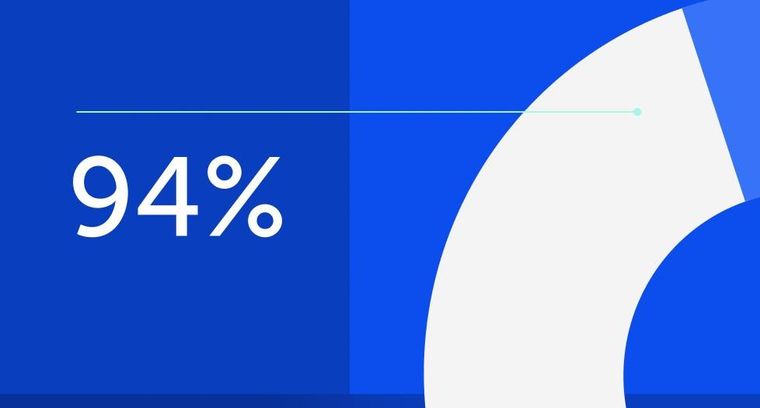
94% of researchers rate our articles as excellent or good
Learn more about the work of our research integrity team to safeguard the quality of each article we publish.
Find out more
ORIGINAL RESEARCH article
Front. Energy Res., 30 September 2021
Sec. Solar Energy
Volume 9 - 2021 | https://doi.org/10.3389/fenrg.2021.722514
The majority of incident solar irradiance causes thermalization in photovoltaic (PV) cells, attenuating their efficiency. In order to use solar energy on a large scale and reduce carbon emissions, their efficiency must be enhanced. Effective thermal management can be utilized to generate additional electrical power while simultaneously improving photovoltaic efficiency. In this work, an experimental model of a hybrid photovoltaic-thermoelectric generation (PV-TEG) system is developed. Ten bismuth telluride-based thermoelectric modules are attached to the rear side of a 10 W polycrystalline silicon-based photovoltaic module in order to recover and transform waste thermal energy to usable electrical energy, ultimately cooling the PV cells. The experiment was then carried out for 10 days in Lahore, Pakistan, on both a simple PV module and a hybrid PV-TEG system. The findings revealed that a hybrid system has boosted PV module output power and conversion efficiency. The operating temperature of the PV module in the hybrid system is reduced by 5.5%, from 55°C to 52°C. Due to a drop in temperature and the addition of some recovered energy by thermoelectric modules, the total output power and conversion efficiency of the system increased. The hybrid system’s cumulative output power increased by 19% from 8.78 to 10.84 W, compared to the simple PV system. Also, the efficiency of the hybrid PV-TEG system increased from 11.6 to 14%, which is an increase of 17% overall. The results of this research could provide consideration for designing commercial hybrid PV-TEG systems.
An unprecedented challenge in the 21st century is to fulfill the outgrowing energy demand of the world with eco-friendly, efficient, cost-effective, and sustainable energy resources. Being clean, sustainable, and the most abundant energy resource on the earth, solar energy draws a great deal of attention to address a plethora of energy issues. As a matter of fact, cumulative solar irradiance reaching the earth’s surface is greater than 7,500 times the total energy consumption per year which is 450 EJ worldwide (Thirugnanasambandam et al., 2010; Moh’d A et al., 2017).
Numerous studies on the conversion of solar energy into thermal, electrical, and chemical energies through photothermal, photovoltaic, and photochemical processes have been conducted in recent years. However, solar photovoltaic conversion is now widely used in a variety of applications. In recent years, PV cell technology has advanced at a rapid pace (Khan et al., 2016). Many researchers are investigating the thermodynamics of solar PV energy conversion utilizing the first and second laws of thermodynamics for performance analysis and efficiency enhancement (Joshi et al., 2014; Arabkoohsar et al., 2015; Esmaili et al., 2015). To investigate the influence of the band energy gap on the efficiency of solar PV cells, Baruch et al. (1995) used a thermodynamic approach. Rusirawan (2012) thermodynamically performed an exergetic analysis of a PV system utilizing the photon energy approach. Sundaram et al. (2015) conducted an exergy and energy efficiency analysis of a 5 MW PV power plant in south India. Bicer et al. (2016) evaluated the irreversibilities related to the photothermoelectric processes in solar PV systems using a comprehensive approach.
