- 1School of Material Science and Engineering, Zhengzhou University, Zhengzhou, China
- 2School of Materials Science and Technology, Nanjing University of Aeronautics and Astronautics, Nanjing, China
- 3Henan Province Industrial Technology Research Institute of Resources and Materials, Zhengzhou University, Zhengzhou, China
- 4Henan Key Laboratory of High-temperature Structural and Functional Materials, Non-ferrous Metal Generic Technology Collaborative Innovation Center, Henan University of Science and Technology, Luoyang, China
As a high-efficiency energy storage and conversion device, lithium-ion batteries have high energy density, and have received widespread attention due to their good cycle performance and high reliability. However, currently commercial lithium batteries usually use organic solutions containing various lithium salts as liquid electrolytes. In practical applications, liquid electrolytes have many shortcomings and shortcomings, such as poor chemical stability, flammability, and explosion. Therefore, the liquid electrolyte has a great safety hazard. The use of solid electrolyte ensures the safety of lithium-ion batteries, and has the advantages of high energy density, good cycle performance, long life, and wide electrochemical window, making the battery safer and more durable, with higher energy density and simple battery Structural design. Solid electrolytes mainly include inorganic solid electrolytes and organic polymer solid electrolytes. Although both inorganic solid electrolytes and polymer solid electrolytes have their own advantages, as far as the existing research work is concerned, whether it is an inorganic system or a polymer system, a single-system solid electrolyte can never achieve the full performance of an ideal solid electrolyte. The composite solid electrolyte composed of active or passive inorganic filler and polymer matrix is considered as a promising candidate electrolyte for all-solid-state lithium batteries. Among many polymer systems, PEO-based is considered to be the most ideal polymer substrate. In this review article, we first introduced the structure, properties, and preparation methods of PEO-based polymer electrolytes. Furthermore, the researches related to the modification of PEO-based polymer solid electrolytes in recent years are summarized. The contribution of polymer structural modification and the introduction of additives to the ionic conductivity, electrochemical stability and mechanical properties of PEO-based solid electrolytes is described. Examples of different composite solid electrolyte design concepts were extensively discussed, such as inorganic inert nanoparticles/PEO, oxide/PEO, and sulfide/PEO. Finally, the future development direction of composite solid electrolytes was prospected.
Introduction
Due to the advancement of the global industrialization process, the rapid economic development and the large consumption of fossil fuels, tremendous effort has been made to develop low-polluting, environmentally friendly renewable energy (Sun et al., 2017; Chen et al., 2020; Ding et al., 2020). To make better use of renewable energy, the research and development of energy devices with the function of timely conversion and storage of electricity has become the primary task of researchers. As an efficient energy storage and conversion device, lithium-ion batteries (LIBs) with high energy and power density have received widespread attention, due to their good cycle performance and high reliability (Zheng et al., 2018). However, the current commercial LIBs usually use liquid electrolytes based on organic solutions containing various lithium salts. In practical applications, the liquid electrolyte has many shortcomings, such as poor chemical stability, flammability, and explosiveness, which leads in great potential security hazard. Many spontaneous explosion accidents, such as mobile phones, computers, and electric vehicles, have been reported, as the battery is overcharged or short-circuited. Therefore, researchers began to use solid state electrolytes (SSEs) instead of liquid organic electrolytes to prepare all-solid-state LIBs (ASSLIBs) as power sources for consumer electronics and automotive power systems (Lin et al., 2020; Yu et al., 2021a; Subramanian et al., 2021). The use of solid electrolytes can effectively improve the energy and power density of the battery. On the one hand, since the solid electrolyte is more than 80–90%, the weight is reduced, and the energy density is further improved. On the other hand, because the use of solid electrolytes can be compatible with high capacity positive and negative electrodes, such as metal lithium. The main idea is to avoid sub-reactions that continue to occur in the liquid electrolyte, and use the mechanical and electrical properties of solid electrolytes to inhibit the formation of lithium dendrites. Further, since the solid electrolyte is separated from the negative electrode material, the short-circuit effect of the lithium delegthrosis is not produced. All in all, solid electrolytes have better compatibility for lithium metal negatives. Compatible with high-capacity positive and lightweight battery systems, making battery energy density greatly improved. At the same time, the application of the solid electrolyte in the electric system makes this battery system more secure and long-circulating life. SSEs have the advantages of high energy density, wide operating temperature range, long cycle life, and wide electrochemical window. It also makes the battery safer and more durable, possesses higher energy density and simple battery structure design.
In recent years, the research of ASSLIBs have mainly focused on the development of ideal SSEs with high room temperature ionic conductivity, low electronic area specific resistance, good ionic selectivity, a wide electrochemical stability window, excellent chemical and thermal stability, and outstanding mechanical properties. Solid electrolyte performance requirements are shown in Figure 1. ASSLIBs are composed of anode, cathode, and SSE, all of which are solid materials. The structure is shown in Figure 2. The working principle of the all-solid-state lithium-ion battery is also the same as that of the liquid lithium-ion battery, that is, the chemical energy of the battery is converted into electrical energy through the discharge process to output the external circuit, and then the external power supply is reversed through the charging process. Restore the lithium-ion battery to its original state. The roles of SSE are not only to transfer metal lithium-ions, but also to replace separator to prevent short circuit between anode and cathode during charging and discharging. In addition, SSEs display a high mechanical strength, which can effectively inhibit the short circuit caused by the generation and penetration of lithium dendrites (Wan et al., 2018; Zhang et al., 2021a).
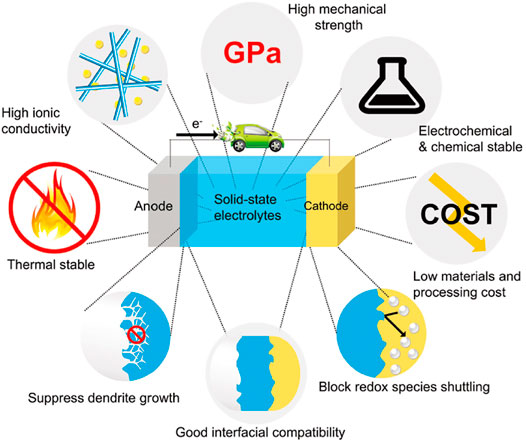
FIGURE 1. Performance requirements of solid electrolyte (Subramanian et al., 2021).
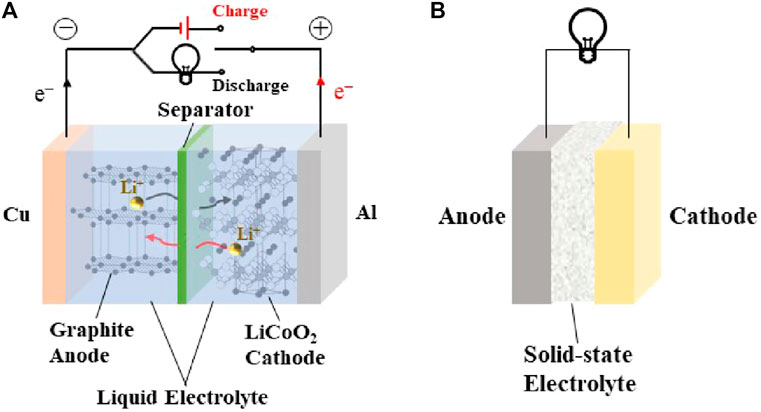
FIGURE 2. Comparison of the structure and transmission mechanism of (A) traditional liquid LIBs and (B) ASSLIBs.
For decades, scientists have been enthusiastic about ASSLIBs. A large number of studies have focused on all aspects of ASSLIBs, especially SSE materials. SSEs are the core component of ASSLIBs. Solid electrolytes mainly include inorganic solid electrolytes and organic polymer solid electrolytes. Inorganic solid electrolyte can maintain good chemical (electrochemical) stability, good mechanical strength, and wide electrochemical window in a wide temperature range. It can also inhibit the growth of lithium dendrites and avoid battery short circuits (Zhang et al., 2020a; Huang et al., 2020). At the same time, there are also problems such as complex preparation process, high cost, poor toughness, and poor mechanical properties. PSSE has good flexibility and stability of the contact interface with the electrode, which effectively solves the interface problem of ISSE. In addition, the polymer can be prepared on a large scale due to its good ductility, easy film formation, and simple preparation process, so it can be further commercialized (Yue et al., 2016). At the same time, the polymer material has medium mechanical strength (106–108 Pa), which can inhibit the growth of lithium dendrites. Among many polymer systems, PEO was first proposed.
In the 1980s, researchers discovered that PSSE based on polyethylene oxide (PEO) shows a good ion conductivity. However, its ion conductivity is generally low at room temperature (10–8–10–6 S cm−1), and its mechanical strength is not high (Long et al., 2016). When the battery assembled with lithium metal as anode is charged and discharged, the lithium dendrites easily penetrate the SSEs and cause short circuits. At the same time, the electrochemical window of PSSEs is narrow, which is not suitable for batteries with high voltage cathode materials (Baek et al., 2017; Zhang et al., 2017). Although a large number of scientific researchers have done a lot of modification work, a single-system SSEs can never achieve all aspects of an ideal SSEs, whether it is an inorganic system or a polymer system. So someone proposed to combine inorganic SSEs (ISSEs) with polymer SSEs to prepare composite SSEs (CSSEs) with excellent comprehensive performance, which became a research hotspot at that time (Yu et al., 2021b).
In order to improve the performance of PSSEs, the researchers successfully modified the PSSE by cross-linking polymerization or copolymerization starting from the polymer matrix (Cheng et al., 2021). Other substances can be added to the polymer matrix for modification of CSSEs (Tan et al., 2018; Mauger et al., 2019). The additives can be divided into inert additives and active additives. By adding a certain substance to form a composite phase, and then controlling the proportion and distribution of the composite phase, new molecular structures and ion transport channels can be generated, which can improve the electrochemical performance of the SSEs (Zhang et al., 2018; Yu and Manthiram, 2021). So researchers have a great research interest in this new type of composite PSSEs (CPSSEs) (Bao et al., 2018; Li et al., 2020; Feng et al., 2021). Among many polymer systems, PEO-based is considered to be the most commonly used polymer substrate. Next, the structure, performance, preparation and other aspects of PEO polymer solid electrolyte are described.
Introduction of PEO Polymer Solid Electrolyte
Structure and Preparation of PEO SSEs
In the 1970s, Wright’s research group first discovered that low-molecular-weight PEO (polyethylene glycol) can combine with lithium salt (Wright, 1975). When the lithium salt was dissolved by PEO, the formed ions can move with the polymer chain segment, thereby enhancing conductivity. Subsequently, in 1979, Armand et al. (Wu and Chiang, 2007) successfully prepared an all-solid-state polymer lithium-ion battery based on PEO electrolyte. PEO is a typical semi-crystalline polymer. Because the oxygen of etheroxy unit on its chain link couples with other cations such as Li+, the PEO polymer matrix can dissolve many different kinds of lithium salts (Van der Ven al., 2013). The conductive process of the PEO-based electrolyte is mainly due to the movement of the amorphous chain links in the polymer, which causes Li+ to continuously complex and de-complex with the ether oxygen groups on the PEO chain, so that lithium ions can migrate between chains (Walter et al., 2000; Ma et al., 2019). Because of the special ion transport mechanism, the ion conductivity of PSSEs is mainly affected by the movement ability of the polymer matrix. PEO is easy to crystallize at room temperature and has poor link mobility, so the room temperature ionic conductivity is only 10–7 S cm−1. A number of studies have shown that PEO matrix can be modified by blending, copolymerization and cross-linking methods to improve its ionic conductivity. PEO chemical stability is better compared to other polymers, and it is not easy to explain the reaction, and can be combined with a variety of lithium salts. However, if PMMA, polyisobutylene is prone to formation reaction.
