- 1Department of Environmental Microbiology, Helmholtz Centre for Environmental Research, Leipzig, Germany
- 2Department of Experimental Ecology of Marine Organisms, Institute of Oceanography, Faculty of Oceanography and Geography, University of Gdańsk, Gdynia, Poland
Wastewater treatment plants (WWTP) are aimed to be transformed from sinks into sources of energy and material. For fostering corresponding engineering efforts and economic assessments, comprehensive knowledge of the energy content of wastewater is required. We show in this proof-of-concept study that these data can be gathered by combining micro-bomb combustion calorimetry with freeze-drying. Thereby, the methodology for measuring the combustion enthalpy (
Introduction
Wastewater treatment plants (WWTPs) belong to the essential infrastructure of modern human societies and contribute to achieving the Sustainable Development Goals (SDGs) postulated by the United Nations General Assembly, especially SDG 6: clean water and sanitation (UN General Assembly, 2015). However, WWTPs consume considerable amounts of electric energy and chemicals for treating wastewater, although wastewater contains 4–6 times more chemical energy than is required for its treatment using conventional technologies (Metcalf & Eddy, Inc et al., 2014; Scherson and Criddle, 2014; Wan et al., 2016; Korth et al., 2017). Thus, reducing energy consumption while increasing energy and resource recovery (e.g., phosphorus and nitrogen) (van der Hoek et al., 2018; Vučić et al., 2021) are necessary improvements for transforming WWTPs from energy sinks to energy-autarkic or even energy-producing facilities.
Besides chemical energy, wastewater also contains thermal energy that bears an excellent exploitation potential, even though it represents low-value exergy (i.e., low share of exergy). Approximately 6–8 times more energy than the chemical energy can be recovered using existing technologies like water source heat pumps for, for example, heating of greenhouses and cooling of buildings (Neugebauer et al., 2015; Shen et al., 2018; Hao et al., 2019). As exploiting its thermal energy is an already well-beaten track, the research focuses on extracting chemical energy and materials from wastewater. This extraction or valorization of chemical energy also represents a sustainable strategy with double benefits as it shall make the energy-demanding degradation of energy-rich compounds in WWTPs obsolete. State-of-the-art energetic use of wastewater is anaerobic digestion of the produced sludge (McCarty et al., 2011). Yet, other biotechnological approaches like microbial electrochemical technologies, complete anaerobic treatment, and different microbial conversions of wastewater compounds in valuable products are under research (Schröder et al., 2015; Kehrein et al., 2020; Zha et al., 2021).
A standard parameter in wastewater analysis that is commonly used for assessing the chemical energy content therein is the chemical oxygen demand (COD). Considering a total theoretical energy potential of 1.96 kWh m−3 of medium-strength municipal wastewater, it captures the largest energy fractions, that is, biodegradable carbon-rich molecules (63% or 1.24 kWh m−3) and inert nonbiodegradable compounds (22% or 0.42 kWh m−3) (Heidrich et al., 2011; Koch et al., 2014; Metcalf & Eddy, Inc et al., 2014; Scherson and Criddle, 2014). In addition, 0.3 kWh m−3 (15%) is based on nitrogen compounds (Scherson and Criddle, 2014). COD measurements are a widespread standard and thus are of particular practical relevance and provide some indications for wastewater’s energy content. Yet, from an engineering perspective, COD does not offer comprehensive thermodynamic information as it only reveals the number of electrons (i.e., energy carriers) within a system but not how much work can be performed by these electrons (i.e., the energy level of these energy carriers). However, this is required for assessing the economic competitiveness of wastewater as a sustainable feedstock for biotechnological processes. Here, the combustion enthalpy (or heat of combustion,
Few studies have analyzed the
Here, we followed an alternative approach and performed a proof-of-concept study in order to use only small-size wastewater samples for obtaining a reliable correlation between
Materials and Methods
Wastewater Sampling and Analysis
Wastewater from the effluent of the primary clarifier of a municipal WWTP with 55,000 population equivalents treating only domestic wastewater was sampled (S1 in Supplementary Material). Once per week (on sequential weekdays), an automated sampling unit collected approximately 800 ml from the primary clarifier effluent every 2 h for 24 h and pooled it. During this procedure, wastewater was stored at 10°C in the sampling system. Approximately 1 h after sampling was finished, 4 × 60 ml were freeze-dried, and standard wastewater parameters were analyzed.
