- Department of Mechanical and Aerospace Engineering, University of Dayton, Dayton, OH, United States
Analyses used to reveal fuel dependencies on lean blow out and ignition at specific operating conditions in specific combustors show inconsistent trends with each other. Such variety is however consistent with the occurrence of transitions between the governing physical phenomena as the ratios between evaporation, mixing, or chemical time scales with their respective residence times also vary with specific operating conditions and combustor geometry. It is demonstrated here that the fuel dependencies on LBO in a large, single-cup, swirl-stabilized, rich-quench-lean combustor varies with operating conditions such that a feature importance match is attained to fuel dependencies observed in a much smaller combustor at one end of the tested range, while a qualitative match to fuel dependencies observed in a lean, premixed, swirler-stabilized combustor of comparable size at the other end of the tested range. The same reference combustor, when tested at cold conditions, is shown to exhibit similar fuel dependencies on ignition performance as the much smaller combustor, when tested at both cold and warm conditions. The practical significance of these findings is that a reference rig, such as the Referee Rig, can capture fuel performance trends of proprietary industry combustors by tailoring the inlet air and fuel temperatures of the tests. It is, therefore, a trustworthy surrogate for screening and evaluating sustainable aviation fuel candidates, reducing the dependency on proprietary industrial combustors for this purpose, thereby increasing transparency within the evaluation process while also expediting the process and reducing cost and fuel volume.
Introduction
As global fuel demand increases environmental, economic, and security interests have led to the investigation of sustainable aviation fuels (SAF) for wider use. Due to the differences in composition between SAF and petroleum-derived fuels, qualification of these fuels is required before implementation. The current process in place for the qualification of SAF, ASTM D4054, focuses on developing “drop-in” hydrocarbon fuels, meaning no changes need to be made to engine, aircraft, and airport infrastructure for a fuel to be compatible. Unless a candidate fuel qualifies for fast track approval, this evaluation is an extensive process that takes years to complete, millions of dollars and thousands of gallons of fuel (Oldani, 2020). As shown in Figure 1, the approval process for non-fast track jet fuel qualification involves four levels of testing as well as two stages of reports with comprehensive stakeholder review. Fuel is first tested for general specifications and fit-for-purpose properties before the phase 1 report is released to the stakeholders who complete a technical review of the data before it can proceed to tier 3 and tier 4 testing. Here, both rig and engine testing are completed. The amount of fuel required for testing increases about 10-fold with every tier in the qualification process.
Recently, a renewable jet fuel produced through catalytic hydrothermolysis referred to as RediJet was submitted to ASTM subcommittee J for aviation fuels for approval. As reported by Coppola (2018), approximately 72,000 gallons of SAF was required to complete the test plan. Component and rig tests were performed by three different engine manufacturers over nine different test conditions. Engine testing was completed by two engine manufacturers, including a flight test with a twin-engine Falcon 20. Three fuel mixtures were used for each test condition: neat Jet A as a baseline, neat RediJet, and a 50:50 blend. A total of 144,000 gallons of jet fuel was used for full qualification of the new “drop-in” SAF. Reducing the volume of fuel required for qualification would be advantageous for both fuel manufacturers and the sponsors who have a vested interest in SAF. The aim of the National Jet Fuel Combustion Program (NJFCP) was to shorten and redirect the process for jet fuel qualification (Colket et al., 2017). By developing predictive models for fuel behavior and adding some tailored, low-volume testing prior to the phase I research report, additional feedback to the ATSM evaluation committee and to fuel manufacturers would be provided to guide early fuel development. The work scope of tier 3 and tier 4 testing could then be directed toward a narrower range of potential concerns thereby reducing total fuel required. Alternatively, the candidate fuel might be reformulated into product that has a higher probability of achieving qualification. Importantly, there is a need to understand how fuel effects in small-scale rigs compare with engine observations. Validation of small-scale rigs against full-scale engines is essential for developing predictive models and testing methodology.
At the program level, a range of operating conditions were identified where lean blowout (LBO) or ignition is most likely to be impacted by differences in fuel composition and properties (Colket and Heyne, 2021). The most sensitive LBO conditions involve a throttle-chop at cruise to flight idle, and the start transient where the increase in fuel flow rate may not sufficiently keep up with the increase in airflow rate if the control schedule is improperly set for the fuel being used. Fuel impacts on ignition center on cold conditions, namely a cold-soaked auxiliary power unit (APU) at altitude or a cold-soaked main engine on the ground. The operating conditions are shown pictorially in Figure 2 for the typical temperatures (
Nine experimental rigs within the NJFCP, featuring a wide range of geometries and time scales were used to observe fuel effects (Colket and Heyne, 2021). As shown in Figure 3, eight of the nine rigs showed correlation between derived cetane number (DCN) and relative equivalence ratio at LBO (
Colborn et al. (2020) showed that the relative LBO at 65°C and 107 kPa air temperature and pressure in the RR is dominated by the Ohnesorge number (Oh) at 2% ΔP/P, while at 6% ΔP/P the DCN dominates, with a smooth transition from one extreme to the other. Also, at 3.5% ΔP/P and 107 kPa, the fuel with the lowest DCN and most favorable atomization properties (labeled as C1) showed no sensitivity to air temperature between 65°C and 83°C. Complementary to this data, Boehm et al. (2020) found this same fuel (C1) had measurably worse LBO performance in a GE9X combustor than the other three fuels tested at three of four test conditions. At a lower air temperature, C1 showed the same LBO performance as the reference petroleum-derived fuel when each were heated to 60°C, which was the reference fuel temperature for this set of tests. A summary of these results is presented in Figure 4. The data suggest that fuel physical and chemical properties are both important near the low temperature boundary of the GE9X engine operating range at conditions important to aircraft engine LBO margin, while only chemical properties are important at higher air temperature and loading.