The special molecular structure of the basic unit of PEO is (CH2-CH2-O) n. PEO usually has two structures: monoclinic and triclinic. Generally speaking, the body material of PEO exists in the monoclinic system. The crystallinity of the material at room temperature is about 85%, and the chemical stability is high (Armand, 1983). PEO is a crystalline, thermoplastic, water-soluble polymer. The repeating unit in the PEO macromolecular chain structure is an ethoxy group. Generally speaking, PEO has a wide molecular weight distribution (Lightfoot et al., 1993). It is customary to refer to products with a relative molecular mass of less than 20,000 as polyethylene glycol (PEG), which is a viscous liquid. With a relative molecular mass greater than 20,000, PEO is white powder. PEO has a highly ordered structure, and its melting point is generally around 65°C. In addition, the ethoxy group of the repeating unit of PEO contains ether oxygen bonds, and the oxygen atoms in the ether oxygen bonds contain lone pairs of electrons (Fenton et al., 1973). Therefore, PEO has a strong affinity for hydrogen bonds and can interact with many small molecular compounds, polymers, and inorganic nanoparticles form complexes. In addition, PEO has a relatively high dielectric constant and a good ability to dissolve lithium salts (Shriver et al., 1981).
PSSEs also require conductive lithium salts to provide the carriers required for conduction. The added lithium salt forms a mixture with the polymer, which reduces the glass transition temperature and melting point, facilitates the migration of ions, and improves ion conductivity (Agrawal and Pandey, 2008). The choice of lithium salt often has certain selection rules, because not all lithium salts can be dissolved in the polymer matrix. And after dissolution, they may not be able to migrate as free ions. Therefore, when choosing a lithium salt, its solubility in the polymer matrix is very important. The amount of lithium salt directly determines the concentration of carriers in the PSSE and has a great influence on the conductivity. Within a certain range, increasing the concentration of lithium salt can increase the carrier concentration, thereby increasing the conductivity (Singh and Gupta, 1998).
The preparation of PSSEs is mainly divided into physical methods and chemical methods (Chandrasekhar, 1998; Wei and Shriver, 1998). The physical method refers to a solution formed by dissolving polymer matrix, lithium salt and other additives (such as plasticizers, inorganic fillers, etc.) in a solvent, and then coating by casting, and then controlling the environment temperature, humidity and pressure to let the solvent evaporate, and then obtain PSSE membranes with different morphologies. Chemical method refers to a mixture of polymer matrix, lithium salt and other additives (such as plasticizers, inorganic fillers, etc.), infiltrated into non-woven fabrics or separators, and then initiated polymerization methods (such as UV light initiation or thermal initiation) to prepare PSSE membrane.
The Working Principle of PEO SSEs
PEO has a relatively high proportion of crystalline phase regions at room temperature. The study found that there are spiral-structured pipes in the crystalline PEO, and ions are conducted through the vacancies in the pipes. The PEO amorphous region conduction model is shown in Figure 3. Because PEO contains a large number of oxygen atoms that can provide lone pairs of electrons, it can form weak complexes with metal ions and promote the movement of lithium ions through the movement of molecular chains. PEO has weak conductivity, but the oxygen atom of the etheroxy group on the copolymer molecule has 2 pairs of lone pairs of electrons and a strong coordination ability. Li+ can interact with the ether oxygen group on the PEO amorphous segment to complex and de-complex to achieve Li+ migration. The molecular structure and spatial structure of PEO determine that it cannot only provide high enough electron-donating groups, but also have flexible polyether segments, which can effectively dissolve cations. PEO has the simplest framework structure and the chemical and electrochemical stability is better.
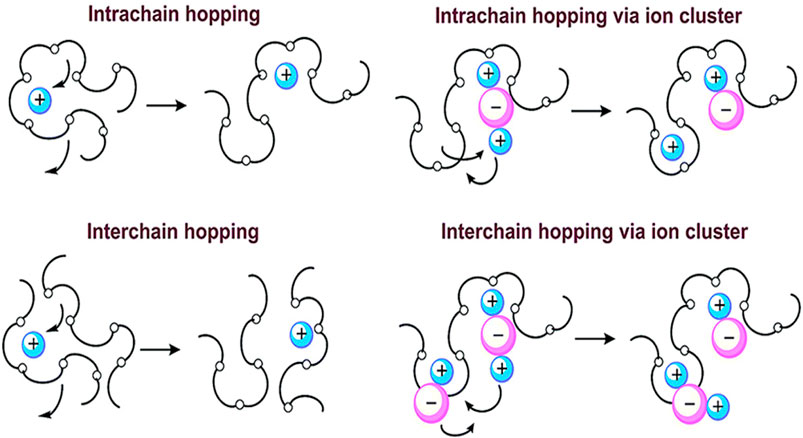
FIGURE 3. Lithium-ion conduction mechanism of PEO-based polymer SSEs (Bao et al., 2018).
Other types of PSSEs, such as PAN-based, PVDF-based electrolytes, have also been studied very early, but the overall performance of these electrolytes is not as good as that of PEO-based polymers. Due to its narrow electrochemical window, PAN-based electrolytes cannot meet the requirements of high voltage and high specific volume systems, so it will not become the mainstream research object. PMMA-based electrolytes, due to its poor mechanical strength, cannot act as the main force of all-SSEs. PVDF-based electrolytes, due to its high dielectric constant, is conducive to the dissociation of lithium salt, and is an ideal polymer matrix for preparing PSSEs. However, PVDF polymer structure is symmetrical and regular, and is easy to form a crystalline structure, which is detrimental to ion conductivity. Among many polymer systems, PEO-based is considered to be the most ideal polymer matrix. PEO has a high dielectric constant and a strong ability to dissociate lithium salts. Its basic unit has a special molecular structure (CH2-CH2-O)n, which can form complexes with many lithium salts (such as LITFSI, LiClO4), and then promote the transmission of Li+ through segment movement. In addition, because PEO can dissolve a variety of salts through the interaction of its ether oxygen functional groups and cations, it can be used in a stand-alone form in the design of LIBs without modifying the current battery manufacturing process.
The Modification of PEO-Based CSSEs
The single type of SSE is difficult to fully meet the challenging requirements of ideal SSEs, especially through a simple and environmentally friendly way. Both inorganic SSEs and polymer SSEs have their own advantages, but their shortcomings cannot be ignored. These shortcomings greatly limit their applications. CSSEs can combine the advantages of ISSEs and PSSEs to improve the ionic conductivity, electrochemical window, mechanical strength, chemical stability, good contact with electrode materials, compatibility and stability, and cycle performance of SSE (Zhang et al., 2021b). In recent years, a large number of scientific researchers have actively invested in the research of composite SSEs and have done a lot of work. CSSE is a kind of polymer as the matrix, through the addition of other substances to compound to form a new composite material.
Modification of PEO-Based CSSEs
PEO-based PSSE is the most studied type of solid PSSE. However, due to its low room temperature ionic conductivity (10−5–10–7 S/cm), narrow electrochemical window and poor mechanical strength at room temperature, its practical application has been greatly restricted. The low ion conductivity of PEO-based SSEs is mainly due to its easy crystallization and high crystallinity.
In recent years, many scientific researchers have used various modification methods to enhance the performance of PEO-based PSSEs, so as to meet the requirements of practical applications. Due to the high crystallinity of the PEO-based SSEs, we can increase the ion mobility by reducing its crystallinity, thereby improving the ion conductivity. At present, our research methods for its modification mainly include blending/copolymerization between polymers and introducing additives for doping modification (Goodenough and Park, 2013). These two methods can effectively increase the number of amorphous phases, reduce the crystallinity of the PEO PSSE, and also improve the mechanical properties. In addition, the introduction of other substances will also reduce the glass transition temperature of the PEO-based PSSE, making it suitable for use in LIBs.
Blending/Copolymerization of Polymers
In recent years, in order to suppress the adverse effects of crystallization on ionic conductivity and enhance the mechanical strength to inhibit the growth of lithium dendrites, people have been committed to the development of new amorphous polymer matrix, including polymer blending, copolymerization, crosslinking, etc. These methods can reduce the crystallinity or Tg of the polymer system by changing the polymer structure, and thus improve electrochemical stability, enhance mechanical strength, and inhibit the formation of lithium dendrites.
Blending/copolymerizing PEO with one or more organic polymers is an effective way to improve the performance of SSEs. Rocco et al. blended PEO and polymethyl vinyl ether maleic acid (PMVE-Mac) and added lithium salt LiClO4 to obtain an organic polymer SSEs (Rocco et al., 2002).Compared with pure PEO, the study showed that the hydrogen bond formed between PMVE-Mac and PEO has a larger free volume and stronger segment movement ability. At room temperature, the maximum conductivity of the mixed SSEs reaches 10–5 S cm−1, and the electrochemical stability window has also been significantly improved. In 2014, the Geoffrey W. Coates research group used polyethylene (PE) and PEO to cross-link and polymerize, and added lithium salt LiTFSI to obtain composite materials with lithium ion conductivity as high as 10–4 S/cm at room temperature (Khurana et al., 2014). This is mainly because the skeleton structure provided by the PE material can prevent the crystallization of PEO and improve its lithium ion conductivity. At the same time, the polymer has a certain mechanical strength and a shear modulus of the same order of magnitude as that of lithium metal, which can effectively inhibit the growth of lithium dendrites. Juan Shi et al. used PEO/PMMA/P (VDF-HVP) copolymerization to prepare a gel-type PSSE (Shi et al., 2018). Because the addition of PMMA destroyed the crystallinity of PEO, its ionic conductivity reached 0.81 mS/cm at 25°C. It can be seen from the electrochemical test results that the coulombic efficiency of the battery with the modified electrolyte after 100 cycles is still very good. From the perspective of interface stability, it has remained stable after five cycles. He et al. introduced carbonate units into the PEO chain, and then blended and cast the block polymer PTEC and LiTFSI on the cellulose membrane to obtain a composite solid PSSE membrane with good mechanical properties (He et al., 2017). Experiments show that the introduction of carbonate repeat units successfully reduces the crystallinity of the SSEs. The ion conductivity reaches 1.12 × 10–5 S cm−1 at 25°C, and the electrochemical window is about 4.5 V. Ling Xu et al. (Xu et al., 2020) added polyvinyl alcohol (PVA) in different proportions to the PEO matrix, blended by solution casting method, and added LiTFSI to obtain a composite PSSE membrane. The specific research results are shown in Figure 4. Tests show that the conductivity of the PSSE with a PVA content of 30% is 0.635 × 10–3 S/cm, and the electrochemical window and interface stability are improved at the same time. The main reason is that the molecular weight of PVA is smaller than that of PEO. PVA can be used as another Li+ conductive framework. It can increase the concentration of free Li+ microscopically, and destroy the regularity of PEO segments, reduces the crystallinity of PEO, and improve lithium-ion conductivity of the electrolyte.