Freeze-Drying of Composite Wastewater Samples
Four pre-dried and weighed serum bottles (V = 100 ml) were each filled with 60 ml wastewater and closed with a freeze-drying stopper (d = 20 mm, Th. Geyer GmbH & Co. KG, Germany). Serum bottles were deep-frozen at −80°C and transferred to the pre-cooled lyophilizer (ALPHA 1–4 LSC, Martin Christ Gefriertrocknungsanlagen GmbH, Germany). Freeze-drying was performed at −20°C and 0.1 mbar for approximately 40 h. Afterward, serum bottles with dry matter were weighed to obtain the wastewater dry weight (
Micro-Bomb Combustion Calorimetry
The energy content was determined using a modified Phillipson KMB-2 type micro-bomb combustion calorimeter (MK-1000) manufactured at the University of Gdansk to study small quantities of biological material (Figure 1) (Drzazgowski et al., 2018). The operational principles of this type of micro-bomb combustion calorimeter were described by Prus (1975). The combustion heat is indirectly measured based on the change in amplified voltage determined using a Pt100 resistance sensor (4-core cable, R0 = 100 Ω). The voltage signal from the sensor (located inside the bomb head) is processed in the A/D converter and digitally transmitted to the microprocessor system. The signal is converted into the temperature value (10 mV per °C), leading to a final
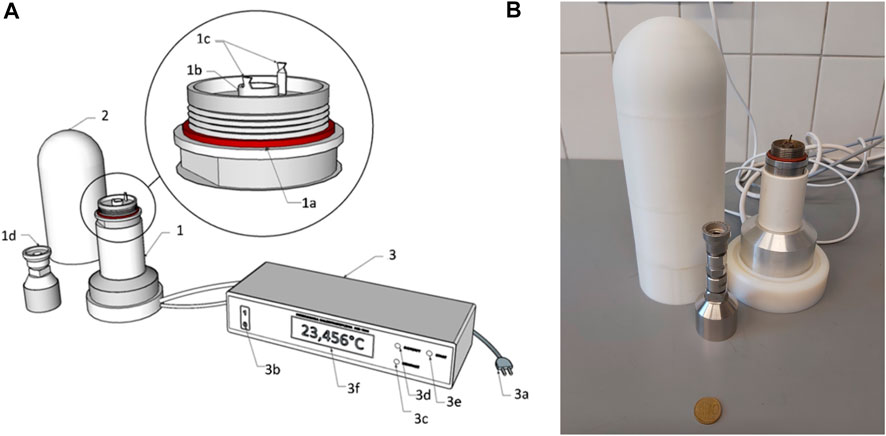
FIGURE 1. Modified Phillipson KMB-2 type micro-bomb combustion calorimeter (MK-1000). (A) Schematic drawing of the micro-bomb combustion calorimeter: 1) bomb stand and head made of corrosion-resistant stainless steel; 1a) silicone O-ring; 1b) sample dish; 1c) electrodes; 1d) bomb head cap with an oxygen valve; 2) insulating jackets; 3) control, ignition and measuring unit; 3a) power cable; 3b) power switch; 3c) control lamp (contact of electrodes through a platinum wire); 3d) control lamp (ready for measurement); 3e) ignition start button; 3f) LC-display. The scheme was adapted from Drzazgowski et al. (2018). (B) Photography for illustrating device proportions.
Subsequently, Eq. 2 yielded the volumetric heat of combustion of wastewater (
Here,
Measuring of Standard Wastewater Parameters
The amount of chemical oxygen demand (COD), nitrate (in mgNO3− L−1), ammonia (in mgNH4+ L−1), total nitrogen (TN, in mgN L−1), and phosphate (in mgPO43− L−1) of composite WW samples were determined using the tests REF 985029, REF 985064, REF 985008, REF 985088, and REF 985055, respectively, of the NANOCOLOR® series (Macherey-Nagel GmbH & Co., KG, Germany) according to the manufacturer’s instructions (ftp://ftp.mn-net.com/english/Instruction_leaflets/NANOCOLOR). Three technical replicates were conducted per single composite wastewater sample. By doing so, nitrate measurements always resulted in negligible concentrations or were below the detection limit (Supplementary Table 1). The biochemical oxygen demand after five days of incubation was determined using OxiTop® (Xylem Analytics Germany Sales GmbH & Co., KG, Germany) according to the manufacturer’s instruction.