Currently, PV systems are used in various applications (directly or indirectly) such as power generation from household rooftop and building integrated scale to large grid station, refrigeration and air conditioning, solar cooking, solar drying, water heating and desalination, lightening systems for streetlights, and traffic signals (Ma et al., 2015). Solar power generation through PV systems has the highest power density among all renewable energy sources (Smil, 2005). Solar plants have a potential life of more than 25 years, but they can persist for up to 100 years (Lakatos et al., 2011). Solar photovoltaic is expected to account for around 16% of worldwide energy output by 2050 (Sathe et al., 2017). Santhi Rekha and Sukchai (2018) developed and tested a parabolic solar concentric cooker with and without phase change material (PCM). They verified that the cooking power of a solar concentric cooker with PCM is two times that of a solar concentric cooker without PCM. Salilih and Birhane (2019) proposed a method for analyzing a vapor compression refrigeration system under real-life situations using PV and compressor characteristics from the datasheets. Manokar et al. (2018) experimentally investigated the performance of PV module-integrated solar still that generates power and desalinates water simultaneously. In another paper, they investigated the influence of mass flow rate in an inclined solar panel combined with a spiral tube water heater experimentally (Manokar and Treatment, 2019). Kabeel et al. (2019) conducted an experiment on inclined PV panel solar still with PCM as well as cover cooling. The findings revealed that the efficiency and power of the inclined PV panel solar still depend upon panel surface, cover, and water temperature. Sasikumar et al. (2020) demonstrated experimentally that higher water flow conditions reduce the electrical, thermal, and energy efficiency of solar panels, as well as the rate of distilled water production.
For several decades, experts from academia and industry have concentrated and conducted extensive research on the PV systems to convert solar energy into electricity. However, the conversion efficiencies of PV modules available in the market have been recorded in the range of 10–15 percent as it utilizes the visible range of solar radiation only. The infrared range is transmitted and induces thermalization of PV cells which adversely affects its efficiency and output power (Dimri et al., 2017). Different techniques for cooling PV modules have therefore been suggested and among them, the fully fledged technologies are air and water cooling with practical implementation worldwide (Makki et al., 2015). In addition to these two, the potential solutions that have also been investigated are nanofluid, heat pipe, and thermoelectric cooling. Hence, the operating temperature of the PV modules can be maintained at an appropriate level to ensure adequate efficiency and power output. The conversion efficiency of PV has been reported to decrease by a range of 0.25–0.5 percent per degree rise in its operating temperature depending on the material used (Grubišić-Čabo et al., 2016). It demonstrates that cooling for photovoltaics is important as its performance will be favored by a slight decrease in operating temperature. Therefore, effective recovery of waste heat from a PV module will not only improve its efficiency but also provide additional useful energy simultaneously.
Thermoelectricity (TE) is essentially a bidirectional energy transformation technology for the conversion of thermal energy into electricity, and vice versa. This technique offers an alternative to conventional processes used for heating, cooling, and power generation from waste heat. The primary benefits of adopting these thermoelectric (TE) devices include gas-free emission, noiseless operation, significant scalability, vibration and maintenance-free operation without moving components, and long-term operational reliability (He et al., 2015; Li et al., 2018). However, the widespread implementation of thermoelectric generators (TEGs) has been limited by low conversion efficiency. The conversion efficiency of these devices depends upon two parameters, geometry and material, and a lot of researches have been conducted to optimize these parameters, eventually improving their efficiency (Li et al., 2017; Shkatulov et al., 2019).
Thermoelectric devices have also been investigated recently for cooling of PV systems by transforming PV cell’s surplus heat into electricity (Deng et al., 2013; Zhu et al., 2016). Cooling photovoltaics using thermoelectric increases conversion efficiency and power production of PV systems, implying the feasibility of constructing a realistic hybrid photovoltaic-thermoelectric generation (PV-TEG) system (Li et al., 2014). Wang et al. (2011) experimentally examined the performance of a dye-synthesized solar cell (DSSC) and a thermoelectric module and discovered that using a TE module improved system efficiency from 9.39 to 13.8%. Lin et al. (2015) derived the mathematical model of hybrid PV-TEG system and proposed its optimal design for practical implementation. They analyzed the effect of TEG structural parameters and PV module operating conditions on hybrid PV-TEG performance. Zhang et al. (2014) estimated the efficiency of hybrid concentrated photovoltaics with thermoelectric generators (CPV-TEG) theoretically by using different PV modules. Kossyvakis et al. (2016) experimentally investigated the output of tandem PV-TEG system of multicrystalline silicon photovoltaic module. They claimed that TE modules with tiny legs boost the power performance of the hybrid system. Sark (2011) claimed from PV-TE hybrid system’s model that the efficiency of this system can be increased by 8–23 percent. Dallan et al. (2015) experimentally compared the PV system alone; the output of the hybrid PV-TEG system increases by integrating TEG as a heat pump in this system. Rejeb et al. (2020) presented a statistical model to investigate the effect of various parameters on the conversion efficiency of a CPV-TEG system, including solar irradiance, optical concentration, thermoelectric leg height, load resistance, and ambient temperature. Novel multicrystalline photovoltaics with the incorporation of bismuth telluride modules on its rear side was proposed by Babu and Ponnambalam (2018). The suggested design results in 5 percent increased electricity production with 6 percent improvement in conversion efficiency under standard test conditions (STC). Cotfas et al. (2016) conducted a simulation study of flat plate PV-TEG system in LABVIEW and reported a power gain of 7 percent with an overall efficiency of almost 19 percent. Liu et al. (2020a) investigated how different glass coatings affected the performance of hybrid PV-TEG with light and heat management. According to the findings, the selective coating can increase the system’s power generation by approximately 14%. In MATLAB/Simulink, Verma et al. (2016) presented simulation models of the PV-TEG system with two independent maximum power point tracking (MPPT) controllers to extract the maximum power. Shittu et al. (2020) examined exergy analysis of a hybrid PV-TEG system with a microchannel heat pipe. The results demonstrate that the hybrid system outperforms the simple system, and the absence of insulation behind the microchannel heat pipe improves the hybrid system’s electrical performance. Liu et al. (2020b) designed a water-cooling-assisted PV-TEG system and reported the temperature and conversion efficiency of the system at a steady state. Findings revealed that, at 1000 W/m2, the steady-state temperature of the PV cell drops from 86.8°C to 54.1°C, while the overall efficiency rises from 15.6 to 21.1 percent.
