- State Key Laboratory of Clean Energy Utilization, Zhejiang University, Hangzhou, China
The evolution behavior of the light tar during coal fast pyrolysis under inert gas, simulated fuel gas (SFG) atmosphere, and catalytic reformation of simulated (CRS) fuel gas over Ni/Al2O3 was studied in this article. The light tar was recovered from the distillation of the crude tar at the temperature of 300°C and subsequently subjected to detection through the GC-MS analysis. It was found that both SFG and CRS over Ni/Al2O3 significantly enhanced the light tar yield, but a little effect was shown on the heavy tar yield. According to the molecular structure characteristics, the compounds in the light tar could be classified into several groups: aromatic components, phenol components, aliphatic components, heteroatom components, and O-containing components (phenol compounds excluded). It was demonstrated that the selectivity of each component in the light tar varied significantly with the pyrolysis atmosphere and temperature. The evolution of the aromatic components took the dominant role in the light tar produced at high temperature. The SFG and CRS contributed markedly to enhancing the evolution of the O-aromatic components in the light tar, whereas they suppressed the evolution of the O-aliphatic components and the phenol components in the light tar at high temperature.
Introduction
To alleviate the pressure on the environment pollution from the direct coal fired power generation, some effective efforts were paid to high-valued and clean coal utilization (Ding et al., 2020). In particular, the coal tar evolving from the coal poly-generation could be widely used as chemical feedstock for extracting high-value chemicals and as crude oil for producing diesel oil and gasoline at adequate reaction conditions (Qi et al., 2014; Gang et al., 2018). Many of such efforts were devoted to pursuing more tar yield. Several types of reactors were used for coal pyrolysis, such as fixed bed reactor, Curie Point reactor, moving bed reactor, and fluidized bed reactor. The experimental results showed that the fluidized bed reactor was a promising way to achieve high tar yield. More tar yield was normally obtained during the coal fast pyrolysis in a fluidized bed reactor for industry (Gonenc et al., 1990). Meanwhile the heavy components in the crude tar took a high proportion in the crude tar produced through a fluidized bed reactor and made some inconvenience for further utilization (Li and Suzuki, 2010; Liu et al., 2016). High value compounds, such as BTX (benzene, toluene, and xylene) and PCX (phenol, cresol, and xylenol), were concentrated in light components in the crude tar yield, and pursuing more light tar yield during the coal pyrolysis process was preferable.
The hydro-pyrolysis was a considerably effective way to improve the light tar yield (Strugnell and Patrick, 1996; Ariunaa et al., 2007; Scaccia et al., 2012). The hydrogen atmosphere could provide hydrogen active radicals which were used to stabilize the volatile intermediates from the coal pyrolysis and enhance the light tar evolution (Liu, 2014). But the expensive hydrogen also increases the cost of the hydro-pyrolysis. Seeking inexpensive substitution of H2 for lowing the cost of the coal hydro-pyrolysis was necessary. The reductive mixtures of CH4 and H2 were selected to promote the light tar yield (Cypres and Baoqing, 1988; Smith et al., 1989; Fidalgo et al., 2014). In addition, the fuel gas produced from the coal conversion process with high contents of H2, CH4, and CO was selected as a cheap substitution of expensive pure H2 for the coal hydro-pyrolysis (Braekman-Danheux et al., 1992; Braekman-Danheux et al., 1995; Liao et al., 1998; Ni et al., 2014). Compared to the natural gas and pure hydrogen, this coal fuel gas was not needed to afford extra expense of transportation, purification, and separation of the atmosphere. In addition, the CO gas also facilitated enhancing the light tar evolution during the coal pyrolysis. These were far-reaching significant to improve the light tar yield at a low cost through the coal hydro-pyrolysis because all the raw materials were from coal. Meanwhile these experimental results revealed that the activity of the mixture gas was related to H2, but CH4 was inert and showed little effect on improving the light tar yield.
The catalytic pyrolysis was another effective way to strengthen the light tar evolution. It had been revealed that CH4 reform reaction activated by kinds of catalysts produced some active radicals, such as CHx- and H- (Suuberg et al., 1980; Guo et al., 2014). These free radicals from the CH4 dissociation could be used to stabilize the intermediate coal radicals and improve the light tar yield by integrating the coal pyrolysis with the methane reformation process (Wu et al., 2018; Jin et al., 2019; Zhao et al., 2019; Lv et al., 2020). Noble metals, such as Pt, Pd, and Ru, showed perfect performance on activating the CH4 reformation process but they were so expensive which made them hardly deployed in the industrial scale coal pyrolysis (Mattos et al., 2003; Porosoff et al., 2015; Usman et al., 2015; Chung and Chang, 2016). Alternatively, the transition metals, such as Ni, Co, and Mo, used as an economic substitution of the noble metals also presented significantly positive effects on catalyzing the CH4 reform reaction (Li et al., 2015; Amin et al., 2016; Karthikeyan et al., 2017; Mousavi et al., 2017; Bilge et al., 2018; Zhang et al., 2018). So far, most of coal pyrolysis integrating with the catalytic reformation reaction activated by the transition catalysts was operated in the fixed bed reactor. However, compared to the fluidized and stirred status of the coal particles in the fluidized bed reactor, the static status of the coal particles in the fixed bed reactor resulted in weak release of volatile matter products, such as tar and char, which led to relatively low tar and gas yield.