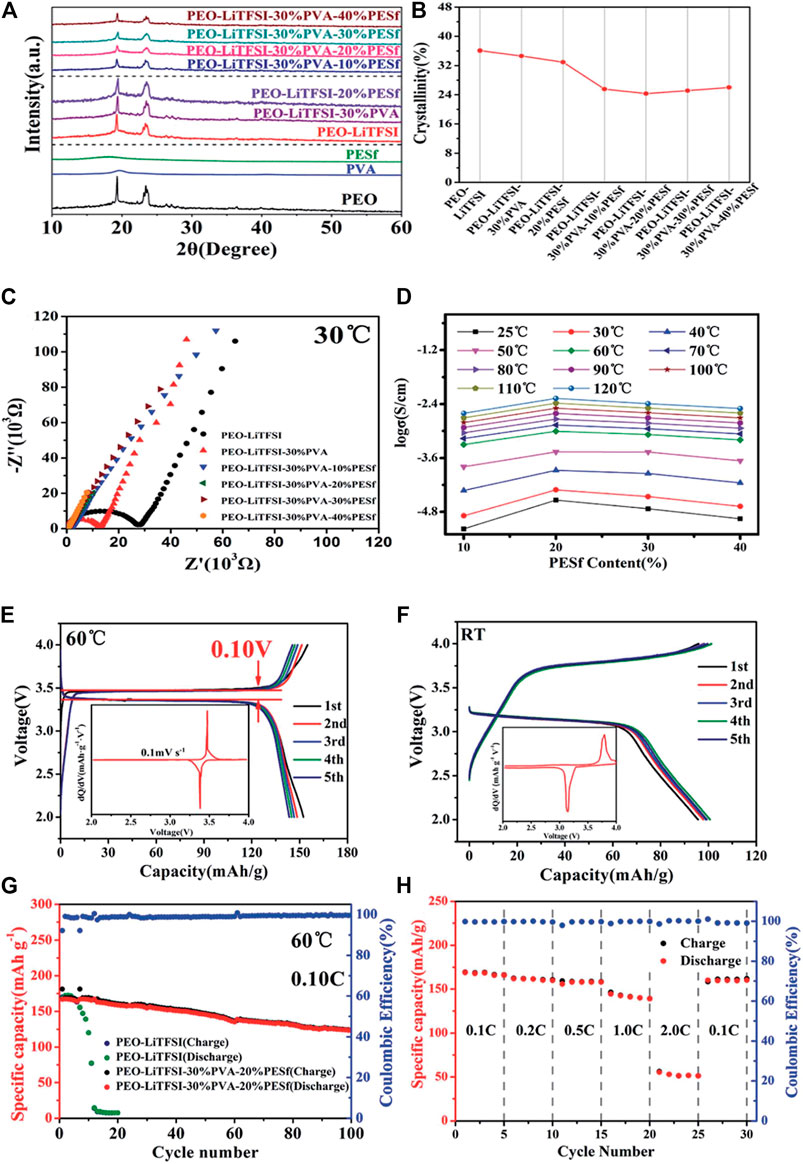
FIGURE 4. (A) XRD patterns of polymer membranes, (B) the crystallinity calculated from XRD patterns; (C) Nyquist plots, (D) ionic conductivity of polymer membranes; Charge/discharge curves for the fifirst five cycles at (E) 60°C; (F) room temperature; (G) Galvanostatic profifiles of LiFeO4/CPE/Li cell and LiFeO4/PEO–LiTFSI/Li cell. (H) The rate capacity of LiFeO4/CPE/Li cell from 0.1 to 2.0°C at 60°C. Reproduced from ref. 44 with permission from RSC Advances copyright 2020.
Doping With Inert Additives
CSSEs is a kind of polymer PEO as the matrix, by adding other substances to improve the performance of the PSSE, thereby forming a new CSSE. The main types of additives introduced are inert inorganic fillers and active inorganic fillers. Inorganic inert fillers, include nano-scale Al2O3, SiO2, TiO2, ZrO2, FeO3, B2O, etc. (Das and Ghosh, 2015; Zhu et al., 2019) For PSSEs, the addition of inorganic inert fillers can hinder the regular arrangement of the polymer segments, keep them in a highly amorphous state, effectively inhibit the crystallization of the polymer matrix, and improve the mobility of the polymer segments. In addition, research has found adding nano-ceramic powder to the polymer-based electrolyte can not only act as a solid plasticizer, inhibit the crystallization kinetics, promote the retention of the amorphous phase to sub-ambient temperature, but also affect the solvent and cation mobility. Nano-sized filler particles serve as cross-linking sites between chain segments and ions, which broaden the ion transport channel and promote ion transport, thereby increasing the ionic conductivity (Sun et al., 2016; Baek et al., 2017). The addition of inorganic inert fillers can also enhance the mechanical and thermal properties of the polymer matrix, at the same time, it can adsorb trace impurities and water in the system, improve the interface between the electrolyte system and the metal lithium, and reduce the polarization and corrosion of the electrode.
In 1998, Scrosati et al. added nano-sized TiO2 and Al2O3 ceramic powder to the PEO matrix. The ion conductivity increased by 1–2 orders of magnitude, and the mechanical properties were also enhanced (Croce et al., 1998). Takeda et al. studied the effect of barium titanate ceramic particles on PEO-Li(CF3SO2)2N-LiPF6 solid PSSE (Yasuo et al., 2009). It was found that barium titanate with high dielectric constant not only destroys the crystallization of PEO, but also promotes the decomposition of lithium salt. The PSSE also exhibits good reversible charge and discharge performance and good interface stability with lithium metal electrodes. Chen et al. incorporated self-made silica aerogel powder (SAP) into the PEO-LiClO4 electrolyte system (Chen-Yang et al., 2008). SAP has a higher surface area and pore volume than conventional silica particles, and can be well dispersed in the electrolyte matrix, thereby significantly reducing the crystallinity of the system. When the EO/Li + molar ratio is 6 and 2wt% SAP is contained, the ionic conductivity of the composite solid electrolyte is increased by three times. In addition, the lithium-ion transfer number of the SSEs is as high as 0.67 at 70°C. Masoud et al. (Masoud et al., 2013) prepared CPSSE by doping self-made LiAlO2 nano-particle ceramic filler into PEO. It was found that the room temperature ionic conductivity of the electrolyte modified with LiAlO2 nanoparticles increased by two orders of magnitude, reaching 9.76 × 10–5 S cm−1. In addition, the electrochemical stability, cycle performance and specific charge-discharge capacity of the battery assembled by the electrolyte are improved. Wang et al. (Wang et al., 2007) designed porous SBA-15 nanoparticles that can realize the rapid conduction of lithium ions and introduced them into polymers to prepare SPSSEs. Because SBA-15 has active sites on the surface, it interacts with the anions of LiClO4 to generate Lewis acid-base interactions, which greatly promotes the dissociation of lithium salts and improves the electrochemical performance of the electrolyte. The ionic conductivity of the SSEs can reach 1.5 × 10–4 S cm−1 at room temperature, the electrochemical window is stable at 4.7 V, and the battery assembled with it at 80°C has a specific charge-discharge capacity of 120 mA h g−1. The above research shows that the introduction of inert fillers can improve the electrochemical performance and the mechanical performance of SSE.
Besides, the fact that sometimes the use of both active and inert additives is possible. Succinonitirle as one of the most significant PEO plasticizers, Hilal et al. (Al-Salih et al., 2020) proposed a polymer-rich quaternary composite solid-state electrolyte composed of PEO-LiTFSI-LLTO-SN 70 wt% PEO, 12 wt% LiTFSI, 9 wt% LLTO and 9 wt% SN was made using traditional solution.
Casting technique and its ionic conductivity reached slightly higher than 10–3 S cm−1 at 55°C. At the same time, there is a big contribution of serotonitrile as a plasticizer as a plasticizer.
In addition, organic porous materials, such as carbon nanotubes, graphene, and MOF, can also be used as additives to improve the ionic conductivity of polymer SSEs (Park et al., 2017; Wang et al., 2018). The structure of the porous material itself can provide a three-dimensional transmission channel for lithium ions, so that it has good electrochemistry performance. In addition, the porous material can adsorb small molecules and improve the stability of the interface between the electrolyte and the electrode.
Doping With Active Additives
The addition of the active solid electrolyte effectively reduces the crystallinity of PEO to provide more amorphous zones for ion conductance; itself can directly provide a large number of Li +, improve the concentration of free Li +, while also promoting ionic dealing, change the polymer structure, increase the ion transmission channel, and initiate a new way of composite conduction, thereby increasing total ion conductivity of the entire system (Commarieu et al., 2018).
Currently commonly used active inorganic electrolyte fillers are mainly divided into oxide SSE and sulfide SSE.
Oxide SSEs Active Filler
Oxide SSEs have better ionic conductivity and higher air stability, and generally a relatively wide electrochemical window. Oxide SSEs are classified according to the crystal structure, which can be divided into crystalline electrolytes and glass (amorphous) electrolytes. Crystalline electrolytes include garnet type SSEs, perovskite type SSEs, NASICON type etc. Glassy electrolytes include inverse perovskite SSEs and LiPON thin film SSEs.
The general formula of the garnet-type SSE can be expressed as Li3+xA3B2O12. It is note that A is an eight-coordinate cation and B is a six-coordinate cation. AO8 and BO6 are staggered and connected in a coplanar way to form a three-dimensional skeleton, and the skeleton gap is filled with octahedral vacancies and tetrahedral vacancies composed of O. In 2007, Muruga et al. (Murugan et al., 2007) found that Li7La3Zr2O12 (LLZO) SSE has high ionic conductivity and wide voltage window.
The general molecular formula of the solid electrolyte of the perovskite structure can be expressed as ABO3, where the A-site cation is generally the rare earth element La, Nd or alkali metals and alkaline earth metals, and the B-site cation is generally a transition metal with a small ion radius, such as Ti. The perovskite electrolyte has the advantages of stable structure, simple preparation process, and wide range of variable composition. For example, LLTO materials exhibit four different crystal structures (cubic phase, tetragonal phase, orthogonal phase and hexagonal phase). The conductivity of tetragonal LLTO particles at room temperature reaches 10−3 S/cm.
In 1976, Goodenough et al. (Goodenough et al., 1976) reported the Na3Zr2Si2PO12 sodium ion SSE with the NASICON structure for the first time. The electrolyte can not only conduct sodium, but also quickly conduct lithium ions. The general structure of fast lithium-ion conductor is LiT2(PO4)3, and T is Ti, Ge, Zr, etc. Using trivalent ions Al, Cr, Ga, etc. for substitution doping, Li1+xMxTi2−x(PO4)3 is obtained, of which Al doped Li1+xAlxTi2−x(PO4)3 (LATP) has high conductivity (Kotobuki et al., 2013; Kotobuki and Koishi, 2013). In addition, Ge and metal Li have high stability. Replace Ti with Ge to obtain another NASICON structure Li1+xAlxGe2–x(PO4)3(LAGP), which has high chemical stability, ion conductivity and electrochemical window (Thokchom and Kumar, 2008). The inverse perovskite structure SSE has the characteristics of low cost, environmental friendliness, high room temperature ionic conductivity (2.5 × 10−2 S/cm), excellent electrochemical window and thermal stability, and stability with metal Li.
The inverse perovskite structure lithium-ion conductor can be expressed as: Li3–2xMxHalO, where M is a high-valent cation such as Mg2+, Ca2+, Sr2+ or Ba2+, and Hal is the element Cl or I. For the first time, Bates et al. (Bates et al., 1992) obtained a lithium phosphorous oxynitride (LiPON) film with a high-purity LiPO4 target by radio frequency magnetron sputtering in high-purity nitrogen. The thickness of the thin-film electrolyte obtained by the magnetron sputtering method is less than 1 μm, and the resistance is small, so it is suitable for thin film lithium-ion batteries. The electrolyte material has good overall performance, the room temperature ion conductivity is 2.3 × 10−6 S/cm, the electrochemical window reaches 5.5 V, the thermal stability is high, and it is compatible with LiMn2O4, LiCoO2 and other common positive electrodes and metal Li negative electrodes good. Several inorganic SSE structures are shown in Figure 5.