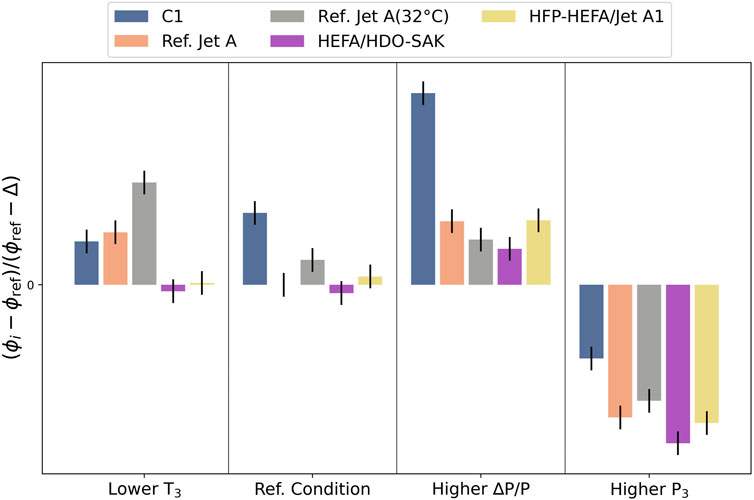
FIGURE 4. Relative LBO at four operating conditions in GE9X-FAR: Figure redrawn using digitally extracted data from GE report to FAA as part of the CLEEN II Consortium Program Update–Public Plenary.
In this report the results introduced above are shown to be consistent with LBO theory (Plee and Mellor, 1979; Mellor, 1980), and the RR, in concert with a well-thought-out test plan, is capable of showing the same fuel dependencies as the APU-CR and the GE9X-FAR. The timescales of evaporation and chemical reactions are impacted significantly by fuel and air temperature, suggesting the tested range of operating conditions is critical to a thorough investigation of fuel dependencies. We assert that it is not necessary to match commercial combustor geometry and operating conditions provided the test combustor is tested over a sufficiently wide range of operating conditions to sweep through the range of timescale ratios that are relevant to commercial combustor operability.
Background
Previous Work
Several investigations relating to fuel effects on LBO have been completed. Rock et al. (2019) measured the LBO threshold in an un-cooled flame tube of 18 different fuels and 3 different inlet air temperatures and noted correlation to DCN, T10, T90, or surface tension dependent on inlet air temperature. Casselberry et al. (2019) demonstrated correlation between pyrolysis products at 625°C and the LBO threshold in the RR when operated at chop-like (warm) conditions, using the same set of 18 fuels as Rock et al. An investigation into the role of preferential vaporization was conducted by Won et al. (2019), suggesting that DCN of the front end of the distillation may be a better indicator of LBO than DCN of the fully vaporized fuel, and they also observed that fuel physical properties are more strongly correlated with LBO than fuel chemistry at low temperature operation. Similarly, Grohmann et al. (2018) observed that both physical and chemical fuel properties influence combustor LBO. While focusing on the effects of atomization, Muthuselvan et al. (2020) related atomization quality with timescales relevant to LBO.
Many experiments and analyses of ignition characteristics of hydrocarbon fuels have focused on pre-vaporized and premixed fuel or other fuels and conditions that depart significantly from the most extreme start-up requirements for gas turbines used in aviation. Excellent reviews on the topic have been published by Aggarwal (1998) and more recently by Colket et al. (Colket and Heyne, 2021). Mayhew (2018) observed correlation between ignition probabilities at cold altitude relight conditions in a derivative of the RR and each of four fuel properties: viscosity, surface tension, T20, and flash point. Opacich et al. (2019) observed similar correlations within datasets derived from both the RR and the APU-CR, although vapor pressure and heat capacity were chosen instead of T20 and flash point to represent volatile properties. Part of this work is a direct follow-up of work introduced by Opacich et al.
LBO Theory
A common theme discussed implicitly or explicitly in several of the works cited above is that LBO performance can be evaluated by considering three timescales that impact LBO limits as shown in Equation 2: chemical, mixing and evaporative timescales (Plee and Mellor, 1979; Mellor, 1980). This theory is further illustrated in Figure 5.
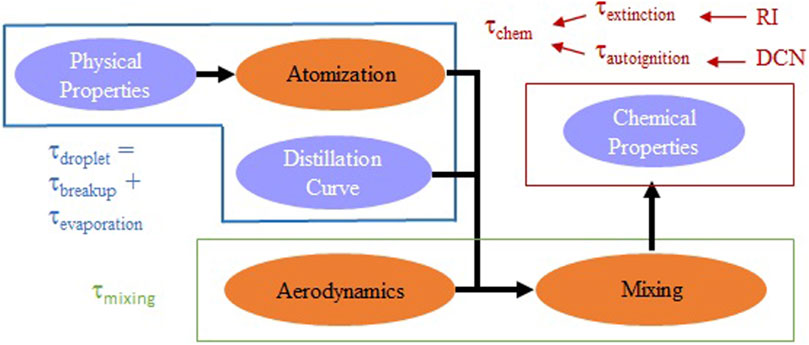
FIGURE 5. Available LBO pathways. Orange ovals represent combustor-specific characteristics and purple ovals show any fuel-dependent properties that can impact LBO limits.