In our previous research, the poly-generation system integrating a CFB combustion reactor and a CFB pyrolysis reactor into a combined system had been constructed and operated well to produce coal fuel gases, tar and char (Ni et al., 2014). Some parts of the coal fuel gas released from the coal pyrolysis reactor were recycled to the coal pyrolysis reactor as the fluidizing gas of the coal hydro-pyrolysis in this poly-generation system. On the basis of previous work, in this research work, the attention was mainly focused on the integration of the fuel gas catalytic reformation and the coal pyrolysis to further promote the light tar yield. In order to carry out the experiment conveniently, we simplified the pyrolysis process. The heat was provided by electric silicon carbide rod. The simulated fuel gas was prepared from cylinders and used as a substitution of the fuel gas that evolved from the coal pyrolysis. The coal fast pyrolysis in a fluidized bed reactor integrated with the catalytic reformation of simulated fuel gas (CRS), a mixture of H2, CH4, CO, and CO2, was deployed over the Ni/Al2O3 catalyst to confirm the promotion of the light tar yield. The coal pyrolysis both under the simulated fuel gas (SFG) atmosphere and under the N2 atmosphere was also performed to further unveil the roles of SFG and CRS, respectively. In addition, the components of the light tar were classified into several groups according to the characteristics of the molecular structure and the evolution behavior of these groups during the coal pyrolysis under three different atmospheres was also investigated.
Experimental Section
Preparation of the Ni/Al2O3 Catalyst
The aluminum oxide (Al2O3) particles with the size of 3–5 mm were dried at 105°C for 8 h in an oven. The ferric nitrate crystals were dissolved in ion-free water and 4 wt% of ferric nitrate solution was obtained. Then the Al2O3 particles mentioned above were immersed into this ferric nitrate solution and maintained for 12 h. The Al2O3 particles with loading ferric nitrate were calcined at 600°C for 4 h. Finally, the calcined Al2O3 particles were heated and reduced at 500°C for 3 h in the H2 atmosphere. The obtained Ni/Al2O3 catalyst was collected and used for the coal fast pyrolysis. The composition of the Ni/Al2O3 catalyst was analyzed through an energy dispersive spectrum (EDS) instrument, and the analysis results are presented in Table 1. In addition, the micro structure of the Ni/Al2O3 catalyst was measured through the BET surface area measurement, and the results are listed in Table 2.
Experimental Process
The thermogravimetric analysis (TG) was carried out through Mettler-Toledo TGA/SDTA851e. The coal particles were crushed, and 20 mg pulverized coal sample was loaded into the crucible and heated from 20 to 1,000°C with a heating rate of 20°C/min and then held for 30 min.
The fast pyrolysis of the bituminous coal was operated at 500–800°C in a bench-scale fluidized bed reactor. The schematic of the fast pyrolysis process is shown in Figure 1. The fluidized bed reactor consisted of three main parts: preheating part (20 mm i.d. and 4.3 m in length), reaction part (50 mm i.d. and 1.2 m in length), and tar recovery system. The preheating section and the reaction section were heated to target temperature by electric silicon carbide rods. The tar recovery system is cooled by the mixture of ethanol and dry ice whose temperature could reach nearly −30°C.
The crushed coal particles with the size of 1–2 mm were selected as feedstock. The results of the proximate analysis and the ultimate analysis of bituminous coal are shown in Table 3. The N2 gas and the simulated fuel gas were used as fluidizing gas. According to previous research results (Ni et al., 2014), the main components of the fuel gas released from the coal pyrolysis in the fluidized bed reactor were CH4, H2, CO, and CO2. The concentrations of CH4, H2, CO, and CO2 in the fuel gas produced at 600°C and recycled to the pyrolysis reactor were approximately 25–35%, 25–35%, 15–25%, and 5–15%, respectively. So the components of CH4, H2, CO, and CO2 in the simulated fuel gas were prepared in a ratio of 3:3:2:1 for this research. The flow rate of the fluidizing gas was kept at 30L/min controlled by the mass flowmeters. The silica sands with a diameter of 0.2–0.4 mm were chosen as the fluidizing material of the fluidized bed reactor. As illustrated in Figure 1, the Ni catalyst was installed in the upstream location between the preheating section and the reaction section in order to activate the fluidizing gas prior to reaching the reaction zone of the fluidizing bed reactor.