Determining Chemical Oxygen Demand Loss During Freeze-Drying
The COD of additional composite wastewater samples were measured as described above, and aliquots of the same wastewater were subjected to the identical drying procedure described in Freeze-Drying of Composite Wastewater Samples. Afterward, dry matter was dehydrated with deionized water, and the COD of the suspension was measured. By doing so, five different wastewater samples were analyzed in triplicate, enabling an assessment of the COD losses during freeze-drying of composite wastewater samples.
Data Analysis
Linear regressions were performed according to Pearson using OriginPro 2019 version 9.6.0.172 (OriginLab Corporation, MA, United States). The suitability of Pearson’s correlation was verified by testing all analyzed parameters (
Results and Discussion
Over 1 year, wastewater from the primary clarifier of one municipal wastewater treatment plant (WWTP, S1) was sampled on a weekly basis, yielding 53 composite samples that were analyzed using standard methods for wastewater characterization. Four aliquots of each sample were freeze-dried. Subsequently, all freeze-dried samples were analyzed by micro-bomb combustion calorimetry, resulting in 158 independent combustion enthalpy (
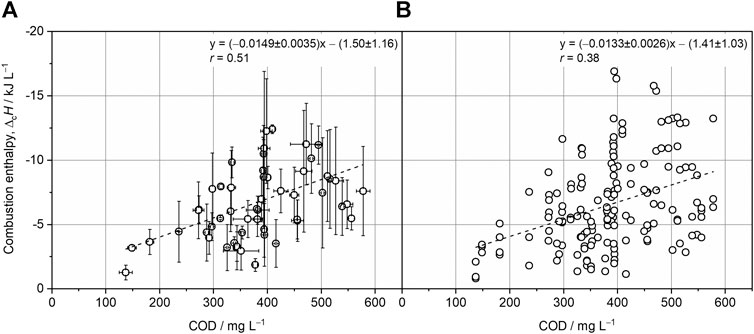
FIGURE 2. Linear regression analysis with the COD of wastewater samples and the respective combustion enthalpies (
During drying of wastewater for combustion calorimetry, the COD loss is an unavoidable experimental drawback, independent of the drying procedure (e.g., freeze-drying or centrifugal evaporation). This loss is mainly ascribed to the evaporation of volatile organic compounds (e.g., acetate) and leads to a systematic underestimation of wastewater’s energy content. Freeze-drying was combined with micro-bomb combustion calorimetry for reducing the residence time of wastewater in the lyophilizer as less than 5% of the sample amount, compared to standard combustion calorimetry, is required for measurements (approx. 30 mg instead of 1 g). Consequently, the loss of volatile organic compounds is also reduced. The COD of composite wastewater samples and the corresponding resuspended dry matter obtained from freeze-drying was measured for evaluating this experimental approach. By analyzing five composite wastewater samples, a mean COD recovery of 71.2 ± 6.1% was determined (Supplementary Material S2), which is lower than the reported COD recovery when using centrifugal evaporation (84.8%) but is in the range of previously reported values for freeze-drying (77.8%) (Dai et al., 2019).
Although measures were taken for increasing the statistical robustness of the analysis (e.g., composite wastewater samples and four technical replicates per composite sample), the measured values of
Regression analysis with further wastewater parameters showed weak correlations of
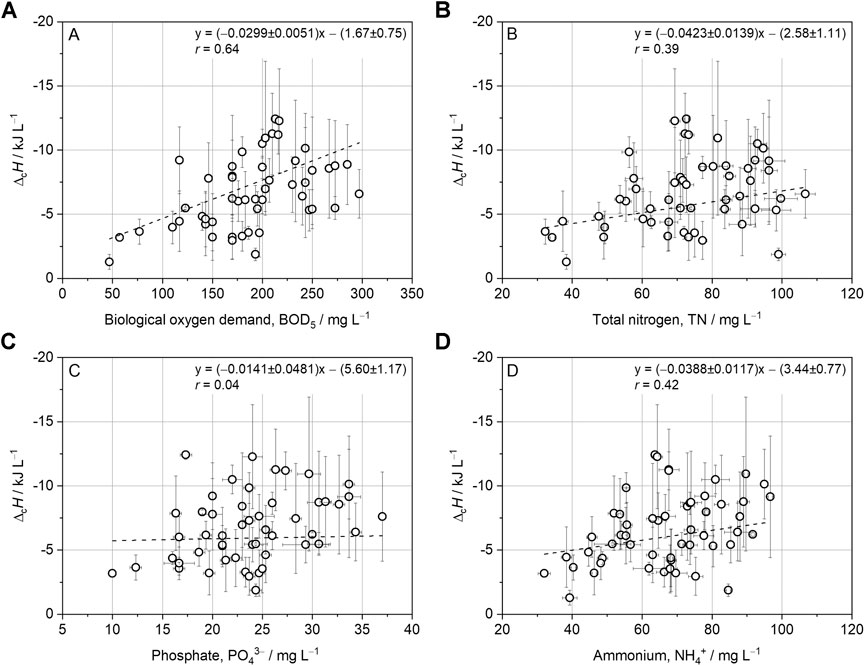
FIGURE 3. Linear regression analysis with combustion enthalpy (
Combining freeze-drying with micro-bomb combustion calorimetry to determine the chemical energy content of wastewater confirms previous results obtained with different but more laborious methodologies. Korth et al. reported −13.0 ± 1.6 kJ gCOD−1 for few samples by applying freeze-drying and conventional combustion calorimetry (Korth et al., 2017). By using centrifugal evaporation and conventional combustion calorimetry, Dai et al. found a correlation of −16.1 kJ gCOD−1 (Dai et al., 2019). As the correlations of −14.9 ± 3.5 kJ gCOD−1 and −13.3 ± 2.6 kJ gCOD−1 revealed here for composite and individual wastewater samples, respectively, are in good accordance with the literature, it can be concluded that micro-bomb combustion calorimetry is an appropriate tool for investigating the energy content of wastewater. This is also reflected by the COD recovery that is comparable to the literature (Supplementary Material S2). By decreasing the time needed for freeze-drying due to the smaller sample size required for micro-bomb combustion calorimetry, the COD loss is reduced. Nevertheless, the heterogeneity of wastewater samples and the requirement of adding benzoic acid for ensuring complete combustion resulted in a frequent scattering of