Fuel physical properties along with aerodynamic shear forces, flow field, fuel nozzle design, and fuel pressure all impact fuel spray atomization: droplet size distribution and spray distribution. While combustor design and operating conditions are important to atomization, fuel properties are also an important factor for some commercial combustors at relevant, in-service operating conditions.
Fuel vapor pressure (and/or thermal conductivity), spray characteristics, and combustor aerodynamics all influence the evaporation timescale. From the perspective of fuel dependencies on LBO, it is important to note the evaporation timescale of some commercial combustors will be impacted significantly by vapor pressure, which varies not only with droplet surface temperature but also with the time-varying composition of the liquid fuel throughout the evaporation process. For systems that are evaporation limited, it is expected that fuels with a higher vapor pressure at a given temperature would ignite more readily than a fuel with lower vapor pressure.
The mixing of fuel vapor with air depends on the flow field, turbulence intensity, and the spatial relationship between the fuel spray, the eddies within the flow field, and the flame. Because turbulence is overwhelmingly more important than laminar diffusion in most industry combustors, there is ample technical justification for neglecting this term when considering fuel effects. Moreover, the characteristic mixing time of a given commercial combustor at any well-defined operating condition is likely to be held as proprietary by the engine companies.
The details around fuel-air mixing influence the gaseous mixture residence time and reactant concentration which, along with species reactivity, determine the fuel chemistry of combustion and blowout. The chemical timescale is relevant to this physics and may be comprised of different pieces such as autoignition and extinction.
Cold Ignition Overview
At extreme low fuel temperatures, fuel vapor pressure is low and with equally low inlet air temperature, no heating of droplets occurs until they reach a heat source, which could be a plasma discharge or the kernel of a previously ignited fuel/air mixture. At the extreme cold condition, the size and spatial distribution of the liquid fuel droplets within the combustor flow field is expected to be critical for most if not all combustors in aviation service. Very little evaporation occurs outside of the domain of the plasma discharge (spark), therefore it must supply enough energy to both evaporate the fuel and overcome the critical kernel radius (Kim et al., 2013). The heat released by each kernel must be high enough to both sustain the flame and sufficiently evaporate enough surrounding liquid fuel droplets to replenish the fuel consumed by the combustion that is occurring inside it. Only then can the flame kernel grow and potentially propagate upstream to an anchor point, transitioning to a self-sustaining flame. This process can be influenced significantly by fuel volatility, thermal properties and the physical properties that influence atomization.
Atomization Overview
Atomization will be affected by the viscosity, density, and surface tension (Guildenbecher et al., 2009; Lefebvre and McDonell, 2017). Increasing the surface tension will inhibit fuel breakup, while increasing the viscosity will dampen instabilities that allow for breakup, and increased density drives lower flow velocities in engines that are controlled to deliver a scheduled enthalpy flux or equivalence ratio. This in turn reduces the gage pressure that supplies the energy driving atomization.
Experiments, Data and Methods
Referee Rig Experiments
Experiments performed in the RR were completed at the Air Force Research Laboratory located at Wright-Patterson Air Force Base, and have been published elsewhere (Hendershott et al., 2018; Colborn et al., 2020). The Referee Rig is a non-proprietarty, single-cup, swirl-stabilized combustor designed by GE (this article’s correspondance author) with input from four other leading engine manufacturers to simulate representative aerodynamic characteristics of both legacy and emerging, swirl-stabilized, combustors (Colket and Heyne, 2021). It is a classic rich-quench-lean, combustor with effusion cooled liners, a flat dome protected by an impingement-cooled heat shield, primary dilution holes located ½ dome height downstream from the dome and seconday dilution holes located just aft of the primary reaction zone. It features a modular construction to facilitate swapping of fuel injectors and swirlers to allow for evalation of different swiler effective areas, swirl numbers, spray angles and flow numbers. However, most of the data collected off this combustor so far has been with just one design configuration. Modification from it’s original 4-cup design to a single cup design was completed by AFRL, and UDRI custom-buillt a thryatron-based exciter to achieve better control over spark energy and frequency relative to jet engine exciters. Readers interested in fabricating a copy of this combustor should contact the author for leads on where to find a copy of the drawings.
The four operating conditions analyzed in this work are as listed in Table 1. Fuel and air temperature were matched in each case, and LBO was determined after each successful ignition at
APU-CR Experiments
The APU-CR experiments were performed at Honeywell Aerospace, in their combustor component test facility, and was operated at simulated engine conditions (Culbertson and Williams, 2017). APUs are small gas turbine engines that are used to provide power to spool-up the main engine during starter-assisted air starts. APUs are particularly sensitive to the physical properties that influence atomization and vaporization (Peiffer et al., 2019) because of their small volume, and corresponding low combustor residence time (τcmb= ρairVcmb/Wair). The 131-9 combustor is swirl-stabilized and relies on a rich-quench-lean combustion process, like many of the much-larger, main engine combustors. A standard 131-9 ignition system was used with the igniter located at approximately the eight o’clock position of the combustor (Culbertson and Williams, 2017). Readers who wish to reproduce any of the data presented in the noted publications should contact Honeywell Aerospace.
The warm ignition (Tfuel = 15°C) light off boundary was determined at a baseline air temperature (−35°C) and pressure (1.05 atm) along with single point derivatives to higher temperature or lower pressure, as listed in Table 1. The cold ignition (Tfuel = −37°C) light off boundary was determined at each of the conditions used for warm ignition plus two additional points at a somewhat colder air temperature and low pressure, also listed in Table 1, and the lean blowout dataset included six operating conditions. For all test conditions, the analyzed equivalence ratios were normalized by Equation 1, just as was done with the RR data.