This is evident from the literature that the efficiency of the PV module increases with the integration of TEG on its backside. However, all these studies assume a steady temperature gradient through TEG which is not valid in case of solar input. Therefore, in this work, the performance of the hybrid PV-TEG system is evaluated at transient hot side temperature of the TE module.
Most of the previous researches have concentrated on describing two individual technologies (PV and TEG) separately and integration of TE modules with monocrystalline PV modules especially. Furthermore, researchers have employed the PV-TEG integration with other auxiliaries such as water or other types of fluid circulation to cool the PV-TEG system, spectrum splitting mechanism, and lubricants between PV and TEG. These auxiliaries add additional cost to the PV-TEG system and require energy as well as proper maintenance for their operation. This study investigates the integration of a silicon-based polycrystalline PV panel with bismuth telluride-based TEG without the usage of any additional component as a way to improve the PV module’s output power and efficiency. An experimental model of a hybrid PV-TEG system is developed in which 10 bismuth telluride-based thermoelectric modules are attached to the rear side of a 10 W polycrystalline silicon-based photovoltaic module in order to recover and transform waste thermal energy to usable electrical energy. The results for both simple and hybrid systems are presented, and it is clear that a hybrid system has boosted output power and efficiency.
The photovoltaic effect is the generation of electrical voltage across a material when it is exposed to light. A photovoltaic cell is a junction between two semiconductors, p-type and n-type, when photons strike on the junction, electron-hole pairs are generated. The induced electric field diffuses and separates these electron-hole pairs at the junction; these separated charges travel in opposite directions, eventually flowing in the external circuit, hence producing electrical current as shown in Figure 1 (Huen et al., 2017).
The equivalent electrical circuit used to model a PV module is shown in Figure 2. Kirchhoff’s Current Law helps to create characteristic models of PV module. Thus, an ideal PV cell series model can be expressed mathematically as follows:
where Ipv is the output photovoltaic current of PV module, Iph is the current generated by incoming photons given in Eq. 3, and Is is the saturation current of diode.
In an actual PV module, one diode and two resistances, one in series and the other in parallel, are used as shown in Figure 3 (Belkassmi et al., 2017).
where Irs is the current through added resistance and incoming photons produce current Iph, which is expressed as
Isc is the short-circuit current, Ki is current conductivity, T is the module temperature, and G is solar irradiance, while To and Go are the temperature and solar irradiance at STC which are 25°C and 1000 W/m2, respectively (Zainal and Yusoff, 2016).
Thus, the resistance current can be mathematically expressed as
Iscr represents short-circuit current, Voc is open-circuit voltage, q is the charge on electron, Ns is the number of PV cells in one module, k is Boltzmann constant 1.38 × 10−23 J, and A is diode ideality factor, while diode current is expressed as (Zainal and Yusoff, 2016)
Eg is the bandgap energy, so Eq. 1 becomes (Zainal and Yusoff, 2016)
Rs and Rsh are resistance in series and parallel, respectively; PV modules are made up of connected PV cells that can be arranged in series, parallel combination. The following equation can be used to measure the input power due to irradiance from light sources (Belkassmi et al., 2017):
where Apv is the exposed surface area of the PV module, while the maximum efficiency of the PV module is expressed as an equation (Belkassmi et al., 2017):
Generally, characteristic curves of a PV module can be seen in Figure 4. The red curve depicts variations in current and voltage, while the green curve depicts variations in power and voltage.
