- 1Laboratory of Chemistry and Electrochemistry of Solids, Department of Chemistry, Université de Montréal, Montréal, QC, Canada
- 2Institute of Condensed Matter and Nanosciences, Université Catholique de Louvain, Louvain-la-Neuve, Belgium
- 3Thayer School of Engineering, Dartmouth College, Hanover, NH, United States
All-Solid-State Lithium Batteries (ASSLBs) are promising since they may enable the use of high potential materials as positive electrode and lithium metal as negative electrode. This is only possible through solid electrolytes (SEs) stated large electrochemical stability window (ESW). Nevertheless, reported values for these ESWs are very divergent in the literature. Establishing a robust procedure to accurately determine SEs’ ESWs has therefore become crucial. Our work focuses on bringing together theoretical results and an original experimental set up to assess the electrochemical stability window of the two NASICON-type SEs Li1.3Al0.3Ti1.7(PO4)3 (LATP) and Li1.5Al0.5Ge1.5(PO4)3 (LAGP). Using first principles, we computed thermodynamic ESWs for LATP and LAGP and their decomposition products upon redox potentials. The experimental set-up consists of a sintered stack of a thin SE layer and a SE-Au composite electrode to allow a large contact surface between SE and conductive gold particles, which maximizes the redox currents. Using Potentiostatic Intermittent Titration Technique (PITT) measurements, we were able to accurately determine the ESW of LATP and LAGP solid electrolytes. They are found to be [2.65–4.6 V] and [1.85–4.9 V] for LATP and LAGP respectively. Finally, we attempted to characterize the decomposition products of both materials upon oxidation. The use of an O2 sensor coupled to the electrochemical setup enabled us to observe operando the production of O2 upon LAGP and LATP oxidations, in agreement with first-principles calculations. Transmission Electron Microscopy (TEM) allowed to observe the presence of an amorphous phase at the interface between the gold particles and LAGP after oxidation. Electrochemical Impedance Spectroscopy (EIS) measurements confirmed that the resulting phase increased the total resistance of LAGP. This work aims at providing a method for an accurate determination of ESWs, considered a key parameter to a successful material selection for ASSLBs.
Introduction
Since their commercialization in 1990, rechargeable lithium-ion batteries (LIBs) have revolutionized global communication and enabled the democratization of portable electronics. LIBs have grown to become a well-established, efficient energy-storage technology in terms of power density and life span. However, commercialized LIBs contain highly flammable and toxic organic liquids as electrolyte (LiPF6 in carbonate-based solvents), entailing significant safety hazard. Moreover, currently used liquid electrolytes hinder the use of lithium metal as a negative electrode material and the commercialization of high-energy-density Li-metal battery systems (Aurbach et al., 2002; Lin et al., 2017). Lithium metal displays a remarkably high theoretical capacity and is considered the best negative electrode material for lithium batteries (3,860 mA h.g−1, −3.05 V vs. SHE) (Winter and Besenhard, 1999). Nevertheless, lithium metal batteries fail to achieve commercialization with conventional carbonate-based liquid electrolytes because of lithium metal’s low cycling efficiency and its detrimental formation of lithium dendrites (Orsini et al., 1998). Upon cycling, the growth of dendrites at the surface of Li-metal electrodes can lead to short-circuits and thermal runaways. Moreover, the limited electrochemical stability window (ESW) of organic liquid electrolytes (up to 4.2 V vs.
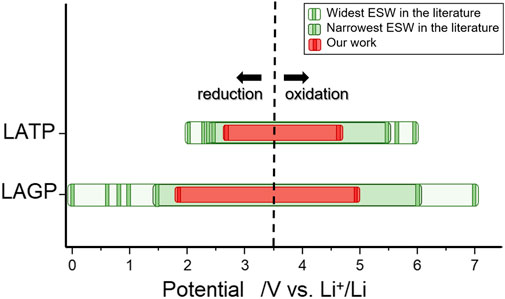
GRAPHICAL ABSTRACT. Electrochemical stability windows for Li1.3Al0.3Ti1.7(PO4)3 (LATP) and Li1.5Al0.5Ge1.5(PO4)3 (LAGP) reported in the literature.