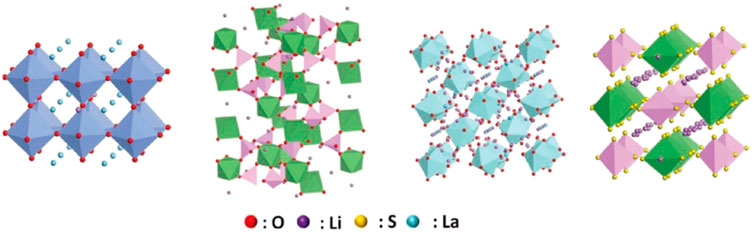
FIGURE 5. Several inorganic SSE structures (from left to right: perovskite type, NASICON type, garnet type, sulfide type).
Garnet Type Active Filler
Hu et al. used cellulose template method to prepare PEO-LLZO composite PSSE on a large scale. The three-dimensional ordered LLZO framework not only provides ion channels, but also solves the inherent interface problems of LLZO by combining with PEO. The conductivity is still as high as 1.12 × 10–4 S/cm. The article by Li Hong’s group on the effect of nano-scale LLZTO on the ionic conductivity of PEO-based PSSEs shows that compared with micron-scale LLZTO particles. the nano-scale LLZTO particles have a more significant improvement in the ionic conductivity of PEO-based PSSEs (Zhang et al., 2016). In the PEO-LLZTO, the PSSEs of different particle sizes of LLZTO and PEO composite have different room temperature ionic conductivity. The author believes that the increase in ion conductivity is related to the size of LLZTO particles. Nano-scale LLZTO particles have a larger specific surface area and can form more lithium ion channels at the interface between LLZTO and PEO. Many researchers have designed 3D structured ceramic electrolytes as active fillers. Fu et al. (Fu et al., 2017) proposed that a three-dimensional LLZO nanofiber network prepared by electrospinning and high temperature annealing was immersed in a PEO-LiTFSI polymer SSEs and successfully prepared a composite SSEs. In this composite SSEs, the nanofiber network not only provides a long-range route for lithium-ion transport, but also provides a new type of lithium-ion transport network that further enhances the strength of the polymer matrix. The lithium-ion conductivity of this composite SSEs at room temperature can be as high as 2.5 × 10–4 S/cm, and the symmetrical Li/Li+ battery can be cycled stably within 1,000 h without dendrite formation. Choi et al. (Choi et al., 2015) added Li7La3Zr2O to the PEO matrix to prepare a new composite electrolyte material. The test shows that it has an ionic conductivity of 0.44 mS cm−1 at 55°C. In 2019, He et al. (He et al., 2019) prepared a composite SSEs composed of Li6.4La3Zr1.4Nb0.6O12 filler and PEO. The specific research results are shown in Figure 6. The ionic conductivity of the solid composite electrolyte was significantly increased to 1.4 × 10–3 S/cm (60°C), the electrochemical window was increased to 5.2 V, and the weight ratio of Li6.4La3Zr1.4Nb0.6O12 powder to PEO was added. It is 0.5:1. The solid battery using composite electrolyte can work at room temperature, and the capacity retention rate after cycling is 99%. The improvement of performance is mainly due to the comprehensive effect of each component in the composite material: the addition of filler effectively reduces the crystallinity of PEO and provides more amorphous regions for ion conduction; the filler acts as a fast ion conductor in the composite material to enhance electrochemical stability; the polymer matrix forms a close surface contact with the filler and the electrode, reducing the volume and interface resistance. In this type of composite electrolyte, PEO-LLZO is a more active electrolyte.
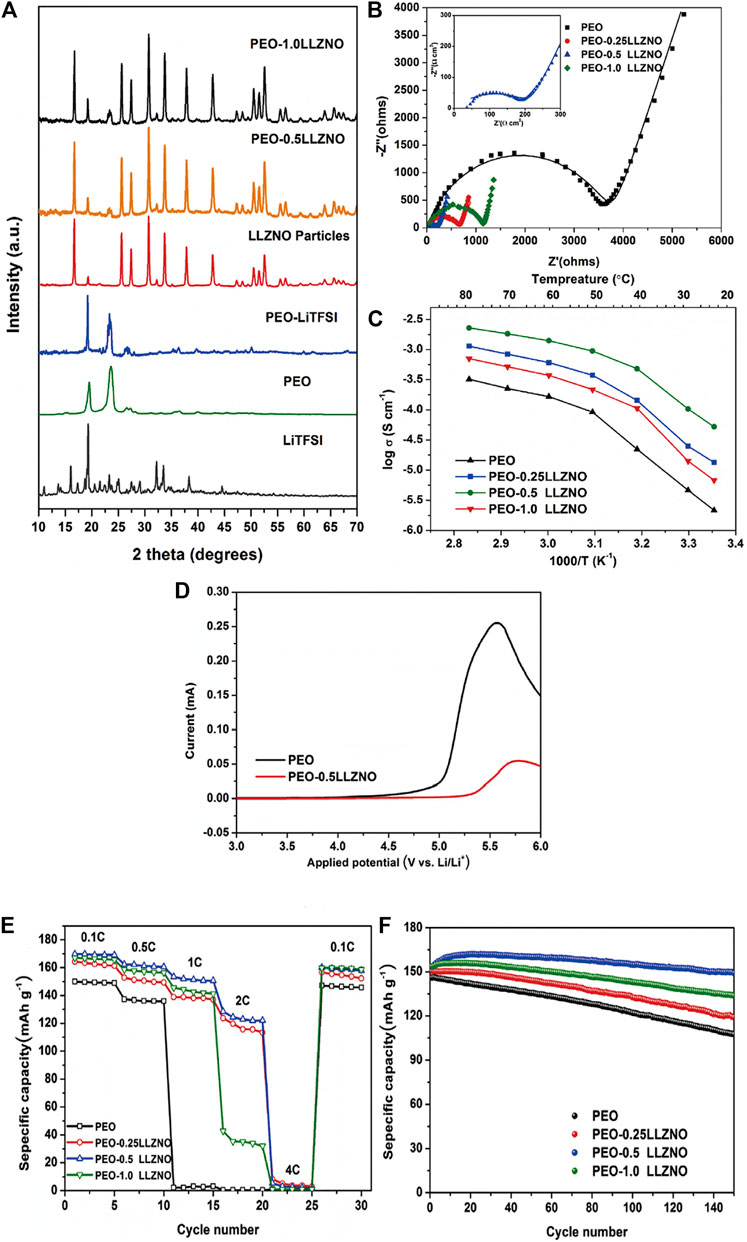
FIGURE 6. (A) XRD patterns of each component of the composite electrolytes, (B) EIS curves of pure PEO and its composite polymer electrolytes at ambient temperature, (C) Arrhenius plots for the ionic conductivities of the composite electrolytes, (D) Linear sweep voltammograms of pure PEO and representative PEO-0.5LLZNO, (E) rate performance of LiFePO4/PEO/Li and LiFePO4/CPE/Li at difffferent rates (0.1, 0.5, 1, 2, 4°C), (F) cycling performance of LiFePO4/PEO/Li and LiFePO4/CPE/Li batteries at the rate of 0.5 and 60°C. Reproduced from ref. 66 with permission from Composites Science and Technology., copyright 2019.
Studies have shown that the content of the ceramic additive in the polymer has a certain effect on the properties of the composite solid electrolyte. Chen Long et al. (Chen et al., 2018a) proposed a composite solid electrolyte membrane PEO-LITFSI-LLZTO by thermal pressing without any organic solvent. The content of the LLZTO powder is changed from 0 to 80% by weight. The results show that when the content of LLZTO is increased to 80% by weight, the ionic conductivity of the composite electrolyte has decreased. Combined with SEM image and DSC test results: As the LLZTO content increases, the composite electrolyte has a crack, flexibility deterioration, and the interface of the ceramic and the polymer, hinders the transmission of lithium ions. It is understood that cracks, flexibility varies in electrolytes, and interface problems between ceramic and polymers limits the utilization of ceramics in the polymer.
Cai Dan et al. (Cai et al., 2021) introduced an ionic liquid containing the PVDF-HFP electrolyte as an intermediate layer in the PEO-based electrolyte/electrode interface, and designed a sandwich IPI composite electrolyte by a simple solution casting method. After introducing the IPL intermediate layer, the severe interface reaction between the PEO-based electrolyte and the LCO cathode is significantly inhibited, and the IPL intermediate layer facilitates the formation of a stable electrolyte/electrode interface. This composite electrolyte exhibits excellent electrochemical properties and thermal stability.
Perovskite Type Active Filler
Zhu et al. (Zhu et al., 2018) found that by adding fibrous filler LLTO (Li0.33La0.557TiO3) to the PEO/LiTFSI electrolyte system, the crystallinity of the PSSE was effectively reduced, and the electrochemical window and ion migration number were obvious increase, when the filler is added to 5% mass fraction, the ion conductivity reaches 5.53 × 10–5 S/cm at room temperature and has better cycle and rate performance. Wu et al. (Wu et al., 2020) will perovskite La0.8Sr0. 2Ga0.8Mg0.2O2.55 (LSGM) is used as a ceramic filler for PEO (PEO) based polymers. The specific research results are shown in Figure 7. The oxygen vacancies on the body and particle surfaces of this material will increase the oxide surface and lithium in the polymer. The interaction between salt anions promotes the migration of Li+ ions. At 30°C, the conductivity of the composite electrolyte is higher than 10–4 S/cm. In this type of composite electrolyte, PEO-LLTO is a more active electrolyte.
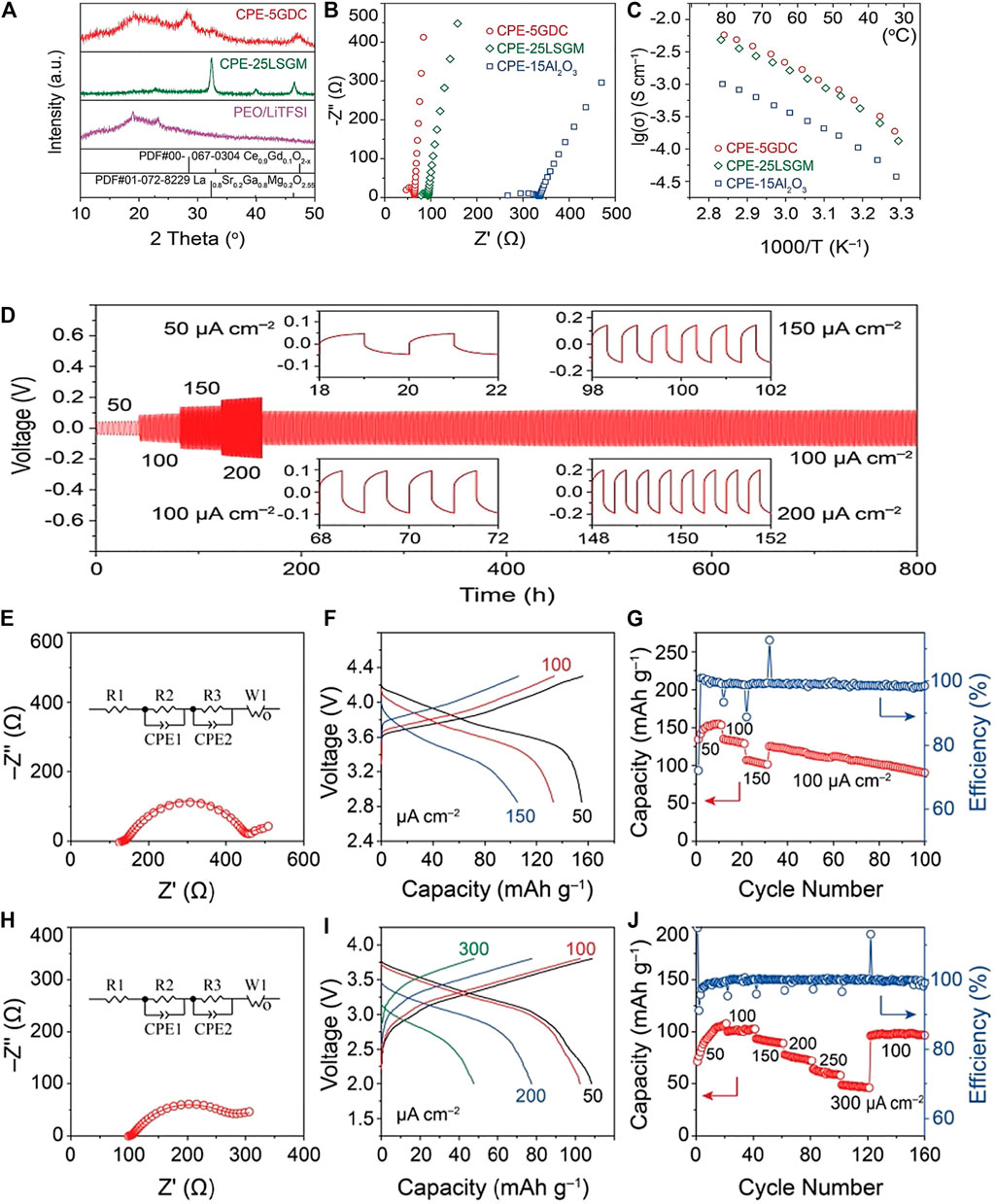
FIGURE 7. (A) XRD patterns, (B) impedance plots (308°C), and (C) Arrhenius plots of Li+ conductivities of the composite electrolytes ([EO]/[Li] ratio n = 10), (D) The cycling performance of the symmetric Li/Li cell with different current densities at 35°C, All-solid-state Li/NMC cell cycled at 358°C. (E) Electrochemical impedance plots, (F) charge/discharge voltage profiles, and (G) capacity retention and cycling efficiency. All-solid-state Li/polyaniline cells cycled at 35°C, (H) Electrochemical impedance plots, (I) charge/discharge voltage, and (J) capacity retention and cycling efficiency. Reproduced from ref.70 with permission from Angew Chem Int Ed Eng., copyright 2020.