Interestingly, the BOD5 and
Considering the COD loss during freeze-drying (28.1 ± 5.9%, Supplementary Material S2), it can be assumed that the combustion enthalpy of wastewater and, consequently, the bioaccessible energy thereof is underestimated, even though the determined correlation factor of −14.9 ± 3.5 kJ gCOD−1 seems reasonable as it falls into the range of various carbon-based compounds (Heidrich et al., 2011; Dai et al., 2019). Considering the observed COD loss during sample treatment in this and prior studies, a correction factor of approximately 1.3 could be derived and applied to the COD–
Conclusion
The novel approach of using micro-bomb combustion calorimetry for analyzing the energy content of wastewater led to an improved sample treatment as only tiny amounts of samples were required for combustion enthalpy measurements. The obtained robust correlation between the combustion enthalpy and COD of wastewater of −14.9 ± 3.5 kJ gCOD−1 confirms the findings of previous studies that used more laborious and time-demanding sample preparation methods. The gathered data can be used for estimating the chemical energy potential of WWTPs treating low-strength wastewater, supporting efforts to transform WWTPs into sources for energy and material. Intriguingly, the global chemical energy potential is assumed to be higher as the first treatment steps of the WWTP and losses during sample preparation (i.e., freeze-drying) reduced the amount of energy-rich compounds before being measured by micro-bomb combustion calorimetry. Nevertheless, the so far restricted data foundation on thermodynamic parameters of wastewater for supporting efforts to engineer energy balances of WWTPs can be further extended, not only by the precious data set reported here.
Data Availability Statement
The original contributions presented in the study are included in the article/Supplementary Material; further inquiries can be directed to the corresponding author.
Author Contributions
BK: methodology, data analysis, and writing—original draft and editing. CH: micro-bomb combustion calorimetry and data analysis. MN-S: conceptualization, micro-bomb combustion calorimetry, and writing—review and editing. TM: conceptualization, methodology, and writing—review and editing. FH: conceptualization, methodology, supervision, project administration, and writing—review and editing.
Funding
This work was supported by the Helmholtz Association in the frame of the Integration Platform “Tapping nature’s potential for sustainable production and a healthy environment” at the UFZ.
Conflict of Interest
The authors declare that the research was conducted in the absence of any commercial or financial relationships that could be construed as a potential conflict of interest.
Acknowledgments
The authors acknowledge Abwasserzweckverband (AZV) für die Reinhaltung der Parthe for providing wastewater samples and the corresponding data sets and C. Dilssner for performing freeze-drying of wastewater and analysis of wastewater parameters. MN-S dedicates this work to Jerzy Drzazgowski from the University of Gdańsk, design engineer of the micro-bomb combustion calorimeter, in gratitude for thirty years of cooperation.