A portion of the residual heat that is conducted directly from the bottom of the PV module to the hot side of the TE module is used to generate electrical energy, while the remaining is transferred to the heat sink and eventually to the environment. A TEG’s basic unit is made up of a number of p-type and n-type semiconductor elements. The basic thermoelectric effects for transforming thermal energy into electricity are the Seebeck and Peltier effects. According to the Seebeck effect, an open-circuit voltage is generated in a thermoelectric material as long as a temperature gradient exists across it.
where S is called the Seebeck coefficient or thermopower, a material property that relates open-circuit voltage Voc with the temperature gradient ΔT.
According to the Peltier effect, when an electric current I is passed through a junction of two dissimilar materials, heat P is generated or absorbed at the junction:
where π is the Peltier coefficient and Tj is the junction temperature.
Besides these two effects, there is Joule’s heat caused by TEG electrical current, Fourier’s heat conduction owing to temperature difference through TEG junctions, and Thomson’s heat caused by both temperature difference and electrical current. Based on all of these results, the heat flow rate from the rear side of the PV module to the TEG hot face and from the TEG cold face to the heat sink is expressed as
where Qh and Qc are the heat flow rates from the hot and cold side of the TE module, respectively, N denotes the number of thermoelectric pairs, R represents the electrical resistance, and K is the thermal conductance of one thermoelement pair.
The electrical currents and voltage generated from TEG are expressed as
where Rin is the internal resistance of the TE module (Rin= NR) and Rload is the external load resistance. So, the output power generated by TEG is given as
And the efficiency of the TE module is given as
The overall conversion efficiency of the hybrid PV-TEG system can be expressed as the sum of the individual efficiencies of PV (ηpv) and TEG (ηteg) (Narducci et al., 2018).
where Ppv is the output power of the PV module, Pteg is the TEG output power, Apv is the surface area of the module, calculated by multiplying the length with the width of the PV module, and Pin is the input solar power.
Figure 5 shows the schematic stages of hybrid PV-TEG mathematical modeling calculations. The sum of PV and TEG output determines total power output and overall efficiency.
Experimental setup, consisting of solar panels without thermoelectric modules and with thermoelectric modules, is shown in Figures 6A,B, respectively. In this work, two 10-W polycrystalline silicon-based PV panels are used. As an alternate method for lowering the operating temperature of the PV module and generating electrical power at the same time, ten thermoelectric modules were glued on the rear side of one photovoltaic panel at appropriate distances. The results for both simple and hybrid systems are presented, and it is clear that a hybrid system boosted output performance parameters of PV module, like open-circuit voltage, current, power, and conversion efficiency.
Two polycrystalline photovoltaic panels of 10 W were used to carry out the comparative performance analysis as shown in Figure 7.
Photovoltaics were positioned at a fixed tilt angle of 46° towards the south. To evaluate the performance of a simple PV and hybrid PV-TEG system, the setup was tested under the climate conditions of Lahore (31.5204° N, 74.3587° E), Pakistan. Typical 10 consecutive days of winter from February 25th to March 6, 2021, were chosen to collect the experimental data. The experiments were carried out from 8 a.m. to 4 p.m. The specifications of the PV module used in these experiments are given in Table 1.
Bismuth telluride (Bi2Te3) thermoelectric modules were used in this study since they are the most commercially available on the market. Table 2 shows the specifications of one TE module.
Irradiance data for a prescribed location were extracted from Tutiempo Network, S.L. Temperature, current, and voltage were manually measured on daily basis with 1 h interval from 8 a.m. to 4 p.m. A k-type thermocouple was used to measure the temperature of the rear sides of both panels at various points and an average temperature of the rear side was recorded. A UT33B+ digital multimeter (DMM) was used to measure the output voltage and current of the simple PV system and hybrid PV-TEG system. The power output of both systems was calculated by multiplying voltage by current, and the efficiency of both systems was calculated using the analytical relation from Eq. 17. After the acquisition of experimental data, output parameters were plotted with time using MATLAB.
Since literature has revealed that the conversion efficiency of PV module is reduced due to an increase in its temperature, this designed methodology utilized thermoelectric cooling in conjunction with power generation to extract heat from the PV module. After analyzing experimental averaged data for 10 consecutive days of winter from February 25 to March 6, 2021, it is discovered that all of the performance parameters of this hybrid PV-TEG system, including voltage, current, power, and efficiency, have improved.