GE9X-FAR Experiments
The GE9X-FAR experiments were performed at GE, in their combustor component test facility, and was operated at simulated engine conditions, which are proprietary. However, a public release of sanitized data is available through reference (Boehm et al., 2020), and readers who wish to reproduce this data should contact GE. Quite unlike the RR and the APU-CR, the GE9X is a large combustor which achieves lean combustion for low NOx emissions with its twin annular premixing swirler. Limited details about the this combustor design have been published by Dhanuka et al. (2011). The understandable restrictions around sharing test data, procedures, and combustor design relating to fuel evaluation tests such as these was and is one of the prime motivators behind the development of the RR.
The GE data was not presented in a format that could be included in the statistical analyses that are reported in this work. The LBO data shown in Figure 4 was normalized at the baseline operating condition by an equation like Equation 4, but it was not reset at each operating condition because dependence on operating condition was part of the story GE communicated. The un-disclosed constant denoted by
Fuel Property Data
The RR and APU-CR experiments were directly or indirectly part of the NJFCP and used fuels that were distributed to affiliated labs by a control center, led by Tim Edwards at the Air Force Research Laboratory, who was also responsible for acquiring and publishing fuel property data (Edwards, 2017). This data is also available through the National Alternative Jet Fuels Test Database (Home | AJF:TD | U of I, 2021). The fuel samples designated as A1, A2, A3, C1, C2, and C5 were tested in both rigs. Additionally, the samples designated as C3, C4, and C7 were also tested in the RR. The GE9X experiments occurred under a different program but did include one fuel (C1) which was provided by Tim Edwards. Documentation of the property data of the fuels used by GE is provided in Table 2.
The fuel densities used in the analyses of the LBO datasets were as measured at 15°C. For analyses of the ignition datasets, all fuel properties were transformed into their respective values at the tested fuel temperature by the approach previously described by Opacich et al. (2019). Fuel properties that were measured over a range of temperatures that bounded the tested fuel temperature were interpolated to the test temperature (e.g., density). Temperature-dependent fuel properties that were not measured over a sufficient range to warrant interpolation (e.g., vapor pressure) were determined as outlined here. First, a surrogate fuel composition was derived by match to measured fuel property data and GCxGC-determined hydrocarbon class concentration data, using published blending rules (Flora et al., 2019) to relate molecular properties and compositions to mixture properties. Next the molecular properties over a range of temperatures were calculated based on the models provided in the molecular properties database published by the National Institute of Standards and Technology (Kroenlein et al., 2012), and the blending rules applied at each modeled temperature. The resulting temperature-dependent mixture properties were then curve-fitted and those models were used to estimate the fuel properties at each, tested fuel temperature.
Analysis
The random forest statistical analysis approach used in this work has been described previously (Peiffer et al., 2019; Colborn et al., 2020). In summary, the method employs random sampling and replacement to decrease overfitting and allows for one dependent variable (e.g., LBO or ignition performance) to be evaluated against multiple independent variables (e.g., fuel properties) (Hastie et al., 2008). Standard Monte Carlo methods were used to simulate uncertainties in each independent variable based on measurement reproducibility as quoted in the relevant ASTM standard with an assumed Gaussian distribution, and these distributions represent the uncertainty domain within the random forest method. The regression approach taken was the same as that described by Opacich et al. (2019) and Peiffer et al. (2019). The simulation includes many trials to capture the full distribution of possible values within the reproducibility domain of each measured value. Each time, the relative importance values of each independent variable is recorded. In this way confidence bands around each relative importance value are estimated.
One set of random forest analyses was used here to assess the relative importance of atomization, evaporation rate, autoignition and extinction in each of two LBO datasets. Since none of these fundamental processes were clearly known or regress-able for all the fuels used in both test articles, it was necessary to choose a set of four independent/orthogonal properties that are known to correlate strongly with each of these four fundamental processes. Primary and secondary droplet breakup at incipient LBO conditions was represented by density at 15°C. T20 was selected to represent evaporation rate. Extinction was represented by radical index (RI), and autoignition was represented by derived cetane number. The idea was to use a comparison of these two analyses to assess how well one dataset, LBO in the RR at cold conditions, represents another dataset, LBO in the APU-CR at normal operating conditions.
Another set of random forest analyses was used to assess the relative importance of three independent variables in each of three cold ignition datasets. The Ohnesorge Number, which combines dynamic viscosity (
represent the atomization dependencies. The fuel dependency on evaporation rate was represented by vapor pressure, and specific heat was used to represent the fuel dependency on droplet heating. The definition of the dependent variable, representing ignition performance is somewhat different between the RR dataset and the APU-CR datasets. For the APU-CR datasets the ignition variable was defined by the minimum equivalence ratio required to achieve ignition within a Honeywell-standard duration of time during which the ignitor is firing periodically as it would in a commercial APU. For the RR dataset the ignition variable was defined by the equivalence ratio corresponding to 10% ignition probability per spark along a binomial regression fitted curve to the equivalence ratio and light/no-light data corresponding to each spark. Details of the binomial regression have been published by Hendershot et al. (2018).