Supplementary Material
The Supplementary Material for this article can be found online at: https://www.frontiersin.org/articles/10.3389/fenrg.2021.705800/full#supplementary-material
References
Dai, Z., Heidrich, E. S., Dolfing, J., and Jarvis, A. P. (2019). Determination of the Relationship between the Energy Content of Municipal Wastewater and its Chemical Oxygen Demand. Environ. Sci. Technol. Lett. 6, 396–400. doi:10.1021/acs.estlett.9b00253
Drzazgowski, J., Jaworski, K., and Kosiń, A. (2018). Microbomb Combustion Calorimeter Type MK-1000.Technical Documentation. University of Gdansk, Institute of Oceanography, Gdynia.
Gordon, H. A. (1981). Errors in Computer Packages. Least Squares Regression through the Origin. The Statistician 30, 23–29. doi:10.2307/2987701
Hao, X., Li, J., van Loosdrecht, M. C. M., Jiang, H., and Liu, R. (2019). Energy Recovery from Wastewater: Heat over Organics. Water Res. 161, 74–77. doi:10.1016/j.watres.2019.05.106
Heidrich, E. S., Curtis, T. P., and Dolfing, J. (2011). Determination of the Internal Chemical Energy of Wastewater. Environ. Sci. Technol. 45, 827–832. doi:10.1021/es103058w
Henze, M., and Comeau, Y. (2008). “Wastewater Characterization,” in Biological Wastewater Treatment: Principles Modelling And Design. Editors M. Henze, M. C. M. van Loosdrecht, G. A. Ekama, and D. Brdjanovic (IWA Publishing)), 33–52.
Jones, E. R., Van Vliet, M. T. H., Qadir, M., and Bierkens, M. F. P. (2021). Country-level and Gridded Estimates of Wastewater Production, Collection, Treatment and Reuse. Earth Syst. Sci. Data 13, 237–254. doi:10.5194/essd-13-237-2021
Kehrein, P., van Loosdrecht, M. C. M., Dewulf, J., Garfi, M., and Posada Duque, J. A. (2020). A Critical Review of Resource Recovery from Municipal Wastewater Treatment Plants – Market Supply Potentials, Technologies and Bottlenecks. Environ. Sci. Water Res. Technol. 14, 112. doi:10.31729/jnma.1240
Koch, C., Popiel, D., and Harnisch, F. (2014). Functional Redundancy of Microbial Anodes Fed by Domestic Wastewater. ChemElectroChem 1, 1923–1931. doi:10.1002/celc.201402216
Korth, B., Maskow, T., Günther, S., and Harnisch, F. (2017). Estimating the Energy Content of Wastewater Using Combustion Calorimetry and Different Drying Processes. Front. Energ. Res. 5, 1–8. doi:10.3389/fenrg.2017.00023
Lamprecht, I. (1999). “Combustion Calorimetry,” in Handbook Of Thermal Analysis And Calorimetry, Volume 4: From Macromolecules To Man. Editor R. Kemp (Amsterdam: Elsevier Science B.V.), 175–218. doi:10.1016/s1573-4374(99)80007-7
McCarty, P. L., Bae, J., and Kim, J. (2011). Domestic Wastewater Treatment as a Net Energy Producer-Can This Be Achieved? Environ. Sci. Technol. 45, 7100–7106. doi:10.1021/es2014264
Metcalf & Eddy, Inc, , Eddy, I., Tchobanoglous, G., Stensel, H., Tsuchihashi, R., and Burton, F. (2014). Wastewater Engineering: Treatment and Resource Recovery. New York: McGraw-Hill Education.
Neugebauer, G., Kretschmer, F., Kollmann, R., Narodoslawsky, M., Ertl, T., and Stoeglehner, G. (2015). Mapping thermal Energy Resource Potentials from Wastewater Treatment Plants. Sustainability 7, 12988–13010. doi:10.3390/su71012988
Normant, M., Chrobak, M., and Szaniawska, A. (2002). Energy Value and Chemical Composition (CHN) of the Chinese Mitten Crab Eriocheir sinensis (Decapoda: Grapsidae) from the Baltic Sea. Thermochim. Acta 394, 233–237. doi:10.1016/S0040-6031(02)00259-9
Pons, M. N., Spanjers, H., Baetens, D., Nowak, O., Gillot, S., Nouwen, J., et al. (2004). Wastewater Characteristics in Europe - A Survey. European Water Management, 1–10.