When the target pyrolysis temperature was achieved, the coal particles were fed into the fluidized bed reactor through sand hopper located at the top of the reactor. The feed rate of coal was set at 30 g per trial. The coal particles were maintained for 3 min in the reaction zone of the fluidized bed reactor. Then the formed char and silica sands were both discharged into char hopper located at the bottom of the reactor. Then the hot mixture of the char and silica sands in the char hopper was cooled to ambient temperature at the atmosphere of nitrogen gas. The hot volatile products that evolved from the bituminous coal fast pyrolysis in the reactor were carried by the fluidizing gas into the tar recovery system. The crude tar components in the hot volatile products were condensed and trapped in the tar recovery system. This resulted in the separation of the crude tar and fuel gas. The uncondensed fuel gas flow out from the tar recovery system. The collected tar product would be subjected to further analysis. The experimental data listed in this article were the averages of three repeated experiments.
Tar Recovery and Measurement
The crude coal tar produced from the coal fast pyrolysis was recovered through dichloromethane solution from the trap located in the cooling bath at −20°C. The water product was separated from the mixture of the crude tar and dichloromethane solution through a pear-shaped funnel. The mixture of the crude tar and dichloromethane solution was subsequently subjected to de-dichloromethane treatment by a rotary evaporation. Then the obtained crude tar was distilled at 300°C and 100 mbar by Buchi-585 evaporator to achieve light tar recovery from the crude tar. The GC-MS analysis was then employed to detect the distribution of the main compounds contained in the light tar fraction. The capillary column was equipped by GC to separate the light compounds of the light tar. The maximum temperature of the oven of GC could reach 300°C. The temperature program was the same as that in a previously published literature (Li et al., 2016). Finally, all the obtained GC-MS data were subjected to a systematical analysis according to the characteristics of the aromatic ring structure of molecular of compounds.
Results and Discussion
Light Tar Evolution Behavior
The crude tar yield under CRS over the Ni/Al2O3 catalyst, SFG, and N2 atmosphere as a function of pyrolysis temperature are presented in Figure 2A. It could be observed that the crude tar yield increased firstly and subsequently decreased with the coal pyrolysis temperature increasing. The maximum tar yield was achieved at 600°C under the three different atmospheres. According to the weight loss behavior under the N2 atmosphere shown in Figure 3, the primary coal pyrolysis was initiated at approximately 400°C. With temperature increasing, the primary pyrolysis reaction became stronger and enhanced the tar oil evolution. However, when the pyrolysis temperature increased to above 600°C, the second pyrolysis reaction happened and the volatile products from pyrolysis were consumed as reactants. As a result, the tar yield was declined at higher pyrolysis temperature due to the second pyrolysis reaction.
The crude tar yield under the CRS and SFG atmosphere showed a higher level than that under the N2 atmosphere. Moreover, the crude tar yield under the CRS atmosphere was the highest. The maximum yields under the SFG and N2 atmospheres were 11 and 9.7 wt%, respectively. With coupling the catalytic reformation of the simulated fuel gas, the highest tar yield under the CRS atmosphere increased to 13 wt%, which was 1.2 and 1.34 times those under the SFG and N2 atmospheres, respectively. The char yield curves listed in Figure 2B declined with the pyrolysis temperature. The char yield under the SFG and CRS atmospheres was lower than that under the N2 atmosphere.
It had been published that the -CHx and -H radicals could be formed in the CH4 reformation reactions (Guo et al., 2014), which could be used to stabilize the intermediate radicals that originated from the coal pyrolysis and suppress the crosslink reactions that happened between the macro-molecular radicals to improve the tar yield. These reformation reactions were more active at high temperature and more small radicals were produced to stabilize the coal pyrolysis free radicals. As a result, more crude tar was obtained under the CSR atmosphere than that under the SFG and N2 atmospheres, respectively, especially at high pyrolysis temperature.
The total volumes of the gas products under different atmospheres are shown in Figure 4. At 500–600°C, the volume of gas products from the coal pyrolysis under SFG (CP-SFG) and the coal pyrolysis integrated with CRS (CP-CRS) was less than that of the sum of the gas products from the coal pyrolysis under N2 (CP-N2) plus the volume of SFG which was fed into the reactor. The decrease in the gas volume suggested that some gas components were activated to produce free radicals to take part in the coal pyrolysis and resulted in increment of the crude tar yield listed in Figure 2A. However, when the temperature increased to 800°C, the gas products yield of CP-SFG and CP-CRS increased to a higher level than that of CP-N2 plus SFG.