Results
LBO Results
While several laboratory rigs show a strong correlation between LBO and DCN (Figure 3), the APU-CR does not. Instead, it shows a strong correlation to physical and volatility properties such as viscosity (
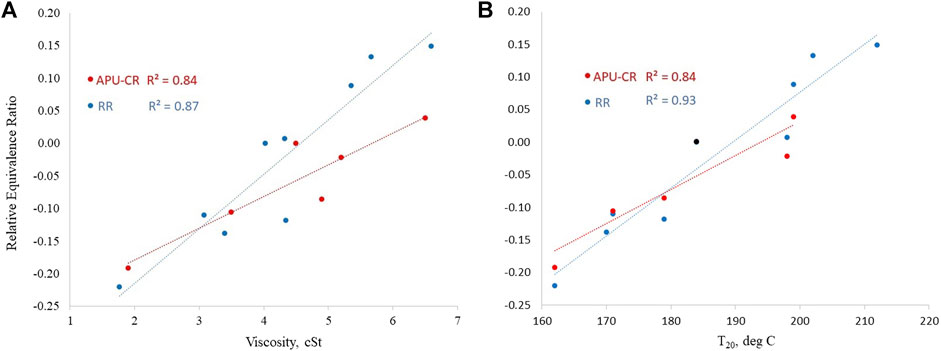
FIGURE 6. APU-CR & RR LBO performance correlation with (A) viscosity (
Main effects plots of Φ versus fuel property, as represented by Figure 6, suggest that both rigs show a correlation to fuel physical properties when the time scale of evaporation is on the same order as the combustor residence time. To further analyze this property dependency, a random forest statistical analysis was performed 1000 times, and a summary of these results is shown in Figure 7.
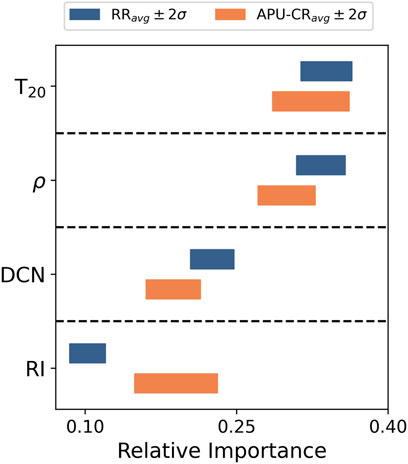
FIGURE 7. LBO determinants importance values for the RR at cold conditions and the APU-CR at normal operating conditions. On average, 98.6% of the LBO performance variance in the RR is explained by the chosen independent variables, while 91.8% of the LBO performance variance in the APU-CR is explained. RR results are shown above APU-CR results in each block.
One important result of this analysis is that each rig shows nearly the same relative importance of T20 (representing evaporation rate) and density (representing atomization) on LBO, which suggests that the RR, when operated at cold conditions, does represent the relevant physics that largely determine LBO performance in the APU-CR operating at representative engine conditions. Another important result is that fuel properties that influence evaporation rate are clearly more important than those that correlate strongly with chemical reactivity. This result suggests that the LBO performance of these two rigs, as operated in these tests, is affected by evaporation more than chemical reactivity, so the data collected in this way should be used to evaluate the impact of fuel physical property variation on LBO. The third important result is that the relative importance of the radical index in the RR at these conditions does not match those of the APU-CR, suggesting that the RR is not a good surrogate for the APU-CR in this context. However, that may not be a requirement since radical index has less impact on LBO than the other properties considered. In contrast, the LBO performance of the GE9X-FAR is more strongly determined by the fuel chemistry properties so a useful surrogate laboratory combustor and test condition for it should reproduce similar values of the chemical property influence factors.
Turning now to the data from the GE9X-FAR, the notable differences, documented in Table 2, between the reference petroleum-derived fuel and the SAF blend component, designated as C1, are as follows. Sample C1 is 6.3% lighter, has 1.7% higher specific energy and has a much lower DCN. The lower density and higher specific energy of C1 are expected to affect LBO toward a lower (more favorable) ϕC1 because lower density leads to a higher volumetric flow rate which leads to higher fuel pressure and therefore finer atomization and the higher LHV leads to a higher flame temperature for a given equivalence ratio. Conversely, the lower DCN of C1 is expected to lead to a higher (less favorable) ϕC1 based on the empirical trends shown in Figure 3. The data shows higher ϕC1 at three of the four test conditions, which is consistent with the much lower DCN of C1 relative to the reference fuel. At the lowest air temperature condition, however there is essentially no difference between ϕC1 and ϕref which is likely the result of the favorable density and specific energy of C1 compensating for its unfavorable DCN. GE also provided LBO data for Jet A fuel at two different temperatures. While this is not the same fuel, it is from the same supplier, and it is reasonable to assume that the properties of each are comparable, if not similar. The colder fuel will have higher density, viscosity, and surface tension and lower initial vapor pressure, but the chemical properties of the fuel vapor are the same. These property differences are reflected in the data: at the lowest air temperature condition the ϕref,@32C is higher than ϕref,@60C. At each of the three conditions where C1 shows measurably worse LBO performance than the reference fuel, the colder reference fuel shows no clear difference compared to the warmer reference fuel. Together, these trends suggest that the LBO phenomenon in the GE9X-FAR is governed by chemistry at three of the four test conditions, but when the air temperature is reduced, evaporation becomes important as well. The two SAF fuels that are derived in part from hydrogenated esters and fatty acid (HEFA) show similar results to each other at all conditions, and an improvement relative to the reference fuel at the lowest temperature test condition, as expected based on their lower viscosity and lower density relative to the reference fuel.