Figure 8A depicts the transient variation of solar irradiance and PV module operating temperature with simple and hybrid systems. Solar irradiance data for our prescribed location were extracted from Tutiempo Network, S.L. Temperatures of a simple PV module and a hybrid system were manually measured using a k-type thermocouple every hour from 8 a.m. to 4 p.m. on a daily basis. Temperatures were taken at various locations on the back surfaces of both panels, and an average temperature was calculated. It has been noted that the maximum solar irradiance during this 10-day period occurred between 11 a.m. and 12 p.m. In addition, for both systems, the maximum module operating temperature was at this time (12 p.m.). The maximum average temperature measured for the simple PV system was 55°C. When TEG modules were connected to it, the operating temperature of this hybrid system dropped to 52°C as shown in Figure 8B.
FIGURE 8. Transient variation of (A) solar irradiance and (B) PV module operating temperature, respectively.
Figures 9A,B depict the transient variation of voltage and current, respectively, in a simple as well as hybrid PV system. A UT33B+ digital multimeter (DMM) was used to measure voltage and current of simple and hybrid PV system. The hybrid system’s open-circuit voltage is actually the sum of the voltages produced by the PV and TEG modules. It is discovered that the open-circuit voltage for both simple and hybrid systems reached its maximum value of 19.73 and 21.43 V, respectively, at 12 p.m. noon, as shown in Figure 9A. Also, the short-circuit current in both cases followed the same trend as voltage. At 8 a.m., the electrical current values for simple and hybrid PV system are 0.184 A–0.213, respectively. The maximum value for a simple PV system that occurred at 12 p.m. is 0.445 A, while it is 0.506 A for the hybrid PV system as shown in the current vs. time graph in Figure 9B.
FIGURE 9. Transient variation of (A) voltage and (B) current of simple PV and hybrid PV-TEG system, respectively.
Figures 10A,B depict the transient variation of power and conversion efficiency, respectively, in a simple as well as hybrid PV system. It is noticed that, due to a drop in temperature and the addition of some recovered energy by thermoelectric modules, both the total output power and conversion efficiency of the hybrid PV-TEG system are enhanced. When compared to a simple PV system, the hybrid PV-TEG system’s total output power increased by 19%, from 8.78 to 10.84 W. The maximum power for both systems reached at 12 p.m. as presented in the power vs. time graph of Figure 9A. Also, the overall conversion efficiency of the aforementioned two systems occurred between 11 a.m. and 12 p.m. with numerical values of 11.6 and 14%, respectively.
FIGURE 10. Transient variation of (A) output power and (B) efficiency of simple PV and hybrid PV-TEG system, respectively.
In this work, an experimental and comparative performance evaluation of a simple flat plate polycrystalline PV system and a hybrid PV-TEG system is analyzed. All performance parameters including current, voltage, power, and efficiency for both systems are calculated over a ten-day period in Lahore, Pakistan, from February 25 to March 6, 2021. The experimental results briefly show that a hybrid PV-TEG system boosted output power and efficiency. The following conclusions can be drawn based on the experimental averaged data gathered for 10 days:
• The operating temperature of the PV module in the hybrid system dropped from 55°C to 52°C, which is 5.5% lower than the simple PV system.
• Due to a drop in temperature and the addition of some recovered energy by thermoelectric modules, the total output power of the hybrid system increased from 8.78 to 10.84 W, which is 19% more than the simple PV system.
• Efficiency of the hybrid PV-TEG system increased from 11.6 to 14%, which is an increase of 17% overall.
This work concludes that thermoelectric cooling in combination with power generation is a considerable way to improve the PV module’s output power and conversion efficiency. This hybrid PV-TEG system proposes effective use of residual heat accumulated on PV module resulting in improved performance when compared to the simple PV systems. The findings of this research could provide consideration for designing commercial hybrid PV-TEG systems ranging from domestic rooftop applications to large-scale solar power stations.
The original contributions presented in the study are included in the article/supplementary material; further inquiries can be directed to the corresponding author.
All authors listed have made a substantial, direct, and intellectual contribution to the work and approved it for publication.
The authors declare that the research was conducted in the absence of any commercial or financial relationships that could be construed as a potential conflict of interest.
All claims expressed in this article are solely those of the authors and do not necessarily represent those of their affiliated organizations or those of the publisher, the editors, and the reviewers. Any product that may be evaluated in this article or claim that may be made by its manufacturer is not guaranteed or endorsed by the publisher.