Further analysis was conducted, and the gas components volume difference between CP-CRS and CP-N2 plus SFG at different temperature is shown in Figure 5. The CP-CRS process had a marked decrease of H2 and CH4 at 500–600°C after the Ni/Al2O3 catalyst. This further suggested that H2 and CH4 were activated by the Ni/Al2O3 catalyst and took part in the coal pyrolysis and increased the crude tar yield. At 800°C, the strong reform reaction of CH4 and CO2 achieved a considerable increase of H2 and CO with a sharp decrease of CH4 and CO2 after the Ni/Al2O3 catalyst. More free radicals or active gas formed in the catalytic reform reaction process participated in the pyrolysis reaction and suppressed the decline of the tar yield. Meanwhile the increase of the H2 and CO products made significant contributions to the increment of the total gas volume under SFG and CRS in Figure 4 at high temperature.
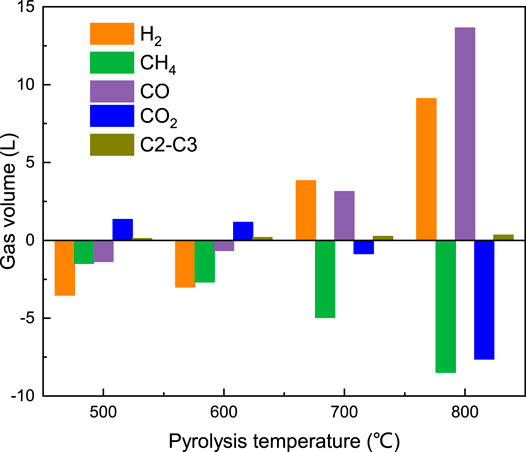
FIGURE 5. Volume difference of the gas components between SFG plus CP-N2 and CP-CRS versus temperature.
The evolution behavior of the crude tar yield was further elucidated in terms of the light tar and the heavy tar. The yields of the light tar and the heavy tar under the three different atmospheres are shown in Figures 6A,B, respectively. At 500°C, the light tar yield under N2, SFG, and CRS was 4.28, 5.51, and 6.44 wt%, respectively. The order of the light tar yield from high to low was CRS, SFG, and N2. The same variation trend of the light tar yield under the three different atmospheres is also observed in Figure 6A at 600–800°C. This suggested that the SFG and CRS over Ni/Al2O3 were much better to promote the light tar evolution. What is more, the promotion under CRS over Ni/Al2O3 was stronger than that under SFG. The heavy tar yield under the three different atmospheres kept a nearly same level, approximately 4.61–4.78 wt% at 500°C, 4.90–4.95 wt% at 600°C, and 3.62–3.65 wt% at 700°C as shown in Figure 6B. A little promotion effect of SFG and CRS on the heavy tar yield could be observed.
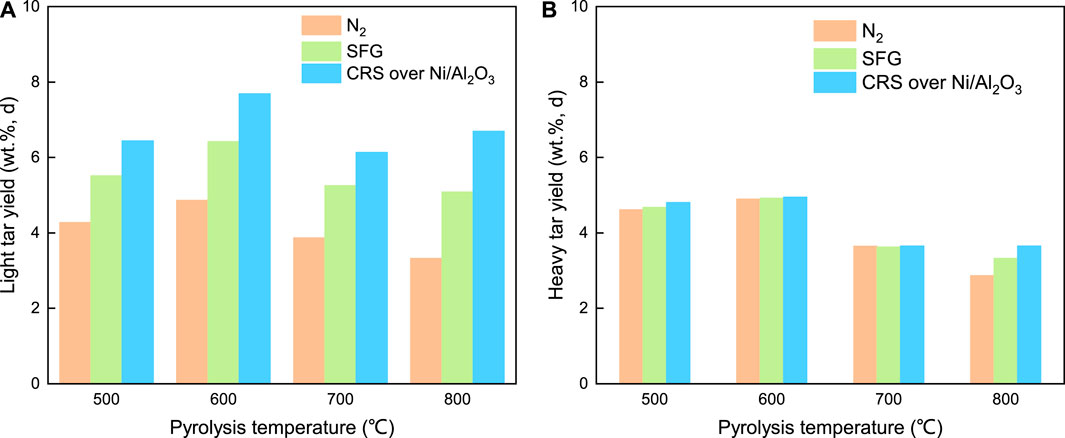
FIGURE 6. The effect of the pyrolysis atmosphere on the yield of the light tar (A) and the heavy tar (B).
By comprehensive comparative consideration of the light tar yield and the heavy tar yield shown in Figures 6A,B, it was found that SFG and CRS over Ni/Al2O3 were preferable to enhance the evolution of the light tar but not the heavy tar during the coal fast pyrolysis in the fluidized bed reactor. This enhancement of the light tar evolution under SFG and CRS made main contributions to increasing the crude tar yield listed in Figure 2A. This was mainly because the molecular weight of the components in the light tar was smaller than that in the heavy tar and the number of molecules of the light tar components was much larger than that of the heavy tar components. As a result, it leaded that the active species from SFG and CRS were captured and bonded more strongly by precursors of light tar than that by precursors of heavy tar. This superiority strengthened the light tar releasing but restrained the heavy tar evolution during the coal fast pyrolysis process under SFG and CRS in the fluidized bed reactor.