Ignition Results
Main effects plots of Φ versus fuel property, as represented by Figure 8, suggest that both rigs show a correlation to fuel physical properties (viscosity) and volatile properties (T20). To further analyze this property dependency, a random forest statistical analysis was performed 1,000 times, and a summary of these results is shown in Figure 9. As noted in Referee Rig Experiments section,
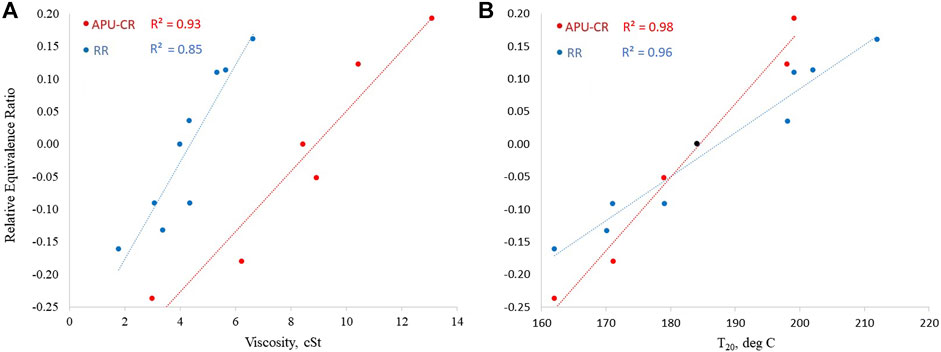
FIGURE 8. RR and APU-CR ignition equivalence ratio as a function of (A) Viscosity and (B) 20% recovered temperature (
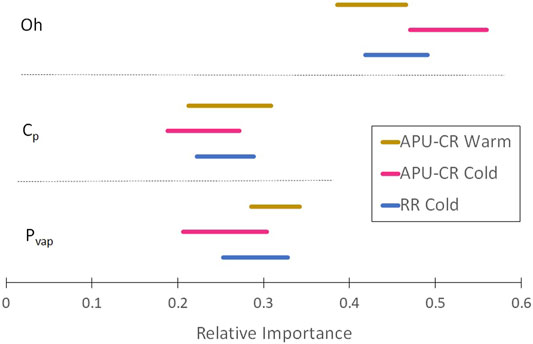
FIGURE 9. Ignition determinants importance values for the RR at cold conditions and the APU-CR at both cold and warm conditions. On average, 94% of the ignition performance variance in the RR is explained by the chosen independent variables, 96% of the ignition performance variance in the APU-CR at cold conditions is explained, and 95% of the ignition performance variance in the APU-CR at warm conditions is explained. The display order from top to bottom in each block is the same as is shown in the legend.
Each of the three selected fuel properties were shown be of similar importance in all three datasets: the RR at cold conditions and in the APU-CR at both cold and warm conditions. The Ohnesorge number accounts for about 46% of the observed variation in ignition performance, while vapor pressure accounts for 29% and specific heat accounts for 25%. In each dataset, these 3 variables alone explain 94–96% of the variation across the whole dataset which is especially interesting given the range of air temperature, pressure, or velocities that were tested. A small disconnect between the relative importance of the Ohnesorge number on ignition performance in the APU-CR with cold fuel relative to warm fuel is consistent with expectations based on visual observation of sprays at similar conditions in a benchtop inspection, where the cold fuel produces visually observable coarser spray.
The main point illuminated by this analysis is that the fuel property dependencies within each of the three datasets are nearly the same, which suggests that it is possible to use a small, standardized set of test articles to characterize fuel dependencies on ignition within the industry-wide fleet of combustors, which has important practical implication for the evaluation of potential SAF’s. From a more fundamental perspective it is an interesting observation that two fuel properties are required to account for the evaporation timescale. This observation suggests that more detailed data relating to fundamental heat and mass transfer processes within the intersecting region of cold fuel droplets and plasmas or pre-existing flame kernels could lead to an even better understanding of the fundamental processes that govern fuel property dependencies on kernel initiation and growth.
Conclusion
In this work it has been suggested that combustor operating conditions can be used to vary the relative importance of the evaporation, chemical, and mixing timescales that are characteristics of combustion phenomenon. By adjusting the operating conditions of the LBO experiments the ratio of the evaporation time scale to residence time can be matched between to combustors with vastly different length scales, the so-called Referee Rig and the Honeywell 131-9 APU combustor rig (APU-CR). It has been demonstrated that the RR, when operated at cold fuel and air conditions, exhibits the same fuel property dependencies (density and the temperature corresponding to 20% distilled) on lean blowout as the APU-CR at normal operating conditions. Further, when operated at representative flight idle conditions, the RR exhibits the same LBO dependencies on fuel properties (derived cetane number) as the GE9X full annular rig (GE9X-FAR) at similar operating conditions. Moreover, it has been observed that when the GE9X-FAR is operated at lower temperature, the LBO phenomenon is not governed primarily by derived cetane number, but rather by a combination of chemical and physical fuel properties, consistent with previous work (Colborn et al., 2020) probing the transition in operating conditions space, between evaporation-governed LBO and chemistry-governed LBO in the RR.
Analysis of data pertaining to the fuel dependencies on cold ignition in the RR as well as both standard-day and cold ignition in the APU-CR shows that atomization and evaporation are equally important to ignition performance. The atomization time scale is represented well by the Ohnesorge number, while the evaporation timescale is represented well by specific heat and vapor pressure. The correlations suggest that evaporation rate, under the conditions of the three sets of experiments, is determined by heat absorption (represented by specific heat) and the response to heat absorption (represented by the initial vapor pressure) with equal weighting. Most importantly, from a practical perspective, such fuel dependencies are shown to be common across a large difference in combustor cup volume or the operating environment.
Together these results indicate that the RR shows a great deal of correlation to real engines with respect to gaging the fuel dependencies of combustor operability, and thus shows potential as a standard, laboratory-scale test article to represent swirl-stabilized combustors in the ASTM fuel evaluation process for sustainable aviation fuels.