NASICON Type Active Filler
Recently, Zhao et al. (Zhao et al., 2016a) found that the ionic conductivity of the composite electrolyte with Li1.5Al0.5Ge1.5(PO4)3 (LAGP) as the active filler was significantly improved. The results show that LAGP particles have a positive effect on the ionic conductivity, lithium-ion transference number, electrochemical stabilities and mechanical properties. Among the PEO/LAGP hybrid electrolytes, the PEO-20%LAGP hybrid electrolyte exhibits a maximum ionic conductivity of 6.76 × 10–4 S/cm and an electrochemical window of 5.3 V at 60°C. Most composite electrolytes are prepared by casting, but the agglomeration of fillers in composite electrolytes prepared by casting limits the further improvement of ionic conductivity. Jun Cheng et al. (Cheng et al., 2020) provided a simple solvent-free cold pressing method to prepare well-dispersed LAGP@PEO composite SSEs. The specific research results are shown in Figure 8. The ionic conductivity of LAGP@PEO composite electrolyte can reach 4.4 × 10–5 S/cm at room temperature, which is nearly an order of magnitude higher than that of cast electrolyte (3.3 × 10–6 S/cm). The overall solid-state battery shows excellent cycle performance and good rated performance at 50°C.
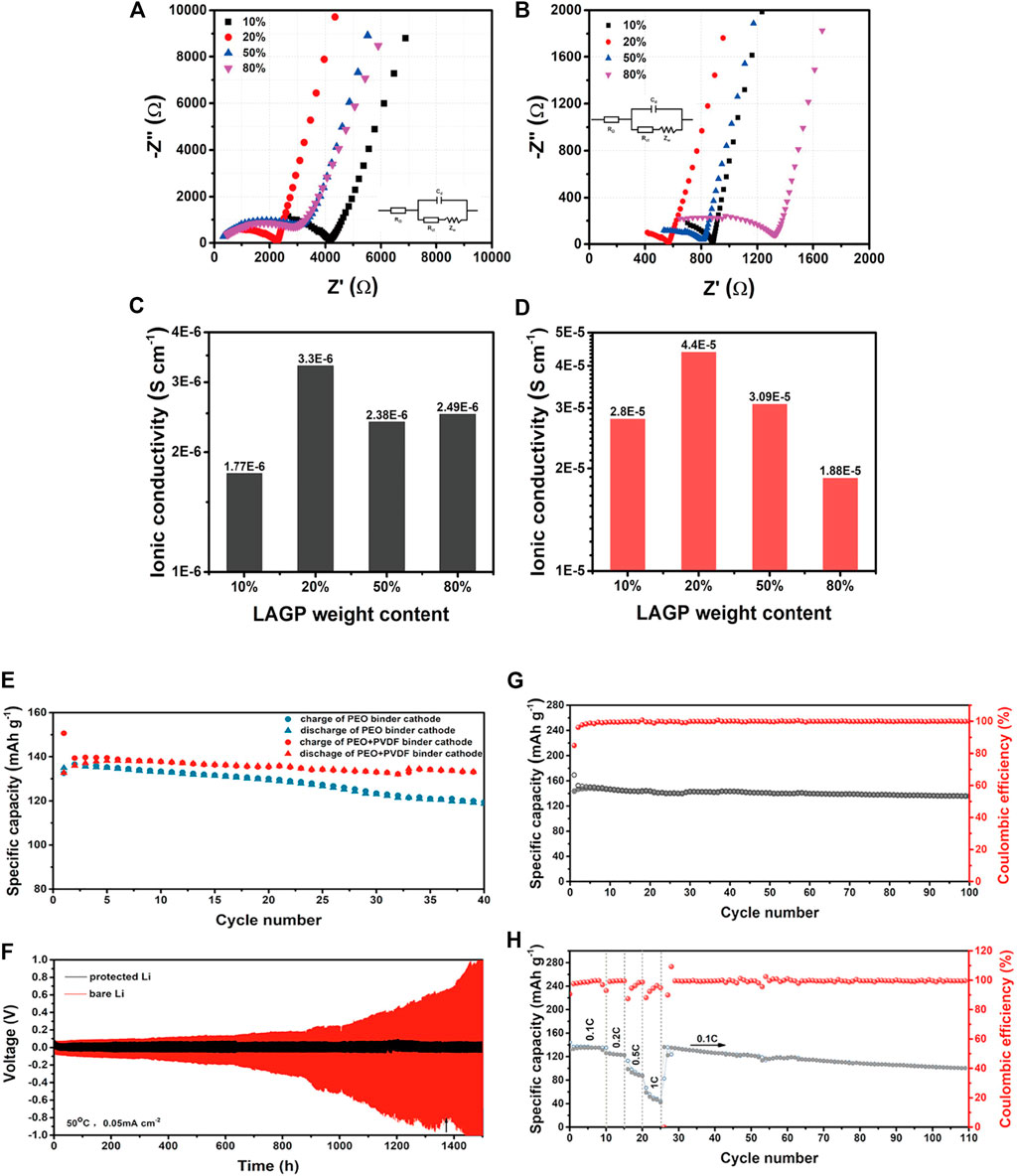
FIGURE 8. The impedance spectra of L@PCE-C (A) and L@PCE-CP (B) composite solid electrolytes with different LAGP contents, ionic conductivity of L@ PCE-C (C) and L@PCE-CP (D) at 25°C, (E) Cycling performances of Li/L@PCE-CP/LFP at 0.1 and 50°C using PL and PPL as cathode binder; (F) voltage-time profiles of symmetric cell with and without Li protective layer at 0.05 mA cm−2 and 50°C, (G) Cycle performance and (H) rate performance of LFP/L@PCE-CP/Li ASSBs at 0.1 and 50°C. Reproduced from ref. 72 with permission from Solid State ionics, copyright 2019.
The ceramic nanoparticles arranged vertically in the polymer matrix represent a solid structure that maximizes the ion conductivity of the composite electrolyte. Wang et al. (Wang et al., 2019a) uses the ice template method to construct a rechargeable solid-state lithium metal battery, which has a vertical arrangement composed of high ionic conductivity Li1.5Al0.5Ge1.5(PO4)3 (LAGP) and PEO (PEO) polymers ceramic/polymer composite electrolyte. The vertical LAGP wall provides a continuous channel for rapid ion migration, and the PEO matrix makes the composite electrolyte fluid. The conductivity of this solid composite electrolyte at room temperature is 1.67 × 10–4 S/cm, and the conductivity at 60°C is 1.11 × 10–3 S/cm. A capacity retention rate of the lithium iron phosphate (LFP)/vertically arranged LAGP-PEO/lithium full battery after 300 cycles is 93.3%. Wang et al. (Zhai et al., 2017) successfully prepared polycarbonate/PEO/LAGP composite PSSE. The system characterizes the influence of the weight ratio of PEO/polycarbonate, the molecular weight of PEO and the weight percentage of LAGP on the conductivity. The results show that the composite solid phase extraction of PPC-PEO10W [5:5]-1% by weight LAGP exhibits a maximum conductivity of 8.79 × 10–5 S/cm at 30°C, and a maximum conductivity of 8.39 × 10–4 S/cm at 60°C. Adding 1% by weight of LAGP to the polymer matrix not only improves the conductivity, but also broadens the electrochemical stability window. In this type of composite electrolyte, PEO-LAGP is a more active electrolyte.
Studies have shown that the form of ceramic additives also has certain effects on the mixing system (Liu et al., 2019). The nanometric ceramic particle filler can effectively improve ionic conductivity and stability. Liu et al. (La Monaca et al., 2019) used Li1.4Al0.4Ti1.6 (PO4) 3 (LATP) electrolyte nanoparticles as active fillers to improve the performance of electrolyte based on polyethylene oxide (PEO). Studies have shown that when the LATP mass fraction is 1%, the ionic conductivity of the composite electrolyte is increased to 1.2 × 10–5 S/cm at room temperature because high conductive LATP nanofiller is effectively suppressed by PEO matrix crystallization. The LATP nanofiller also enhances its electrochemical properties, mechanical and thermal stability. Zhai et al. (Wang et al., 2019b) reported on Li1+XALxTi2-x(PO4)3(LATP) nanoparticles vertically arranged and connected in a PEO (PEO) matrix to maximize ion conduction while maintaining the fluidity of the composite. This vertically arranged structure can be manufactured by an ice template-based method, and its electrical conductivity reaches 0.52 × 10–4 S/cm, which is 3.6 times that of a composite electrolyte with randomly dispersed LATP nanoparticles. Compared with pure PEO electrolyte, composite electrolyte also shows enhanced thermal stability and electrochemical stability.
Sulfide SSEs Active Filler
Oxide-based inorganic SSEs have good electrochemical stability, but generally have relatively low ion conductivity at room temperature. Compared with oxide SSEs, sulfide SSEs have high ionic conductivity, low grain boundary resistance and high oxidation potential. The ionic conductivity can reach 10–3 S/cm or even 10–2 S/cm, which is comparable to the ionic conductivity of liquid electrolytes. At the same time, inorganic SSEs generally have poor air stability, especially sulfide electrolytes, which can only be completed under vacuum conditions during preparation. This leads to a complicated preparation process, high cost, and poor toughness of the inorganic SSE, resulting in poor mechanical properties. Therefore, in recent years, in order to improve its various properties, scientists have made improvements in materials preparation technology, sintering technology and component doping.