ASSLBs are sparking rising interest thanks to their enhanced safety, achieved by replacing the flammable and reactive conventional liquid electrolyte with a safer and more thermally stable ceramic or polymer solid-state electrolyte (SE) (Gao et al., 2018; Zhang et al., 2018; Zheng et al., 2018; Bag and Thangadurai, 2020). Several families of ceramic SEs have been investigated based on their ionic conductivity as well as thermal and chemical stability. Sulfur-based SE such as Li10GeP2S12 from the thio-LiSICON family (derived from β-Li3PO4 crystal structure) and Argyrodites (products of the Li2S-P2S5-LiX phase diagram with X = Cl, Br, I), display very promising conductivities (up to 10−2 S.cm−1 at room temperature (Kamaya et al., 2011)) but suffer from severe chemical instability/sensitivity to air or moisture, causing the generation of toxic H2S gas (Muramatsu et al., 2011; Weber et al., 2016). Studies have also demonstrated the thermal and electrochemical instability of sulfide-based SE, leading to harmful battery degradation and safety issues (Sakuda et al., 2010; Muramatsu et al., 2011; Auvergniot et al., 2017; Chen et al., 2018; Zhang et al., 2018; Zhang et al., 2019). Oxide-based SE families, such as NASICONs (Na1+xZr2P3−xSixO12), garnets (A3B2X3O12) and perovskites (ABO3) display good conductivities (10−3/−4 S.cm−1 at room temperature) and have the advantage over sulfides to be less air and moisture-sensitive, leading to much easier handling despite requiring higher sintering temperatures (Meesala et al., 2017; Lau et al., 2018; Zheng et al., 2018). Therefore, oxide-based ceramics appear to be the most practical and reliable type of SE developed to date. NASICON-type SEs such as Li1.5Al0.5Ge1.5(PO4)3 (LAGP) and Li1.3Al0.3Ti1.7(PO4)3 (LATP) were particularly investigated for their ionic conductivities up to 1.09 × 10–3 S.cm–1 and 5.08 × 10−3 S.cm–1 at room temperature respectively (Thokchom and Kumar, 2008; Hupfer et al., 2016) and their stability against water and air (Imanishi et al., 2008; Hasegawa et al., 2009). ASSLBs are developed with the prospect of using lithium metal as negative electrode and high potential materials as positive electrode to significantly increase LIBs power and energy densities. ASSLBs can only meet these expectations because of the SEs presumed large electrochemical stability windows. However, assessing ESWs relies strongly on the type of characterization technique and experimental settings. Therefore, values for these electrochemical windows are very divergent in the literature published through the last decade. For example, as shown in Table 1, reported values for the ESWs of LAGP and LATP depend on the electrochemical technique used. Recently, several studies have come to specifically decry the frequent overestimation of solid electrolytes ESWs (Han et al., 2016; Tian et al., 2017; Zhang et al., 2018; Schwietert et al., 2020), which are proven to be much more limited in practice (Cui et al., 2016; Han et al., 2016; Wenzel et al., 2016; Chung and Kang, 2017). To characterize the ESW using cyclic voltammetry (CV), ceramic solid electrolytes are pressed into pellets and coated with a conductive metal (Au) before placing them between an inert blocking electrode (stainless steel, Pt or Au) and a polymer protected lithium metal electrode for cycling. In this configuration, a very limited contact area is available between the SE and the inert blocking electrode. Being in a solid-state medium, only the portion of SE in contact with the coated metal is expected to react. If the reaction forms a solid insulating phase, only the first few nanometers of the SE will react at the SE-Au coating interface as electrons cannot reach the reaction front. Resulting redox currents are therefore relatively small and hardly noticeable on CV curves. Moreover, using high sweeping speeds and omitting the ohmic drop correction can also alter the CV curve (Bard and Faulkner, 2000). Tested through this method, very wide ESWs can be reported. However, solid electrolytes including LAGP, LATP, Li0.35La0.55TiO3 (LLTO), and Li3.5Zn0.25GeO4 (LiSICON) were investigated through alternative techniques, such as in situ X-ray Photoelectron Spectroscopy (XPS) and Energy-Dispersive X-ray Spectroscopy (EDS), the results revealed much higher reduction potentials than previously established by CV (Alpen et al., 1978; Knauth, 2009; Hartmann et al., 2013; Wenzel et al., 2015). Based on first-principles studies, most ESWs appear much narrower than expected experimentally (Ong et al., 2013; Zhu et al., 2015; Richards et al., 2016; Zhu et al., 2016). Zhu et al. (2015) suggest that the practical stability of SE materials is not thermodynamically intrinsic but is rather due to kinetic phase stabilizations. The sluggish kinetics of the decomposition reactions happening at the extremes of the ESWs cause a high overpotential, leading to wider experimental electrochemical windows. To justify this difference, Schwietert et al. (2020) suggested that the favourable decomposition pathway for some SE was indirect rather than direct, via (de)lithiated states of the solid electrolyte, into the thermodynamically stable decomposition products.
In this study, we intend to establish a robust way to efficiently assess ESWs for the two NASICON-type materials Li1+xAlxM2-x (PO4)3 (M = Ge, Ti). Using first principles, we computed thermodynamic ESWs for LATP and LAGP and predicted their decomposition products upon redox potentials to gain more insight on the decomposition mechanisms. Experimentally, spark plasma sintering (SPS) technology was used to sinter together active material (SE) and conductive metal (Au) into a composite electrode to maximize the contact area between them and therefore increase the decomposition currents. Using Au as the conductive metal instead of commonly used carbon black prevent any side/decomposition reaction during sintering. Additionally, carbon black has the disadvantage to absorb a significant amount of moisture despite extensive drying (Plummer, 1930; Dewey et al., 1932; Liu et al., 2017), resulting in electrochemical artifacts above 4 V vs.
