. (2018). High-voltage Operation of Binder-free CNT Supercapacitors Using Ionic Liquid Electrolytes. J. Mater. Res. 33, 1179–1188. doi:10.1557/jmr.2017.455
Keywords: ionic liquids, supercapacaitor, electrolyte, design strategies, mechanism, pseudocapacitor, hybrid capacitor, challenges
Citation: Lethesh KC, Bamgbopa MO and Susantyoko RA (2021) Prospects and Design Insights of Neat Ionic Liquids as Supercapacitor Electrolytes. Front. Energy Res. 9:741772. doi: 10.3389/fenrg.2021.741772
Received: 15 July 2021; Accepted: 20 September 2021;
Published: 04 October 2021.
Edited by:
Jun Yan, Harbin Engineering University, ChinaReviewed by:
Manickam Minakshi, Murdoch University, AustraliaEmre Cevik, Imam Abdulrahman Bin Faisal University, Saudi Arabia
Copyright © 2021 Lethesh, Bamgbopa and Susantyoko. This is an open-access article distributed under the terms of the Creative Commons Attribution License (CC BY). The use, distribution or reproduction in other forums is permitted, provided the original author(s) and the copyright owner(s) are credited and that the original publication in this journal is cited, in accordance with accepted academic practice. No use, distribution or reproduction is permitted which does not comply with these terms.
*Correspondence: Kallidanthiyil Chellappan Lethesh, lethesh.chellappan@dewa.gov.ae, letheshkc@gmail.com