Sulfide SSEs are classified into crystalline SSEs, glass SSEs and glass ceramic SSEs according to their crystalline form. In 1999, Kanno et al. (Kanno et al., 2000) proposed to substitute sulfur for oxygen in LISICON to obtain a thio-LISICON structure crystalline SSE. Later, Kamaya et al. (Kamaya et al., 2011) reported a sulfide crystalline electrolyte Li10GeP2S12 (LGPS) with a three-dimensional lithium-ion diffusion channel, and its room temperature conductivity reached 1.2 × 10−2 S/cm. Subsequently, Kato et al. (Kato et al., 2016) developed a new type of sulfide crystalline electrolyte Li9.54Si1.74P1.44S11.7Cl0.3, which has an ion conductivity of 2.5 × 10−2 S/cm at 27°C, which is twice that of LGPS. The sulfide glass SSEs include Li2S–SiS2 and Li2S–P2S5, and their intrinsic conductivity is only 10−8–10−6 S/cm. The ionic conductivity of the glass ceramic SSE obtained by high temperature crystallization treatment can reach 10–4–10−2 S/cm. Hayashi et al. (Hayashi et al., 2003) found that after high-temperature crystallization treatment, part of the Li2S–P2S5 glass phase crystallizes to form glass ceramics. The two-phase structure makes the electrolyte conductivity significantly improved. The intermediate phase thio-LISICON II analogue obtained by mechanical ball milling and high temperature crystallization improves the ionic conductivity of the electrolyte 80Li2S-20P2S5 from 1.7 × 10–4 to 7.2 × 10–4 S/cm. The 70Li2S-30P2S5 electrolyte with lower lithium content has a room temperature ion conductivity of 3.2 × 10–3 S/cm, which is nearly 2 orders of magnitude higher than that. In addition, lithium-ion conductivity can also be improved by doping (Chen et al., 2018b). When halides (such as LiI, LiBr, etc.) are incorporated into 70Li2S-30P2S5 glass electrolyte, the room temperature ion conductivity can reach 10–3 S/cm.
Synthetic sulfide/polymer composite electrolyte is an effective method to reduce the thickness of the electrolyte layer and maintain high ionic conductivity. In recent years, great progress has been made in reducing the thickness of the electrolyte layer, but ground distribution of nano-scale polymer binders in the sulfide particles of the composite electrolyte remains a challenge. The large polymer particles in the composite electrolyte hinder the transmission of ions and reduce the ion conductivity of the composite electrolyte membrane, resulting in poor performance of the composite electrolyte membrane. The research of Zhao et al. (Zhao et al., 2016b) found that the addition of filler LGPS (Li10GeP2S12) can effectively reduce the crystallinity of PEO/LiTFSI PSSE, thereby achieving the effect of reducing the impedance value and improving the ionic conductivity, and its interface impedance value to metal lithium decreases. The ionic conductivity reached 1.18 × 10–5 S cm−1 after adding 1% LGPS, and 10% SN was added continuously, the ionic conductivity reached 9.10 × 10–5 S cm−1 at room temperature.
Zhang et al. (Zhang et al., 2020b) synthesized a free-standing sulfide/polymer composite SSEs membrane with a thickness of 120 μm by a liquid phase method. The specific research results are shown in Figure 9. After adding lithium salt, the ionic conductivity of the composite electrolyte increased to 4.7 × 10–4 S/cm. The conductivity is 2.7 times that of 78Li2S-22P2S5 glass ceramic (7822gc) electrolyte particles. All solid-state lithium-sulfur batteries (ASSLIBs) with sulfide/polymer composite electrolyte membrane showed a high discharge capacity of 725.1 mA h g−1 and a capacity retention rate of 93.2% after 100 cycles of 0.176 mA cm−2.
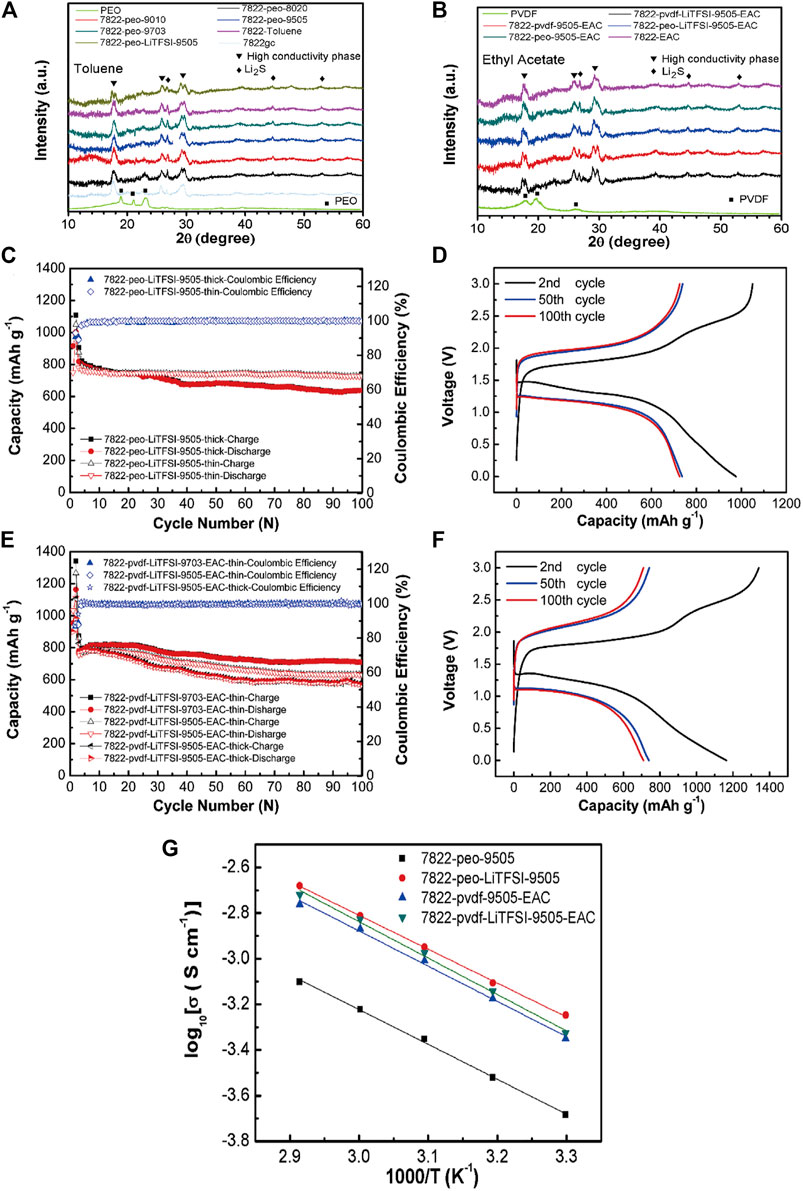
FIGURE 9. XRD patterns of 7822gc, composite electrolytes, and polymer electrolytes prepared with (A) toluene or (B) ethyl acetate as solvent, Cycle performance of (C) S-CNT||7822-peo-LiTFSI-9505||Li–In and (D) S-CNT||7822-pvdf-LiTFSI-9505(9703)-EAC||Li–In all-solid-state cells, The charge-discharge voltage profifiles at 2nd, 50th, and 100th cycles of the cells using 20 mg 7822-peo-LiTFSI-9505 and 7822-pvdf-LiTFSI-9703-EAC as the electrolyte layers are shown in (E,F,G) Arrhenius plots of ionic conductivity of the composite electrolytes. Reproduced from ref.80 with permission from. Energy Storage Materials, copyright 2020.
Conclusion
In summary, in the past few decades, many researches have been done on PEO-based CSSEs. The performance of the electrolyte can be improved through two ways of modifying the structure of the polymer and introducing filler doping. Through the modification of PEO polymer, it usually shows higher ionic conductivity, superior thermal and electrochemical stability and better flexibility than pure PSSEs. Blending/copolymerization between polymers can change the polymer structure, reduce the crystallinity or Tg of the polymer system, improve electrochemical stability, enhance mechanical strength, and inhibit the formation of lithium dendrites. The incorporation of inorganic fillers into the PSSE not only improves the ion conductivity, but also enhances the mechanical strength and stability and stability of the solid PSSE. In this review, we summarized the research progress in the structural modification of PEO PSSEs in recent years and the research content of introducing various types of fillers to form composite SSEs. And their comprehensive performance was evaluated.
Author Contributions
DZ: Writing-Original draft preparation, Investigation, Formal analysis, Data Curation, Resources. Lina Zhang: Data Curation, Visualization Investigation. XW: Supervision, Writing—Review & Editing, Funding acquisition. JW: Writing—Review & Editing, Funding acquisition. QL: Validation, Funding acquisition. KP: Software, Formal analysis. JH: Project administration, Funding acquisition.
Conflict of Interest
The authors declare that the research was conducted in the absence of any commercial or financial relationships that could be construed as a potential conflict of interest.
Publisher’s Note
All claims expressed in this article are solely those of the authors and do not necessarily represent those of their affiliated organizations, or those of the publisher, the editors and the reviewers. Any product that may be evaluated in this article, or claim that may be made by its manufacturer, is not guaranteed or endorsed by the publisher.
References
Agrawal, R. C., and Pandey, G. P. (2008). Solid Polymer Electrolytes: Materials Designing and All-Solid-State Battery Applications: An Overview. J. Phys. D: Appl. Phys. 41, 223001. doi:10.1088/0022-3727/41/22/223001
Al-Salih, H., Huang, A., Yim, C-H., Freytag, I. A., Goward, G. R., Baranova, E., et al. (2020). A Polymer-Rich Quaternary Composite Solid Electrolyte for Lithium Batteries. J. Electrochem. Soc. 167. doi:10.1149/1945-7111/ab7fb8
Armand, M. (1983). Polymer Solid Electrolytes - an Overview. Solid State Ionics 9-10, 745–754. doi:10.1016/0167-2738(83)90083-8
Baek, S.-W., Honma, I., Kim, J., and Rangappa, D. (2017). Solidified Inorganic-Organic Hybrid Electrolyte for All Solid State Flexible Lithium Battery. J. Power Sourc. 343, 22–29. doi:10.1016/j.jpowsour.2017.01.030
Bao, J., Shi, G., Tao, C., Wang, C., Zhu, C., Cheng, L., et al. (2018). Polycarbonate-based Polyurethane as a Polymer Electrolyte Matrix for All-Solid-State Lithium Batteries. J. Power Sourc. 389, 84–92. doi:10.1016/j.jpowsour.2018.04.020
Bates, J., Dudney, N. J., Gruzalski, G. R., Zuhr, R. A., Choudhury, A., Luck, C. F., et al. (1992). Electrical Properties of Amorphous Lithium Electrolyte Thin Films. Solid State Ionics 53-56, 647–654. doi:10.1016/0167-2738(92)90442-r
Cai, D., Wu, X., Xiang, J., Li, M., Su, H., Qi, X., et al. (2021). Ionic-liquid-containing Polymer Interlayer Modified PEO-Based Electrolyte for Stable High-Voltage Solid-State Lithium Metal Battery. Chem. Eng. J. 424, 130522. doi:10.1016/j.cej.2021.130522
Chandrasekhar, V. (1998). Polymer Solid Electrolytes: Synthesis and Structure. Adv. Polym. Sci. 135, 139–205.