Data Availability Statement
The raw data supporting the conclusions of this article are provided in the supplementary material or should be requested from the original source, as cited.
Author Contributions
RB rewrote the original draft, brought in data and discussion around Figure 4 and Table 2, added about 20 references and cleaned up the rest. RB also provided conceptualization. JC wrote the original draft, brought in the data around Table 1 and Figures 3, 6, 8, and performed the statistical analyses JH provided conceptualization, funding and manuscript review and editing.
Funding
This research was funded by the U.S. Federal Aviation Administration Office of Environment and Energy through ASCENT, the FAA Center of Excellence for Alternative Jet Fuels and the Environment, project 34 through FAA Award Number 13-C-AJFE-UD-024 under the supervision of Anna Oldani. Any opinions, findings, conclusions or recommendations expressed in this material are those of the authors and do not necessarily reflect the views of the FAA. Open access publication fees are provided by the University of Dayton.
Conflict of Interest
The authors declare that the research was conducted in the absence of any commercial or financial relationships that could be construed as a potential conflict of interest.
Publisher’s Note
All claims expressed in this article are solely those of the authors and do not necessarily represent those of their affiliated organizations, or those of the publisher, the editors and the reviewers. Any product that may be evaluated in this article, or claim that may be made by its manufacturer, is not guaranteed or endorsed by the publisher.
Acknowledgments
The authors acknowledge support and helpful technical discussions with Tim Edwards and Edwin Corporan of the Air Force Research Laboratory as well as Scott Stouffer and Tyler Hendershott of the University of Dayton Research Institute.
Supplementary Material
The Supplementary Material for this article can be found online at: https://www.frontiersin.org/articles/10.3389/fenrg.2021.701901/full#supplementary-material
References
Aggarwal, S. K. (1998). A Review of spray Ignition Phenomena: Present Status and Future Research. Prog. Energ. Combustion Sci. 24 (6), 565–600. doi:10.1016/S0360-1285(98)00016-1
Boehm, R., Thomsen, D. D., and Andac, M. G. (2020). GE-aviation Program Update - Public Plenary. Cincinnati, OH: FAA CLEEN II Consortium.
Casselberry, R. Q., Corporan, E., and DeWitt, M. J. (2019). Correlation of Combustor Lean Blowout Performance to Supercritical Pyrolysis Products. Fuel 252, 504–511. doi:10.1016/j.fuel.2019.04.128
Colborn, J. G., Heyne, J. S., Stouffer, S. D., Hendershott, T. H., and Corporan, E. (2020). Chemical and Physical Effects on Lean Blowout in a Swirl-Stabilized Single-Cup Combustor. Proceedings of the Combustion Institute 38 (4):6309-6316. doi:10.1016/j.proci.2020.06.119
Colket, M., and Heyne, J. (2021). Fuel Effects on Operability of Aircraft Gas Turbine Combustors. AIAA, Prog. Astronautics Aeronautics 262submitted in proofing. doi:10.2514/4.106040
Colket, M., Heyne, J., Rumizen, M., Gupta, M., Edwards, T., Roquemore, W. M., et al. (2017). Overview of the National Jet Fuels Combustion Program. AIAA J. 55 (4), 1087–1104. doi:10.2514/1.J055361
Coppola, E. N. (2018). CHJ Pathway ReadiJet Renewable Jet Fuel Produced by Catalytic Hydrothermolysis (CH). ASTM Committee D02 on Petroleum Products and Lubricants, Subcommittee D02.J0.06 on Emerging Turbine Fuels.
Culbertson, B., and Williams, R. (2017). Alternative Aviation Fuels for Use in Military APUs and Engines, Versitile Affordable Advanced Turbine Engine (VAATE), Phase II and III, Delivery Order 0007 : AFRL-RQ-WP-TR-2017-0047.
Dhanuka, S. K., Temme, J. E., and Driscoll, J. F. (2011). Unsteady Aspects of Lean Premixed Prevaporized Gas Turbine Combustors: Flame-Flame Interactions. J. Propulsion Power 27 (3), 631–641. doi:10.2514/1.B34001
Edwards, J. T. (2017). Reference Jet Fuels for Combustion Testing. In:55th AIAA Aerospace Sciences Meeting. Grapevine, TX, Reston, VA: AIAA. doi:10.2514/6.2017-0146
Flora, G., Kosir, S. T., Behnke, L., Stachler, R. D., Heyne, J. S., Zabarnick, S., et al. (2019). Properties Calculator and Optimization for Drop-In Alternative Jet Fuel Blends, AIAA Scitech 2019 Forum. doi:10.2514/6.2019-2368
Grohmann, J., Rauch, B., Kathrotia, T., Meier, W., and Aigner, M. (2018). Influence of Single-Component Fuels on Gas-Turbine Model Combustor Lean Blowout. J. Propulsion Power 34 (1), 97–107. doi:10.2514/1.B36456
Guildenbecher, D. R., López-Rivera, C., and Sojka, P. E. (2009). Secondary Atomization. Exp. Fluids 46, 371–402. doi:10.1007/s00348-008-0593-2
Hastie, T., Tibshirani, R., and Friedman, J. (2008). The Elements of Statistical Learning, Data Mining, Inference, and Prediction. 2nd Editio. Stanford, California: Springer.
Hendershott, T. H., Stouffer, S., Monfort, J. R., Diemer, J., Busby, K., Corporan, E., et al. (2018). Ignition of Conventional and Alternative Fuel at Low Temperatures in a Single-Cup Swirl-Stabilized Combustor. In: AIAA Aerospace Sciences Meeting. Kissimmee, Florida: American Institute of Aeronautics and Astronautics, Inc. doi:10.2514/6.2018-1422
Home | AJF:TD | U of I n.D. (2021). Available at: https://altjetfuels.illinois.edu/. (Accessed Jul 5, 2021).