Arabkoohsar, A., Machado, L., Farzaneh-Gord, M., and Koury, R. N. N. (2015). The First and Second Law Analysis of a Grid Connected Photovoltaic Plant Equipped with a Compressed Air Energy Storage Unit. Energy 87, 520–539. doi:10.1016/j.energy.2015.05.008
Babu, C., and Ponnambalam, P. (2018). managementThe Theoretical Performance Evaluation of Hybrid PV-TEG System. Energ. Convers. Manag. 173, 450–460. doi:10.1016/j.enconman.2018.07.104
Baruch, P., De Vos, A., Landsberg, P. T., and Parrott, J. E. (1995). On Some Thermodynamic Aspects of Photovoltaic Solar Energy Conversion. Solar Energ. Mater. Solar Cell 36 (2), 201–222. doi:10.1016/0927-0248(95)80004-2
Belkassmi, Y., Rafiki, A., Gueraoui, K., Elmaimouni, L., Tata, O., and Hassanain, N. (2017). Modeling and Simulation of Photovoltaic Module Based on One Diode Model Using Matlab/Simulink, Proceedings of the International Conference on Engineering & MIS (ICEMIS), Monastir, Tunisia, May 8–10, 2017. IEEE. doi:10.1109/icemis.2017.8272965
Bicer, Y., Dincer, I., and Zamfirescu, C. (2016). A Holistic Approach to Thermodynamic Analysis of Photo-Thermo-Electrical Processes in a Photovoltaic Cell. Energ. Convers. Manag. 123, 218–231. doi:10.1016/j.enconman.2016.05.090
Cotfas, D. T., Cotfas, P. A., Machidon, O. M., and Ciobanu, D. (2016). Investigation of the Photovoltaic Cell/thermoelectric Element Hybrid System Performance. IOP Conf. Ser. Mater. Sci. Eng. 133, 012037. doi:10.1088/1757-899x/133/1/012037
Dallan, B. S., Schumann, J., and Lesage, F. J. (2015). Performance Evaluation of a Photoelectric-Thermoelectric Cogeneration Hybrid System. Solar Energy 118, 276–285. doi:10.1016/j.solener.2015.05.034
Deng, Y., Zhu, W., Wang, Y., and Shi, Y. (2013). Enhanced Performance of Solar-Driven Photovoltaic-Thermoelectric Hybrid System in an Integrated Design. Solar Energy 88, 182–191. doi:10.1016/j.solener.2012.12.002
Dimri, N., Tiwari, A., and Tiwari, G. N. (2017). Thermal Modelling of Semitransparent Photovoltaic thermal (PVT) with Thermoelectric Cooler (TEC) Collector. Energ. Convers. Manag. 146, 68–77. doi:10.1016/j.enconman.2017.05.017
Esmaili, P., Dincer, I., and Naterer, G. F. (2015). Development and Analysis of an Integrated Photovoltaic System for Hydrogen and Methanol Production. Int. J. Hydrogen Energ. 40 (34), 11140–11153. doi:10.1016/j.ijhydene.2015.04.077
Grubišić-Čabo, F., Nižetić, S., and Giuseppe Marco, T. J. T. O. F. (2016). Photovoltaic Panels: A Review of the Cooling Techniques. Trans. FAMENA 40 (SI-1), 63–74.
He, W., Zhang, G., Zhang, X., Ji, J., Li, G., and Zhao, X. (2015). Recent Development and Application of Thermoelectric Generator and Cooler. Appl. Energ. 143, 1–25. doi:10.1016/j.apenergy.2014.12.075
Huen, P., Daoud, W. A., and Reviews, S. E. (2017). Advances in Hybrid Solar Photovoltaic and Thermoelectric Generators. Renew. Sust. Energ. Rev. 72, 1295–1302. doi:10.1016/j.rser.2016.10.042
Joshi, A. S., Dincer, I., and Reddy, B. V. (2014). Solar Exergy Maps for Photovoltaic/thermal Systems. Int. J. Exergy 14 (2), 191–211. doi:10.1504/ijex.2014.060283
Kabeel, A. E., Sathyamurthy, R., El-Agouz, S. A., Muthu manokar, A., and El-Said, E. M. S. (2019). Experimental Studies on Inclined PV Panel Solar Still with Cover Cooling and PCM. J. Therm. Anal. Calorim. 138 (6), 3987–3995. doi:10.1007/s10973-019-08561-6
Khan, J., Arsalan, M. H., and Reviews, S. E. (2016). Solar Power Technologies for Sustainable Electricity Generation - A Review. Renew. Sust. Energ. Rev. 55, 414–425. doi:10.1016/j.rser.2015.10.135