Chen, L., Li, Y., Li, S.-P., Fan, L.-Z., Nan, C.-W., and Goodenough, J. B. (2018). PEO/garnet Composite Electrolytes for Solid-State Lithium Batteries: From "Ceramic-In-Polymer" to "Polymer-In-Ceramic". Nano Energy 46, 176–184. doi:10.1016/j.nanoen.2017.12.037
Chen, S., Xie, D., Liu, G., Mwizerwa, J. P., Zhang, Q., Zhao, Y., et al. (2018). Sulfide Solid Electrolytes for All-Solid-State Lithium Batteries: Structure, Conductivity, Stability and Application. Energ. Storage Mater. 14, 58–74. doi:10.1016/j.ensm.2018.02.020
Chen, Y., Wen, K., Chen, T., Zhang, X., Armand, M., and Chen, S. (2020). Recent Progress in All-Solid-State Lithium Batteries: The Emerging Strategies for Advanced Electrolytes and Their Interfaces. Energ. Storage Mater. 31, 401–433. doi:10.1016/j.ensm.2020.05.019
Cheng, J., Hou, G., Sun, Q., Liang, Z., Xu, X., Guo, J., et al. (2020). Cold-pressing PEO/LAGP Composite Electrolyte for Integrated All-Solid-State Lithium Metal Battery. Solid State Ionics 345, 115156. doi:10.1016/j.ssi.2019.115156
Cheng, Z., Liu, T., Zhao, B., Shen, F., Jin, H., and Han, X. (2021). Recent Advances in Organic-Inorganic Composite Solid Electrolytes for All-Solid-State Lithium Batteries. Energ. Storage Mater. 34, 388–416. doi:10.1016/j.ensm.2020.09.016
Chen-Yang, Y. W., Wang, Y. L., Chen, Y. T., Li, Y. K., Chen, H. C., and Chiu, H. Y. (2008). Influence of Silica Aerogel on the Properties of Polyethylene Oxide-Based Nanocomposite Polymer Electrolytes for Lithium Battery. J. Power Sourc. 182, 340–348. doi:10.1016/j.jpowsour.2008.04.001
Choi, J.-H., Lee, C.-H., Yu, J.-H., Doh, C.-H., and Lee, S.-M. (2015). Enhancement of Ionic Conductivity of Composite Membranes for All-Solid-State Lithium Rechargeable Batteries Incorporating Tetragonal Li7La3Zr2O12 into a Polyethylene Oxide Matrix. J. Power Sourc. 274, 458–463. doi:10.1016/j.jpowsour.2014.10.078
Commarieu, B., Paolella, A., Daigle, J.-C., and Zaghib, K. (2018). Toward High Lithium Conduction in Solid Polymer and Polymer-Ceramic Batteries. Curr. Opin. Electrochemistry 9, 56–63. doi:10.1016/j.coelec.2018.03.033
Croce, F., Appetecchi, G. B., Persi, L., and Scrosati, B. (1998). Nanocomposite Polymer Electrolytes for Lithium Batteries. Nature 394, 456–458. doi:10.1038/28818
Das, S., and Ghosh, A. (2015). Ion Conduction and Relaxation in PEO-LiTFSI-Al2O3 Polymer Nanocomposite Electrolytes. J. Appl. Phys. 117, 174103. doi:10.1063/1.4919721
Ding, B., Wang, J., Fan, Z., Chen, S., Lin, Q., Lu, X., et al. (2020). Solid-state Lithium-Sulfur Batteries: Advances, Challenges and Perspectives. Mater. Today 40, 114–131. doi:10.1016/j.mattod.2020.05.020
Feng, J., Wang, L., Chen, Y., Wang, P., Zhang, H., and He, X. (2021). PEO Based Polymer-Ceramic Hybrid Solid Electrolytes: a Review. Nano Convergence 8, 2. doi:10.1186/s40580-020-00252-5
Fenton, D. E., Parker, J. M., and Wright, P. V. (1973). Complexes of Alkali Metal Ions with Poly(ethylene Oxide). Polymer 14, 589. doi:10.1016/0032-3861(73)90146-8
Fu, K., Gong, Y., Hitz, G. T., McOwen, D. W., Li, Y., Xu, S., et al. (2017). Three-dimensional Bilayer Garnet Solid Electrolyte Based High Energy Density Lithium Metal-Sulfur Batteries. Energy Environ. Sci. 10, 1568–1575. doi:10.1039/c7ee01004d
Goodenough, J. B., and Park, K.-S. (2013). The Li-Ion Rechargeable Battery: A Perspective. J. Am. Chem. Soc. 135, 1167–1176. doi:10.1021/ja3091438
Goodenough, J. B., Hong, H. Y.-P., and Kafalas, J. A. (1976). Fast Na+-Ion Transport in Skeleton Structures. Mater. Res. Bull. 11 (2), 203–220. doi:10.1016/0025-5408(76)90077-5
Hayashi, A., Hama, S., Minami, T., and Tatsumisago, M. (2003). Formation of Superionic Crystals from Mechanically Milled Li2S-P2s5 Glasses. Electrochemistry Commun. 5, 111–114. doi:10.1016/s1388-2481(02)00555-6
He, W., Cui, Z., Liu, X., Cui, Y., Chai, J., Zhou, X., et al. (2017). Carbonate-linked Poly(ethylene Oxide) Polymer Electrolytes towards High Performance Solid State Lithium Batteries. Electrochimica Acta 225, 151–159. doi:10.1016/j.electacta.2016.12.113
He, K., Chen, C., Fan, R., Liu, C., Liao, C., Xu, Y., et al. (2019). Polyethylene Oxide/garnet-type Li6.4La3Zr1.4Nb0.6O12 Composite Electrolytes with Improved Electrochemical Performance for Solid State Lithium Rechargeable Batteries. Composites Sci. Technol. 175, 28–34. doi:10.1016/j.compscitech.2019.02.030
Huang, J., Liang, F., Hou, M., Zhang, Y., Chen, K., and Xue, D. (2020). Garnet-type Solid-State Electrolytes and Interfaces in All-Solid-State Lithium Batteries: Progress and Perspective. Appl. Mater. Today 20, 100750. doi:10.1016/j.apmt.2020.100750
Kamaya, N., Homma, K., Yamakawa, Y., Hirayama, M., Kanno, R., Yonemura, M., et al. (2011). A Lithium Superionic Conductor. Nat. Mater 10, 682–686. doi:10.1038/nmat3066
Kanno, R., Hata, T., and Kawamoto, Y. (2000). Synthesis of a New Lithium Ionic Conductor, Thio-LISICON-Lithium Germanium Sulfide System. Solid State Ionics 130 (1), 97–104. doi:10.1016/s0167-2738(00)00277-0
Kato, Y., Hori, S., Saito, T., Suzuki, K., Hirayama, M., Mitsui, A., et al. (2016). High-power All-Solid-State Batteries Using Sulfide Superionic Conductors. Nat. Energ. 1, 16030. doi:10.1038/nenergy.2016.30
Khurana, R., Schaefer, J. L., Archer, L. A., and Coates, G. W. (2014). Suppression of Lithium Dendrite Growth Using Cross-Linked Polyethylene/poly(ethylene Oxide) Electrolytes: a New Approach for Practical Lithium-Metal Polymer Batteries. J. Am. Chem. Soc. 136, 7395–7402. doi:10.1021/ja502133j
Kotobuki, M., and Koishi, M. (2013). Preparation of Li1.5Al0.5Ti1.5(PO4)3 Solid Electrolyte via a Sol-Gel Route Using Various Al Sources. Ceramics Int. 39, 4645–4649. doi:10.1016/j.ceramint.2012.10.206
Kotobuki, M., Koishi, M., and Kato, Y. (2013). Preparation of Li1.5Al0.5Ti1.5(PO4)3 Solid Electrolyte via a Co-precipitation Method. Ionics 19, 1945–1948. doi:10.1007/s11581-013-1000-4
La Monaca, A., Paolella, A., Guerfi, A., Rosei, F., and Zaghib, K. (2019). Electrospun Ceramic Nanofibers as 1D Solid Electrolytes for Lithium Batteries. Electrochemistry Commun. 104, 106483. doi:10.1016/j.elecom.2019.106483
Li, L., Deng, Y., and Chen, G. (2020). Status and prospect of Garnet/polymer Solid Composite Electrolytes for All-Solid-State Lithium Batteries. J. Energ. Chem. 50, 154–177. doi:10.1016/j.jechem.2020.03.017
Lightfoot, P., Mehta, M. A., and Bruce, P. G. (1993). Crystal Structure of the Polymer Electrolyte Poly(ethylene oxide)3:LiCF3SO3. Science 262 (5135), 883–885. doi:10.1126/science.262.5135.883
Lin, Y.-Y., Yong, A. X. B., Gustafson, W. J., Reedy, C. N., Ertekin, E., Krogstad, J. A., et al. (2020). Toward Design of Cation Transport in Solid-State Battery Electrolytes: Structure-Dynamics Relationships. Curr. Opin. Solid State. Mater. Sci. 24, 100875. doi:10.1016/j.cossms.2020.100875
Liu, L., Chu, L., Jiang, B., and Li, M. (2019). Li1.4Al0.4Ti1.6(PO4)3 Nanoparticle-Reinforced Solid Polymer Electrolytes for All-Solid-State Lithium Batteries. Solid State Ionics 331, 89–95. doi:10.1016/j.ssi.2019.01.007
Long, L., Wang, S., Xiao, M., and Meng, Y. (2016). Polymer Electrolytes for Lithium Polymer Batteries. J. Mater. Chem. A. 4, 10038–10069. doi:10.1039/c6ta02621d
Ma, F., Zhang, Z., Yan, W., Ma, X., Sun, D., Jin, Y., et al. (2019). Solid Polymer Electrolyte Based on Polymerized Ionic Liquid for High Performance All-Solid-State Lithium-Ion Batteries. ACS Sustain. Chem. Eng. 7, 4675–4683. doi:10.1021/acssuschemeng.8b04076
Masoud, E. M., El-Bellihi, A.-A., Bayoumy, W. A., and Mousa, M. A. (2013). Effect of LiAlO2 Nanoparticle Filler Concentration on the Electrical Properties of PEO-LiClO4 Composite. Mater. Res. Bull. 48, 1148–1154. doi:10.1016/j.materresbull.2012.12.012
Mauger, A., Julien, C. M., Paolella, A., Armand, M., and Zaghib, K. (2019). Building Better Batteries in the Solid State: A Review. Materials (Basel) 12, 3892. doi:10.3390/ma12233892
Murugan, R., Thangadurai, V., and Weppner, W. (2007). Fast Lithium Ion Conduction in Garnet-type Li7La3Zr2O12. Angew. Chem. Int. Ed. 46 (41), 7778–7781. doi:10.1002/anie.200701144
Park, S. S., Tulchinsky, Y., and Dincă, M. (2017). Single-Ion Li+, Na+, and Mg2+ Solid Electrolytes Supported by a Mesoporous Anionic Cu-Azolate Metal-Organic Framework. J. Am. Chem. Soc. 139, 13260–13263. doi:10.1021/jacs.7b06197
Rocco, A. M., da Fonseca, C. P., and Pereira, R. P. (2002). A Polymeric Solid Electrolyte Based on a Binary Blend of Poly(ethylene Oxide), Poly(methyl Vinyl Ether-Maleic Acid) and LiClO 4. Polymer 43, 3601–3609. doi:10.1016/s0032-3861(02)00173-8
Shi, J., Yang, Y., and Shao, H. (2018). Co-polymerization and Blending Based PEO/PMMA/P(VDF-HFP) Gel Polymer Electrolyte for Rechargeable Lithium Metal Batteries. J. Membr. Sci. 547, 1–10. doi:10.1016/j.memsci.2017.10.033
Shriver, D. F., Papke, B. L., Ratner, M. A., Dupon, R., Wong, T., and Brodwin, M. (1981). Structure and Ion Transport in Polymer-Salt Complexes. Solid State Ionics 5, 83–88. doi:10.1016/0167-2738(81)90199-5