In addition, the water yield was a distinct indicator of the catalytic reform reaction of CH4 and the de-hydroxyl reaction of the phenol components. The water yield under the three different atmospheres at different temperature is presented in Figure 7. With the coal pyrolysis temperature increasing, the curve of the water yield under N2 was flat and the curves of the water yield under SFG and CRS ascended sharply. The difference between the water yield under N2 and that under SFG and CRS increased significantly when the pyrolysis temperature increased to above 700°C at which the catalytic reformation reaction happened, shown in Figure 5, and the de-hydroxyl reaction of phenols occurred, presented in Figure 8. More free radicals formed under CRS enhanced the two reactions mentioned above and more water yield was obtained under CRS over Ni/Al2O3.
The Characterization of the Light Tar Components
The systematical analysis of the data obtained from the GC-MS detection of the light tar was conducted. For better understanding of the evolution and distribution behavior of the light tar components in this article, both several hundred aromatic compounds and some non-aromatic compounds discovered in the light tar were classified into five categories: aromatic components, aliphatic components, phenol components, O-containing components (phenols excluded), and other heteroatom components according to the characteristics of the molecular structure and the functional groups. The information of the detailed contents of each category is illustrated in Table 4.
The Evolution Behavior of the Series Varying With the Coal Pyrolysis Temperature
The selectivity of each category in the light tar that evolved from the coal fast pyrolysis under SFG is listed in Figure 9. At 500°C, the selectivity of the aromatic components in the light tar accounted for approximately 30 area%. The selectivity of the phenol components, aliphatic components, and heteroatom compounds remained at a similar level with the values of 20.2 area%, 18.3 area%, and 16.7 area%, respectively. The O-containing components (phenol compounds excluded) presented a lowest selectivity among all the components in the light tar. With the coal pyrolysis temperature increasing, the selectivity of the aromatic components increased monotonously and reached the maximum level at 800°C, accounting for more than 50 area% of the total components’ peak area, whereas the selectivity of the aliphatic components and the heteroatom components decreased. For the phenol components, the maximum selectivity of 25.7 area% was achieved at 600°C and then decreased with the pyrolysis temperature further growing. The selectivity of the O-containing components showed little variation with the coal pyrolysis temperature.
As shown in Figure 10, the selectivity of the aromatic components produced both under the N2 atmosphere and under CRS over Ni/Al2O3 catalyst increased monotonously with the coal pyrolysis temperature as well. In addition, at different temperature, the selectivity of the aromatic components that evolved under CRS over Ni/Al2O3 catalyst presented the highest level (64.7 area% at 800°C), followed by that under SFG (58.3 area% at 800°C) and N2 (56.5 area% at 800°C) in order. These revealed that, compared to the N2 atmosphere, the SFG made a stronger effect on improving the evolution of the aromatic components during the coal fast pyrolysis. The fuel gas atmosphere activated by the Ni catalyst could further enhance the evolution of the aromatic components.
These improvements of the aromatic components’ selectivity under the atmosphere of SFG and CRS over Ni/Al2O3 were achieved at the cost of the phenol components consumption. The de-hydroxyl reaction of the phenol components in the light tar could happen at pyrolysis temperature above 700°C. H free radicals formed in the SFG atmosphere facilitated these de-hydroxyl reactions through the combination of H radicals and hydroxyl groups. The selectivity of the phenol components shown in Figure 8 decreased from 24.4 area% under N2 atmosphere to 17.2 area% under SFG at 700°C. More free radicals could form under CRS over Ni/Al2O3 and further enhanced these de-hydroxyl reactions. The selectivity of the phenol components reduced to 14.8 area% under CRS. Meanwhile these de-hydroxyl reactions were verified by the increment of the water yield listed in Figure 7 under the SFG atmosphere and activated SFG atmosphere. As a result, the de-hydroxyl reactions did a favor to the conversion from the phenol components to the aromatic components and led the selectivity of the aromatic components to increase markedly under SFG and CRS over Ni/Al2O3.
In the other aspect, it was also observed in Figure 11 that the selectivity of the phenol components was higher under SFG than under N2 atmosphere at 500°C. It was indicated that the free radicals from SFG could be used to enhance the phenol components evolution when the de-hydroxyl reaction had not happened at low temperature. More free radicals could be produced under CRS over Ni/Al2O3 and further enhanced the phenol components evolution at a low temperature.
In contrast to the phenol components, the selectivity of the O-containing components (phenols excluded) in the light tar (15 area% at 500°C) listed in Figure 11 which evolved under SFG was much lower than that under the N2 atmosphere (26 area% at 500°C) at low pyrolysis temperature. The evolution of the O-containing components (phenols excluded) was suppressed under SFG and CRS over Ni/Al2O3 at low pyrolysis temperature of 500–600°C. As temperature increased (700–800°C), the selectivity of the O-containing components under the N2 atmosphere decreased to a level similar to that under SFG and CRS over Ni/Al2O3.