Kossyvakis, D. N., Voutsinas, G. D., and Hristoforou, E. V. (2016). Experimental Analysis and Performance Evaluation of a Tandem Photovoltaic-Thermoelectric Hybrid System. Energ. Convers. Manag. 117, 490–500. doi:10.1016/j.enconman.2016.03.023
Lakatos, L., Hevessy, G., and Kovács, J. (2011). Advantages and Disadvantages of Solar Energy and Wind-Power Utilization. World Futures 67 (6), 395–408. doi:10.1080/02604020903021776
Li, G., Feng, W., Jin, Y., Chen, X., and Ji, J. (2017). Discussion on the Solar Concentrating Thermoelectric Generation Using Micro-channel Heat Pipe Array. Heat Mass. Transfer 53 (11), 3249–3256. doi:10.1007/s00231-017-2026-3
Li, G., Shittu, S., Diallo, T. M. O., Yu, M., Zhao, X., and Ji, J. (2018). A Review of Solar Photovoltaic-Thermoelectric Hybrid System for Electricity Generation. Energy 158, 41–58. doi:10.1016/j.energy.2018.06.021
Li, Y., Witharana, S., Cao, H., Lasfargues, M., Huang, Y., and Ding, Y. (2014). Wide Spectrum Solar Energy Harvesting through an Integrated Photovoltaic and Thermoelectric System. Particuology 15, 39–44. doi:10.1016/j.partic.2013.08.003
Lin, J., Liao, T., and Lin, B. (2015). Performance Analysis and Load Matching of a Photovoltaic-Thermoelectric Hybrid System. Energ. Convers. Manag. 105, 891–899. doi:10.1016/j.enconman.2015.08.054
Liu, J., Tang, H., Zhang, D., Jiao, S., Zhou, Z., Zhang, Z., et al. (2020). Performance Evaluation of the Hybrid Photovoltaic-Thermoelectric System with Light and Heat Management. Energy 211, 118618. doi:10.1016/j.energy.2020.118618
Liu, Z., Yuan, S., Yuan, Y., Li, G., and Wang, Q. (2020). A Thermoelectric Generator and Water-Cooling Assisted High Conversion Efficiency Polycrystalline Silicon Photovoltaic System. Front. Energ. 5 (2), 358–366. doi:10.1007/s11708-020-0712-1
Ma, T., Yang, H., Zhang, Y., Lu, L., and Wang, X. (2015). Using Phase Change Materials in Photovoltaic Systems for thermal Regulation and Electrical Efficiency Improvement: a Review and Outlook. Renew. Sust. Energ. Rev. 43, 1273–1284. doi:10.1016/j.rser.2014.12.003
Makki, A., Omer, S., and Sabir, H. (2015). Advancements in Hybrid Photovoltaic Systems for Enhanced Solar Cells Performance. Renew. Sust. Energ. Rev. 41, 658–684. doi:10.1016/j.rser.2014.08.069
Manokar, A. M. J. D., and Treatment, W. (2019). Experimental Study on Effect of Different Mass Flow Rate in an Inclined Solar Panel Absorber Solar Still Integrated with Spiral Tube Water Heater. Desalination Water Treat. 176, 285–291. doi:10.5004/dwt.2020.25531
Manokar, A. M., Winston, D. P., Kabeel, A. E., and Sathyamurthy, R. (2018). Sustainable Fresh Water and Power Production by Integrating PV Panel in Inclined Solar Still. J. Clean. Prod. 172, 2711–2719. doi:10.1016/j.jclepro.2017.11.140
Moh’d A, A.-N., Tashtoush, B. M., Khasawneh, M. A., and Al-Keyyam, I. (2017). A Hybrid Concentrated Solar thermal Collector/thermo-Electric Generation System. Energy 134, 1001–1012. doi:10.1016/j.energy.2017.06.093
Narducci, D., Bermel, P., Lorenzi, B., Wang, N., and Yazawa, K. (2018). Hybrid and Fully Thermoelectric Solar Harvesting, 268. Cham, Switzerland: Springer.
Rejeb, O., Shittu, S., Ghenai, C., Li, G., Zhao, X., and Bettayeb, M. (2020). Optimization and Performance Analysis of a Solar Concentrated Photovoltaic-Thermoelectric (CPV-TE) Hybrid System. Renew. Energ. 152, 1342–1353. doi:10.1016/j.renene.2020.02.007
Rusirawan, D. (2012). Energetic Modeling of Photovoltaic Modules in Grid-Connected Systems. Environ. Sci. PhD Thesis.