Singh, K. P., and Gupta, P. N. (1998). Study of Dielectric Relaxation in Polymer Electrolytes. Eur. Polym. J. 34 (7), 1023–1029. doi:10.1016/s0014-3057(97)00207-3
Subramanian, K., Alexander, G. V., Karthik, K., Patra, S., Indu, M. S., Sreejith, O. V., et al. (2021). A Brief Review of Recent Advances in Garnet Structured Solid Electrolyte Based Lithium Metal Batteries. J. Energ. Storage 33, 102157. doi:10.1016/j.est.2020.102157
Sun, Y., Liu, N., and Cui, Y. (2016). Promises and Challenges of Nanomaterials for Lithium-Based Rechargeable Batteries. Nat. Energ. 1, 16071. doi:10.1038/nenergy.2016.71
Sun, C., Liu, J., Gong, Y., Wilkinson, D. P., and Zhang, J. (2017). Recent Advances in All-Solid-State Rechargeable Lithium Batteries. Nano Energy 33, 363–386. doi:10.1016/j.nanoen.2017.01.028
Tan, S.-J., Zeng, X.-X., Ma, Q., Wu, X.-W., and Guo, Y.-G. (2018). Recent Advancements in Polymer-Based Composite Electrolytes for Rechargeable Lithium Batteries. Electrochem. Energ. Rev. 1, 113–138. doi:10.1007/s41918-018-0011-2
Thokchom, J. S., and Kumar, B. (2008). Composite Effect in Superionically Conducting Lithium Aluminium Germanium Phosphate Based Glass-Ceramic. J. Power Sourc. 185, 480–485. doi:10.1016/j.jpowsour.2008.07.009
Van der Ven, A., Bhattacharya, J., and Belak, A. A (2013). Understanding Li Diffusion in Li-Intercalation Compounds. Acc. Chem. Res 46 (5), 1216–1225. doi:10.1021/ar200329r
Walter, R., Walkenhorst, R., Smith, M., Selser, J. C., Piet, G., and Bogoslovov, R. (2000). The Role of Polymer Melt Viscoelastic Network Behavior in Lithium Ion Transport for PEO melt/LiClO4 SPEs: the "wet Gel" Model. J. Power Sourc. 89, 168–175. doi:10.1016/s0378-7753(00)00426-2
Wan, J., Xie, J., Mackanic, D. G., Burke, W., Bao, Z., and Cui, Y. (2018). Status, Promises, and Challenges of Nanocomposite Solid-State Electrolytes for Safe and High Performance Lithium Batteries. Mater. Today Nano 4, 1–16. doi:10.1016/j.mtnano.2018.12.003
Wang, X.-L., Mei, A., Li, M., Lin, Y.-H., and Nan, C.-W. (2007). Polymer Composite Electrolytes Containing Ionically Active Mesoporous SiO2 Particles. J. Appl. Phys. 102, 054907. doi:10.1063/1.2776251
Wang, Z., Wang, Z., Yang, L., Wang, H., Song, Y., Han, L., et al. (2018). Boosting Interfacial Li+ Transport with a MOF-Based Ionic Conductor for Solid-State Batteries. Nano Energy 49, 580–587. doi:10.1016/j.nanoen.2018.04.076
Wang, X., Zhai, H., Qie, B., Cheng, Q., Li, A., Borovilas, J., et al. (2019). Rechargeable Solid-State Lithium Metal Batteries with Vertically Aligned Ceramic Nanoparticle/polymer Composite Electrolyte. Nano Energy 60, 205–212. doi:10.1016/j.nanoen.2019.03.051
Wang, Z., Gu, H., Wei, Z., Wang, J., Yao, X., and Chen, S. (2019). Preparation of New Composite Polymer Electrolyte for Long Cycling All-Solid-State Lithium Battery. Ionics 25, 907–916. doi:10.1007/s11581-019-02852-6
Wei, X., and Shriver, D. F. (1998). Highly Conductive Polymer Electrolytes Containing Rigid Polymers. Chem. Mater. 10 (9), 2307–2308. doi:10.1021/cm980170z
Wright, P. V. (1975). Electrical Conductivity in Ionic Complexes of Poly(ethylene Oxide). Br. Poly. J. 7, 319–327. doi:10.1002/pi.4980070505
Wu, M.-S., and Chiang, P.-C. J. (2007). High-rate Capability of Lithium-Ion Batteries after Storing at Elevated Temperature. Electrochimica Acta 52, 3719–3725. doi:10.1016/j.electacta.2006.10.045
Wu, N., Chien, P. H., Qian, Y., Li, Y., Xu, H., Grundish, N. S., et al. (2020). Enhanced Surface Interactions Enable Fast Li + Conduction in Oxide/Polymer Composite Electrolyte. Angew. Chem. Int. Ed. 59, 4131–4137. doi:10.1002/anie.201914478
Xu, L., Wei, K., Cao, Y., Ma, S., Li, J., Zhao, Y., et al. (2020). The Synergistic Effect of the PEO-PVA-PESf Composite Polymer Electrolyte for All-Solid-State Lithium-Ion Batteries. RSC Adv. 10, 5462–5467. doi:10.1039/c9ra09645k
Yasuo, T., Nobuyuki, I., and Osamu, Y. (2009). Developments of the Advanced All Solid State Polymer Electrolyte Lithium Secondary Battery. Electrochemistry 77, 785–794. doi:10.5796/electrochemistry.77.784
Yu, X., and Manthiram, A. (2021). A Review of Composite Polymer-Ceramic Electrolytes for Lithium Batteries. Energ. Storage Mater. 34, 282–300. doi:10.1016/j.ensm.2020.10.006
Yu, C., Zhao, F., Luo, J., Zhang, L., and Sun, X. (2021). Recent Development of Lithium Argyrodite Solid-State Electrolytes for Solid-State Batteries: Synthesis, Structure, Stability and Dynamics. Nano Energy 83, 105858. doi:10.1016/j.nanoen.2021.105858
Yu, Q., Jiang, K., Yu, C., Chen, X., Zhang, C., Yao, Y., et al. (2021). Recent Progress of Composite Solid Polymer Electrolytes for All-Solid-State Lithium Metal Batteries. Chin. Chem. Lett. doi:10.1016/j.cclet.2021.03.032
Yue, L., Ma, J., Zhang, J., Zhao, J., Dong, S., Liu, Z., et al. (2016). All Solid-State Polymer Electrolytes for High-Performance Lithium Ion Batteries. Energ. Storage Mater. 5, 139–164. doi:10.1016/j.ensm.2016.07.003
Zhai, H., Xu, P., Ning, M., Cheng, Q., Mandal, J., and Yang, Y. (2017). A Flexible Solid Composite Electrolyte with Vertically Aligned and Connected Ion-Conducting Nanoparticles for Lithium Batteries. Nano Lett. 17, 3182–3187. doi:10.1021/acs.nanolett.7b00715
Zhang, J., Zhao, N., Zhang, M., Li, Y., Chu, P. K., Guo, X., et al. (2016). Flexible and Ion-Conducting Membrane Electrolytes for Solid-State Lithium Batteries: Dispersion of Garnet Nanoparticles in Insulating Polyethylene Oxide. Nano Energy 28, 447–454. doi:10.1016/j.nanoen.2016.09.002
Zhang, Q., Liu, K., Ding, F., and Liu, X. (2017). Recent Advances in Solid Polymer Electrolytes for Lithium Batteries. Nano Res. 10, 4139–4174. doi:10.1007/s12274-017-1763-4
Zhang, T., Wang, Y., Song, T., Miyaoka, H., Shinzato, K., Miyaoka, H., et al. (2018). Ammonia, a Switch for Controlling High Ionic Conductivity in Lithium Borohydride Ammoniates. Joule 2, 1522–1533. doi:10.1016/j.joule.2018.04.015
Zhang, T., He, W., Zhang, W., Wang, T., Li, P., Sun, Z., et al. (2020). Designing Composite Solid-State Electrolytes for High Performance Lithium Ion or Lithium Metal Batteries. Chem. Sci. 11, 8686–8707. doi:10.1039/d0sc03121f
Zhang, Y., Chen, R., Wang, S., Liu, T., Xu, B., Zhang, X., et al. (2020). Free-standing Sulfide/polymer Composite Solid Electrolyte Membranes with High Conductance for All-Solid-State Lithium Batteries. Energ. Storage Mater. 25, 145–153. doi:10.1016/j.ensm.2019.10.020
Zhang, T., Shao, Y., Zhang, X., Huang, Y., Wang, S., Zhou, W., et al. (2021). Fast Lithium Ionic Conductivity in Complex Hydride‐Sulfide Electrolytes by Double Anions Substitution. Small Methods 5, 2100609. doi:10.1002/smtd.202100609
Zhang, X., Zhang, T., Shao, Y., Cao, H., Liu, Z., Wang, S., et al. (2021). Composite Electrolytes Based on Poly(Ethylene Oxide) and Lithium Borohydrides for All-Solid-State Lithium-Sulfur Batteries. ACS Sustain. Chem. Eng. 9, 5396–5404. doi:10.1021/acssuschemeng.1c00381
Zhao, Y., Huang, Z., Chen, S., Chen, B., Yang, J., Zhang, Q., et al. (2016). A Promising PEO/LAGP Hybrid Electrolyte Prepared by a Simple Method for All-Solid-State Lithium Batteries. Solid State Ionics 295, 65–71. doi:10.1016/j.ssi.2016.07.013
Zhao, Y., Wu, C., Peng, G., Chen, X., Yao, X., Bai, Y., et al. (2016). A New Solid Polymer Electrolyte Incorporating Li10GeP2S12 into a Polyethylene Oxide Matrix for All-Solid-State Lithium Batteries. J. Power Sourc. 301, 47–53. doi:10.1016/j.jpowsour.2015.09.111
Zheng, F., Kotobuki, M., Song, S., Lai, M. O., and Lu, L. (2018). Review on Solid Electrolytes for All-Solid-State Lithium-Ion Batteries. J. Power Sourc. 389, 198–213. doi:10.1016/j.jpowsour.2018.04.022
Zhu, L., Zhu, P., Fang, Q., Jing, M., Shen, X., and Yang, L. (2018). A Novel Solid PEO/LLTO-nanowires Polymer Composite Electrolyte for Solid-State Lithium-Ion Battery. Electrochimica Acta 292, 718–726. doi:10.1016/j.electacta.2018.10.005
Keywords: oxide, sulfide, PEO, structure modification, composite solid electrolyte
Citation: Zhang D, Li L, Wu X, Wang J, Li Q, Pan K and He J (2021) Research Progress and Application of PEO-Based Solid State Polymer Composite Electrolytes. Front. Energy Res. 9:726738. doi: 10.3389/fenrg.2021.726738
Received: 17 June 2021; Accepted: 10 September 2021;
Published: 28 September 2021.
Edited by:
Yaser A. Abu-Lebdeh, National Research Council Canada (NRC-CNRC), CanadaReviewed by:
Andrea Paolella, Hydro-Québec, CanadaMohamed S. E. Houache, National Research Council Canada (NRC-CNRC), Canada
Hilal Al-Salih, University of Ottawa, Canada
Copyright © 2021 Zhang, Li, Wu, Wang, Li, Pan and He. This is an open-access article distributed under the terms of the Creative Commons Attribution License (CC BY). The use, distribution or reproduction in other forums is permitted, provided the original author(s) and the copyright owner(s) are credited and that the original publication in this journal is cited, in accordance with accepted academic practice. No use, distribution or reproduction is permitted which does not comply with these terms.
*Correspondence: Xiaochao Wu, d3V4aWFvY2hhb0B6enUuZWR1LmNu; Jun Wang, d2FuZ2pAenp1LmVkdS5jbg==