Meanwhile the selectivity of the O-containing components under SFG and CRS over Ni/Al2O3 kept a relatively constant level at 500–800°C, respectively. The further analysis was conducted by classifying the O-containing components into two sub-categories consisting of O-aromatic components and O-aliphatic components according to the characteristics of the molecular structure. Under the N2 atmosphere, the selectivity of both the O-aromatic components and the O-aliphatic components in the light tar decreased with the increase of the coal pyrolysis temperature. Meanwhile, under the other two atmospheres, the selectivity of the O-aromatic components and the O-aliphatic components presented a different variation with the coal pyrolysis temperature increasing.
For the O-aliphatic components listed in Figure 12, the selectivity in the light tar under SFG and CRS over Ni/Al2O3 was decreased with the coal pyrolysis temperature. On the contrary, the selectivity of the O-aromatic components in the light tar shown in Figure 13 produced under SFG and CRS over Ni/Al2O3 catalyst increased with the increase of the coal pyrolysis temperature. At 500–600°C, the selectivity of the O-aromatic components that evolved under SFG and CRS over Ni/Al2O3 was lower than that under the N2 atmosphere. When the pyrolysis temperature increased to above 700°C, at which the second pyrolysis reaction happened, the selectivity of the O-aromatic components under SFG and CRS over Ni/Al2O3 increased to a higher level than that under the N2 atmosphere. This was because, at low pyrolysis temperature, the H radicals abounding in SFG and CRS were combined with oxygen existing in volatile products and suppressed the O-aromatic components evolution during the coal fast pyrolysis. At high pyrolysis temperature above 700°C, the reforming reaction between CH4 and CO2 happened. More O-containing active species were formed and facilitated the formation of O-containing heterocycle structure which combined to the aromatic ring structure. It led to the production of more O-aromatic components and increasing the selectivity of O-aromatics in the light tar.
Conclusion
The evolution behavior of the light tar during the coal fast pyrolysis at 500–800°C in a fluidized bed reactor under N2, SFG, and CRS over Ni/Al2O3 was investigated in this article. More crude tar yield was obtained from the coal fast pyrolysis under SFG and CRS over Ni/Al2O3. Compared to the N2 atmosphere, the SFG and CRS over Ni/Al2O3 could strengthen significantly the light tar evolution, but the heavy tar yield was scarcely promoted by SFG and CRS over Ni/Al2O3. The selectivity of only the aromatic components in the light tar was increased monotonously with the pyrolysis temperature and led the aromatic components to take the dominant role in the light tar at high temperature. The SFG and CRS over Ni/Al2O3 enhanced the aromatic components evolution but suppressed the O-aliphatic components. The O-aromatic components evolution was suppressed under SFG and CRS over Ni/Al2O3 at low temperature. The reformation reaction that happened at high temperature is helpful to enhance the evolution of the O-aromatic components. In addition, the evolution of the phenol components under SFG and CRS over Ni/Al2O3 was strengthened at low pyrolysis temperature but reduced at high pyrolysis temperature above 600°C.
Data Availability Statement
The original contributions presented in the study are included in the article/supplementary material; further inquiries can be directed to the corresponding author.
Author Contributions
The authors accomplished this work together. Different degrees of contribution and contributor roles were played by the authors. CL played the leading role in writing-original draft, formal analysis, and methodology. QY operated the experimental work. Professor ZL took the leading supervision work. Professor ZL, Professor MF, and JC shared equal contribution in writing-review and editing. In addition, all authors contributed equally to the conceptualization.
Funding
This work was supported by the National Key R&D Program of China (no. 2018YFB060500).
Conflict of Interest
The authors declare that the research was conducted in the absence of any commercial or financial relationships that could be construed as a potential conflict of interest.
Publisher’s Note
All claims expressed in this article are solely those of the authors and do not necessarily represent those of their affiliated organizations, or those of the publisher, the editors, and the reviewers. Any product that may be evaluated in this article, or claim that may be made by its manufacturer, is not guaranteed or endorsed by the publisher.