Salilih, E. M., and Birhane, Y. T. (2019). Modelling and Performance Analysis of Directly Coupled Vapor Compression Solar Refrigeration System. Solar Energy 190, 228–238. doi:10.1016/j.solener.2019.08.017
Santhi Rekha, S., and Sukchai, S. (2018). Design of Phase Change Material Based Domestic Solar Cooking System for Both Indoor and Outdoor Cooking Applications. J. Solar Energ. Eng. 140 (4). doi:10.1115/1.4039605
Sark, W. G. J. H. M. (2011). Feasibility of Photovoltaic - Thermoelectric Hybrid Modules. Appl. Energ. 88 (8), 2785–2790. doi:10.1016/j.apenergy.2011.02.008
Sasikumar, C., Manokar, A. M., Vimala, M., Prince Winston, D., Kabeel, A. E., Sathyamurthy, R., et al. (2020). Experimental Studies on Passive Inclined Solar Panel Absorber Solar Still. J. Therm. Anal. Calorim. 139 (6), 3649–3660. doi:10.1007/s10973-019-08770-z
Sathe, T. M., Dhoble, A. S., and Reviews, S. E. (2017). A Review on Recent Advancements in Photovoltaic thermal Techniques. Renew. Sust. Energ. Rev. 76, 645–672. doi:10.1016/j.rser.2017.03.075
Shittu, S., Li, G., Zhao, X., Zhou, J., Ma, X., and Akhlaghi, Y. G. (2020). Experimental Study and Exergy Analysis of Photovoltaic-Thermoelectric with Flat Plate Micro-channel Heat Pipe. Energ. Convers. Manag. 207, 112515. doi:10.1016/j.enconman.2020.112515
Shkatulov, A. I., Kim, S. T., Miura, H., Kato, Y., and Aristov, Y. I. (2019). Adapting the MgO-CO2 Working Pair for Thermochemical Energy Storage by Doping with Salts. Energ. Convers. Manag. 185, 473–481. doi:10.1016/j.enconman.2019.01.056
Smil, V. (2005). Energy at the Crossroads: Global Perspectives and Uncertainties. Cambridge, Massachusetts, US: MIT Press.
Sundaram, S., Babu, J. S. C., and management, (2015). Performance Evaluation and Validation of 5MWp Grid Connected Solar Photovoltaic Plant in South India. Energ. Convers. Manag. 100, 429–439. doi:10.1016/j.enconman.2015.04.069
Thirugnanasambandam, M., Iniyan, S., and Goic, R. (2010). A Review of Solar thermal Technologies☆. Renew. Sust. Energ. Rev. 14 (1), 312–322. doi:10.1016/j.rser.2009.07.014
Verma, V., Kane, A., and Singh, B. (2016). Complementary Performance Enhancement of PV Energy System through Thermoelectric Generation. Renew. Sust. Energ. Rev. 58, 1017–1026. doi:10.1016/j.rser.2015.12.212
Wang, N., Han, L., He, H., Park, N.-H., and Koumoto, K. (2011). A Novel High-Performance Photovoltaic-Thermoelectric Hybrid Device. Energy Environ. Sci. 4 (9), 3676–3679. doi:10.1039/c1ee01646f
Zainal, N. A., and Yusoff, A. R. (2016), Modelling of Photovoltaic Module Using Matlab Simulink, IOP Conference Series: Materials Science and Engineering, Volume 114, 2nd International Manufacturing Engineering Conference and 3rd Asia-Pacific Conference on Manufacturing Systems (iMEC-APCOMS 2015), Kuala Lumpur, Malaysia, November 12–14, 2015. Bristol, England: IOP Publishing.
Zhang, J., Xuan, Y., and Yang, L. (2014). Performance Estimation of Photovoltaic-Thermoelectric Hybrid Systems. Energy 78, 895–903. doi:10.1016/j.energy.2014.10.087
Keywords: photovoltaic, thermoelectric, hybrid, photovoltaic-thermoelectric, experimentation, performance evaluation
Citation: Khan MAI, Khan MI, Kazim AH, Shabir A, Riaz F, Mustafa N, Javed H, Raza A, Hussain M and Salman CA (2021) An Experimental and Comparative Performance Evaluation of a Hybrid Photovoltaic-Thermoelectric System. Front. Energy Res. 9:722514. doi: 10.3389/fenrg.2021.722514
Received: 08 June 2021; Accepted: 04 August 2021;
Published: 30 September 2021.
Edited by:
Ravishankar Sathyamurthy, KPR Institute of Engineering and Technology, IndiaReviewed by:
Muthu Manokar A., B. S. Abdur Rahman Crescent Institute Of Science And Technology, IndiaCopyright © 2021 Khan, Khan, Kazim, Shabir, Riaz, Mustafa, Javed, Raza, Hussain and Salman. This is an open-access article distributed under the terms of the Creative Commons Attribution License (CC BY). The use, distribution or reproduction in other forums is permitted, provided the original author(s) and the copyright owner(s) are credited and that the original publication in this journal is cited, in accordance with accepted academic practice. No use, distribution or reproduction is permitted which does not comply with these terms.
*Correspondence: Chaudhary Awais Salman, YXdhaXMuc2FsbWFuQG1kaC5zZQ==
Disclaimer: All claims expressed in this article are solely those of the authors and do not necessarily represent those of their affiliated organizations, or those of the publisher, the editors and the reviewers. Any product that may be evaluated in this article or claim that may be made by its manufacturer is not guaranteed or endorsed by the publisher.
Research integrity at Frontiers
Learn more about the work of our research integrity team to safeguard the quality of each article we publish.