Kim, H. H., Won, S. H., Santner, J., Chen, Z., and Ju, Y. (2013). Measurements of the Critical Initiation Radius and Unsteady Propagation of N-Decane/air Premixed Flames. Proc. Combustion Inst. 34 (1), 929–936. doi:10.1016/j.proci.2012.07.035
Kroenlein, K., Muzny, C., Kazakov, A., Diky, V., Chirico, R., and Magee, J. (2012). NIST Standard Reference 203: TRC Web Thermo Tables (WTT) [Internet]. Gaithersburg. MD: National Institutes of Standards and Technology n. d., 1.
Lefebvre, A. H., and McDonell, V. G. (2017). Atomization and Sprays. 2nd ed. Boca Raton, Florida: CRC Press Taylor & Francis Group.
Mayhew, E. K. (2018). Impact of Alternative Jet Fuels on Gas Turbine Combustion Systems. Dissertation for the Doctoral Degree. Urbana-Champaign: University of Illinois at.
Mellor, A. M. (1980). Semi-empirical Correlations for Gas Turbine Emissions, Ignition, and Flame Stabilization. Prog. Energ. Combustion Sci. 6 (4), 347–358. doi:10.1016/0360-1285(80)90010-6
Muthuselvan, G., Suryanarayana Rao, M., Iyengar, V. S., Pulumathi, M., Thirumalachari, S., and K, S. (2020). Effect of Atomization Quality on Lean Blow-Out Limits and Acoustic Oscillations in a Swirl Stabilized Burner. Combustion Sci. Techn. 192 (6), 1028–1052. doi:10.1080/00102202.2019.1607846
Opacich, K. C., Heyne, J. S., Peiffer, E., and Stouffer, S. D. (2019). Analyzing the Relative Impact of Spray and Volatile Fuel Properties on Gas Turbine Combustor Ignition in Multiple Rig Geometries. In: AIAA Scitech 2019 Forum. San Diego: California. doi:10.2514/6.2019-1434
Peiffer, E. E., Heyne, J. S., and Colket, M. (2019). Sustainable Aviation Fuels Approval Streamlining: Auxiliary Power Unit Lean Blowout Testing. AIAA J. 57 (11), 4854–4862. doi:10.2514/1.j058348
Plee, S., and Mellor, A. (1979). Characteristic Time Correlation for Lean Blowoff of bluff-body-stabilized Flames☆. Combustion and Flame 35, 61–80. doi:10.1016/0010-2180(79)90007-5
Rock, N., Chterev, I., Emerson, B., Won, S. H., Seitzman, J., and Lieuwen, T. (2019). Liquid Fuel Property Effects on Lean Blowout in an Aircraft Relevant Combustor. J. Eng. Gas Turbines Power 141 (7), 071005–071017. doi:10.1115/1.4042010
Stouffer, S. D., Hendershott, T. H., Colborn, J., Monfort, J. R., Corporan, E., Wrzesinski, P., et al. (2020). Fuel Effects on Altitude Relight Performance of a Swirl Cup Combustor. In: AIAA Scitech 2020 Forum. Reston, VA: AIAA. doi:10.2514/6.2020-1882
Won, S. H., Rock, N., Lim, S. J., Nates, S., Carpenter, D., Emerson, B., et al. (2019). Preferential Vaporization Impacts on Lean Blow-Out of Liquid Fueled Combustors. Combustion and Flame 205, 295–304. doi:10.1016/j.combustflame.2019.04.008
Nomenclature
D = characteristic diameter
Oh = Ohnesorge number
T20 = 20% recovered temperature
Tfuel = fuel temperature
W = mass flow rate
τcmb = combustor residence time
APU = auxiliary power unit
APU-CR = honeywell 131-9 APU combustor rig
ASTM = ASTM international
A2 = reference fuel, single source
C1 = jet fuel blend component, ASTM D7755, annex A5
DCN = derived cetane number
FAA = federal aviation administration
GE9X-FAR = GE9X full annular combustor rig
LBO = lean blowout
NJFCP = national jet fuel combustion program
RI = radical index
RR = referee rig
SAF = sustainable aviation fuel
Keywords: lean blowout, ignition, fuel dependencies, sustainable aviation fuel, jet fuel, rig to engine correlation
Citation: Boehm RC, Colborn JG and Heyne JS (2021) Comparing Alternative Jet Fuel Dependencies Between Combustors of Different Size and Mixing Approaches. Front. Energy Res. 9:701901. doi: 10.3389/fenrg.2021.701901
Received: 28 April 2021; Accepted: 29 July 2021;
Published: 13 August 2021.
Edited by:
Nathan Brown, Federal Aviation Administration, United StatesReviewed by:
Muhammad Farooq, University of Engineering and Technology, Lahore, PakistanSen Li, Institute of Mechanics (CAS), China
Copyright © 2021 Boehm, Colborn and Heyne. This is an open-access article distributed under the terms of the Creative Commons Attribution License (CC BY). The use, distribution or reproduction in other forums is permitted, provided the original author(s) and the copyright owner(s) are credited and that the original publication in this journal is cited, in accordance with accepted academic practice. No use, distribution or reproduction is permitted which does not comply with these terms.
*Correspondence: Randall C. Boehm, cmJvZWhtMUB1ZGF5dG9uLmVkdQ==