References
Amin, M. N., Li, Y., Razzaq, R., Lu, X., Li, C., and Zhang, S. (2016). Pyrolysis of low rank coal by nickel based zeolite catalysts in the two-staged bed reactor. J. Anal. Appl. Pyrolysis 118, 54–62. doi:10.1016/j.jaap.2015.11.019
Ariunaa, A., Li, B.-q., Li, W., Purevsuren, B., Munkhjargal, S., Liu, F.-r., et al. (2007) Coal pyrolysis under synthesis gas, hydrogen and nitrogen. J. fuel Chem. Technol. 35 (1), 1–4. doi:10.1016/s1872-5813(07)60007-3
Bilge, S., Donar, Y. O., and Sınağ, A. (2018). Effect of metal oxide nanoparticles on the evolution of valuable gaseous products during pyrolysis of Turkish low-rank coal. J. Anal. Appl. Pyrolysis 136, 242–247. doi:10.1016/j.jaap.2018.09.019
Braekman-Danheux, C., Cyprès, R., Fontana, A., Laurent, P., and Van Hoegaerden, M. (1992). Coal hydromethanolysis with coke-oven gas: 1. Influence of temperature on the pyrolysis yields. Fuel 71 (3), 251–255. doi:10.1016/0016-2361(92)90069-z
Braekman-Danheux, C., Cyprès, R., Fontana, A., and van Hoegaerden, M. (1995). Coal hydromethanolysis with coke-oven gas. Fuel 74 (1), 17–19. doi:10.1016/0016-2361(94)p4324-u
Chung, W.-C., and Chang, M.-B. (2016). Review of catalysis and plasma performance on dry reforming of CH4 and possible synergistic effects. Renew. Sust. Energ. Rev. 62, 13–31. doi:10.1016/j.rser.2016.04.007
Cypres, R., and Baoqing, L. (1988). Effects of pretreatment by various gases on hyoropyrolysis of a belgian coal. Fuel Process. Tech. 20, 337–347. doi:10.1016/0378-3820(88)90031-8
Ding, Y., Wang, T., Dong, D., and Zhang, Y. (2020). Using Biochar and Coal as the Electrode Material for Supercapacitor Applications. Front. Energ. Res. 7, 159. doi:10.3389/fenrg.2019.00159
Fidalgo, B., van Niekerk, D., and Millan, M. (2014). The effect of syngas on tar quality and quantity in pyrolysis of a typical South African inertinite-rich coal. Fuel 134, 90–96. doi:10.1016/j.fuel.2014.05.032
Gang, Y., Zhang, X., Lei, X., Guo, H., Li, W., and Li, D. (2018). Hydroprocessing of low-temperature coal tar to produce jet fuel. RSC Adv. 8 (42), 23663–23670. doi:10.1039/c8ra04531c
Gonenc, Z. S., Gibbins, J. R., Katheklakis, I. E., and Kandiyoti, R. (1990). Comparison of coal pyrolysis product distributions from three captive sample techniques. Fuel 69 (3), 383–390. doi:10.1016/0016-2361(90)90104-x
Guo, X., Fang, G., Li, G., Ma, H., Fan, H., Yu, L., et al. (2014). Direct, nonoxidative conversion of methane to ethylene, aromatics, and hydrogen. Science 344 (6184), 616–619. doi:10.1126/science.1253150
Jin, L., Zhao, H., Wang, M., Wei, B., and Hu, H. (2019). Effect of temperature and simulated coal gas composition on tar production during pyrolysis of a subbituminous coal. Fuel 241, 1129–1137. doi:10.1016/j.fuel.2018.12.093
Karthikeyan, D., Atchudan, R., Selvakumar, P., Sivakumar, R., and Shanmugam, M. (2017). Effect of preparation methods on structure and catalytic activity of Ni loaded Ce x Zr1−x O2 catalysts for hydrogen production via autothermal reforming of ethane. Res. Chem. Intermed 43 (5), 2817–2837. doi:10.1007/s11164-016-2796-0
Li, C., Fang, M., Xiao, P., Shi, Z., Cen, J., Yan, Q., et al. (2016) Investigation of the evolution behavior of light tar during bituminous coal pyrolysis in a fluidized bed reactor. Chem. Res. Chin. Univ. 32 (6), 1019–1027. doi:10.1007/s40242-016-6091-9
Li, C., and Suzuki, K. (2010). Resources, properties and utilization of tar. Resour. conservation recycling 54 (11), 905–915. doi:10.1016/j.resconrec.2010.01.009
Li, C., Tan, P.-J., Li, X.-D., Du, Y.-L., Gao, Z.-H., and Huang, W. (2015). Effect of the addition of Ce and Zr on the structure and performances of Ni-Mo/CeZr-MgAl(O) catalysts for CH4-CO2 reforming. Fuel Process. Tech. 140, 39–45. doi:10.1016/j.fuproc.2015.08.020
Liao, H., Li, B., and Zhang, B. (1998). Co-pyrolysis of coal with hydrogen-rich gases. 1. Coal pyrolysis under coke-oven gas and synthesis gas. Fuel 77 (8), 847–851. doi:10.1016/s0016-2361(97)00257-3
Liu, P., Le, J., Wang, L., Pan, T., Lu, X., and Zhang, D. (2016). Relevance of carbon structure to formation of tar and liquid alkane during coal pyrolysis. Appl. Energ. 183, 470–477. doi:10.1016/j.apenergy.2016.08.166
Lv, J., Wang, D., Wang, M., Li, Y., Jin, L., and Hu, H. (2020). Integrated coal pyrolysis with dry reforming of low carbon alkane over Ni/La2O3 to improve tar yield. Fuel 266, 117092. doi:10.1016/j.fuel.2020.117092
Mattos, L. V., Rodino, E., Resasco, D. E., Passos, F. B., and Noronha, F. B. (2003). Partial oxidation and CO2 reforming of methane on Pt/Al2O3, Pt/ZrO2, and Pt/Ce–ZrO2 catalysts. Fuel Process. Tech. 83 (1-3), 147–161. doi:10.1016/s0378-3820(03)00063-8
Mousavi, S. M., Meshkani, F., and Rezaei, M. (2017). Preparation of mesoporous nanocrystalline 10% Ni/Ce1−xMnx O2 catalysts for dry reforming reaction. Int. J. Hydrogen Energ. 42 (39), 24776–24784.