Prus, T. (1975). “Calorimetry and Body Composition,” in Methods for Ecological Bioenergetics. IBP Handbook No. 24. Editors W. Grodzinski, R. Z. Klekowski, and A. Duncan (Oxford: Blackwell Scientific Publications), 149–160.
Scherson, Y. D., and Criddle, C. S. (2014). Recovery of Freshwater from Wastewater: Upgrading Process Configurations to Maximize Energy Recovery and Minimize Residuals. Environ. Sci. Technol. 48, 8420–8432. doi:10.1021/es501701s
Schröder, U., Harnisch, F., and Angenent, L. T. (2015). Microbial Electrochemistry and Technology: Terminology and Classification. Energy Environ. Sci. 8, 513–519. doi:10.1039/C4EE03359K
Shen, C., Lei, Z., Wang, Y., Zhang, C., and Yao, Y. (2018). A Review on the Current Research and Application of Wastewater Source Heat Pumps in China. Therm. Sci. Eng. Prog. 6, 140–156. doi:10.1016/j.tsep.2018.03.007
Shizas, I., and Bagley, D. (2004). Experimental Determination of Energy Content of Unknown Organics in Municipal Wastewater Streams. J. Energ. Eng. 130, 45–53. doi:10.1061/(ASCE)0733-9402(2004)130:2(45)
UN General Assembly (2015). Resolution 70/1: Tranforming Our World: The 2030 Agenda for Sustainable Development. New York. doi:10.1057/978-1-137-45443-0_24
Urbańczyk, E., Sowa, M., and Simka, W. (2016). Urea Removal from Aqueous Solutions—A Review. J. Appl. Electrochem. 46, 1011–1029. doi:10.1007/s10800-016-0993-6
U.S. Energy Information Administration (2021). International Electricity Consumption, Available at: https://www.eia.gov/international/data/world/electricity/electricity-consumption [Accessed June 7, 2021].
van der Hoek, J., Duijff, R., and Reinstra, O. (2018). Nitrogen Recovery from Wastewater: Possibilities, Competition with Other Resources, and Adaptation Pathways. Sustainability 10, 4605. doi:10.3390/su10124605
von Stockar, U. (2013). “Biothermodynamics of Live Cells: Energy Dissipation and Heat Generation in Cellular Cultures,” in In Biothermodynamics: The Role of Thermodynamics in Biochemical Engineering. Editors U. von Stockar, and L. A. M. van der Wielen (Lausanne: EPFL Press)), 475–534.
Vučić, V., Süring, C., Harms, H., Müller, S., and Günther, S. (2021). A Framework for P-Cycle Assessment in Wastewater Treatment Plants. Sci. Total Environ. 760, 143392. doi:10.1016/j.scitotenv.2020.143392
Wan, J., Gu, J., Zhao, Q., and Liu, Y. (2016). COD Capture: A Feasible Option towards Energy Self-Sufficient Domestic Wastewater Treatment. Sci. Rep. 6, 1–9. doi:10.1038/srep25054
World Nuclear Association (2021). Heat Values of Various Fuels. Available at: https://world-nuclear.org/information-library/facts-and-figures/heat-values-of-various-fuels.aspx [Accessed June 7, 2021].
Keywords: combustion calorimetry, wastewater, chemical oxygen demand, energy, circular economy
Citation: Korth B, Heber C, Normant-Saremba M, Maskow T and Harnisch F (2021) Precious Data from Tiny Samples: Revealing the Correlation Between Energy Content and the Chemical Oxygen Demand of Municipal Wastewater by Micro-Bomb Combustion Calorimetry. Front. Energy Res. 9:705800. doi: 10.3389/fenrg.2021.705800
Received: 06 May 2021; Accepted: 11 June 2021;
Published: 05 July 2021.
Edited by:
S. Venkata Mohan, Indian Institute of Chemical Technology (CSIR), IndiaReviewed by:
Naresh Kumar Amradi, Yonsei University, South KoreaAnish Ghimire, Kathmandu University, Nepal
Copyright © 2021 Korth, Heber, Normant-Saremba, Maskow and Harnisch. This is an open-access article distributed under the terms of the Creative Commons Attribution License (CC BY). The use, distribution or reproduction in other forums is permitted, provided the original author(s) and the copyright owner(s) are credited and that the original publication in this journal is cited, in accordance with accepted academic practice. No use, distribution or reproduction is permitted which does not comply with these terms.
*Correspondence: Benjamin Korth, YmVuamFtaW4ua29ydGhAdWZ6LmRl