Ni, M., Li, C., Fang, M., Wang, Q., Luo, Z., and Cen, K. (2014). Research on coal staged conversion poly-generation system based on fluidized bed. Int. J. Coal Sci. Technol. 1 (1), 39–45. doi:10.1007/s40789-014-0004-7
Porosoff, M. D., Myint, M. N. Z., Kattel, S., Xie, Z., Gomez, E., Liu, P., et al. (2015) Identifying Different Types of Catalysts for CO2Reduction by Ethane through Dry Reforming and Oxidative Dehydrogenation. Angew. Chem. Int. Ed. 54 (51), 15501–15505. doi:10.1002/anie.201508128
Qi, S.-C., Zhang, L., Wei, X.-Y., Hayashi, J.-i., Zong, Z.-M., and Guo, L.-L. (2014). Deep hydrogenation of coal tar over a Ni/ZSM-5 catalyst. RSC Adv. 4 (33), 17105. doi:10.1039/c3ra47701k
Scaccia, S., Calabrò, A., and Mecozzi, R. (2012). Investigation of the evolved gases from Sulcis coal during pyrolysis under N2 and H2 atmospheres. J. Anal. Appl. Pyrolysis 98, 45–50. doi:10.1016/j.jaap.2012.05.001
Smith, G. V., Wiltowski, T., and Phillips, J. B. (1989). Conversion of coals and chars to gases and liquids by treatment with mixtures of methane and oxygen or nitric oxide. Energy Fuels 3 (4), 536–537. doi:10.1021/ef00016a020
Strugnell, B., and Patrick, J. W. (1996). Rapid hydropyrolysis studies on coal and maceral concentrates. Fuel 75 (3), 300–306. doi:10.1016/0016-2361(95)00241-3
Suuberg, E. M., Peters, W. A., and Howard, J. B. (1980). Product compositions in rapid hydropyrolysis of coal. Fuel 59 (6), 405–412. doi:10.1016/0016-2361(80)90193-3
Usman, M., Wan Daud, W. M. A., and Abbas, H. F. (2015). Dry reforming of methane: Influence of process parameters-A review. Renew. Sust. Energ. Rev. 45, 710–744. doi:10.1016/j.rser.2015.02.026
Wu, Y., Li, Y., Jin, L., and Hu, H. (2018). Integrated Process of Coal Pyrolysis with Steam Reforming of Ethane for Improving the Tar Yield. Energy Fuels 32 (12), 12268–12276. doi:10.1021/acs.energyfuels.8b02964
Zhang, F., Liu, Z., Zhang, S., Akter, N., Palomino, R. M., Vovchok, D., et al. (2018). In Situ Elucidation of the Active State of Co-CeOx Catalysts in the Dry Reforming of Methane: The Important Role of the Reducible Oxide Support and Interactions with Cobalt. ACS Catal. 8 (4), 3550–3560. doi:10.1021/acscatal.7b03640
Keywords: fast pyrolysis, light tar, SFG, CRS over Al2O3 catalyst, selectivity
Citation: Li C, Luo Z, Fang M, Yan Q and Cen J (2021) Integrated Process of Coal Fast Pyrolysis in a Fluidized Bed Reactor With Reforming Reaction of Simulated Fuel Gas to Promote Light Tar Evolution. Front. Energy Res. 9:717470. doi: 10.3389/fenrg.2021.717470
Received: 31 May 2021; Accepted: 29 July 2021;
Published: 26 August 2021.
Edited by:
Jiafei Zhao, Dalian University of Technology, ChinaReviewed by:
Tian Li, Norwegian University of Science and Technology, NorwayHongsheng Dong, Dalian Institute of Chemical Physics (CAS), China
Copyright © 2021 Li, Luo, Fang, Yan and Cen. This is an open-access article distributed under the terms of the Creative Commons Attribution License (CC BY). The use, distribution or reproduction in other forums is permitted, provided the original author(s) and the copyright owner(s) are credited and that the original publication in this journal is cited, in accordance with accepted academic practice. No use, distribution or reproduction is permitted which does not comply with these terms.
*Correspondence: Zhongyang Luo, enlsdW9Aemp1LmVkdS5jbg==