Materials and Methods
Grand Potential Phase Diagram (GPPD)
The GPPD is used to compute the ESWs of LAGP and LATP. The GPPD is constructed from a phase diagram by keeping the system open to Li. To construct such phase diagrams, the relevant free energy is the grand potential Φ, it is determined for each composition c at a given chemical potential μLi. The grand potential is derived from the Legendre transform of the energy (Ong et al., 2008), following the equation:
Where E(c) is the energy of composition c, nLi(c) is the number of lithium atoms found in the composition c and μLi is the chemical potential of lithium. The GPPD is constructed from the grand potentials of all relevant compositions at 0 K and incorporates all the stable phases of the initial phase diagram. The electrochemical stability window of a SE is the range of potentials over which the SE phase is considered stable. When using the GPPD, the ESW is the range of chemical potentials μLi over which the SE composition is considered stable (energy above Hull = 0) relatively to competing compositions of the phase diagram. The conversion from chemical potential to potential vs.
Where μ Li is the standard computed energy for lithium metal, e is the elementary electron charge. Decomposition products for the SEs are identified to be the most stable compositions (energy above Hull = 0) in which the SE decomposes, at the ESW limits.
All relevant compositions and their computed enthalpies are collected from The Materials Project database (Jain et al., 2013). The GPPD is generated for LAGP and LATP phase diagrams over a [0–8.0] V vs.
Reactants Syntheses
LAGP ceramic was synthesized using LiOH·H2O (Nemaska Lithium), Al(OH)3 (Alfa Aesar), GeO2 (Strem) and (NH4)2HPO4 (Sigma Aldrich) in stoichiometric ratios. The starting materials were ground manually for 10 min in a porcelain mortar and heated in a Pyrex beaker at 400°C (2°C/min heating rate) for 18 h to allow complete phosphating and gradually remove all decomposition gases (i.e. NH3, H2O). The powder was melted at 1,200°C inside a fused silica crucible for 1 h and the resulting liquid was casted into a stainless-steel mold at room temperature. The resulting colorless glass was ground into a fine powder during 20 min in a zirconia high energy ball mill (SPEX 8,000M Mixer Mill). To obtain the LAGP ceramic, the glass powder was annealed at 580°C in a fused silica crucible for 48 h, between the glass transition (Tg = 520°C) and crystallization (Tc = 610°C) temperatures (Fu, 1997), allowing complete crystallization of the glass. All the heating steps were performed in an electric furnace. LATP was synthesized by dissolving stoichiometric amounts of NH4H2PO4 and TiO2 (Sigma Aldrich) in deionized water (Holzapfel et al., 2012). The resulting solution was stirred continuously and heated under reflux at 160°C for 16 h. After cooling down to 80°C, Al(OH)3 was added to the solution and stirred for 30 min. Dissolved LiOH·H2O in deionized water was added at last before drying the final mixture at 300°C overnight. The resulting powder was milled using a zirconia high energy ball mill (SPEX 8,000M Mixer Mill) for 30 min. Finally, the powder was loaded in a crucible and annealed at 900°C for 6 h (2°C/min heating rate).
Spark Plasma Sintering
SPS experiments were carried out to sinter SE-Au/SE stacks using a Dr Sinter Lab Jr series 632Lx SPS. Au powder (Sigma Aldrich, CAS number 265772, <45 μm, 99.99% trace metals basis) and SE ceramic powder (LAGP or LATP) were mixed in an agate mortar following a 25:75 wt% ratio of SE:Au (2:1 v%). Au is introduced in large quantities to make up for its high density and allow a large surface area of contact with LAGP or LATP. 150 mg of the resulting powder were loaded into a Grafoil® coated graphite die (Ø = 8 mm) and pressed between two graphite punches. The Grafoil® is used to favor the demolding. The graphite die was placed inside the SPS chamber between two graphite spacers and uniaxially pressed at 100 MPa for 15 min to maximize particle cohesion. 75 mg of the SE powder were homogenously spread on top of the pre-compressed SE-Au pellet. The graphite die was placed inside the SPS chamber a second time and pressed at 100 MPa under vacuum. The sintering was performed up to 650°C for LAGP-Au/LAGP and 850°C for LATP-Au/LATP at a 50°C/min heating rate, where it was kept for 3–5 min before cooling down to room temperature at a 100°C/min rate. Voltage and current used in the process were applied under automatic operation mode. The resulting SE-Au/SE stacks were then placed in an electric furnace and heated to 750°C at a 5°C/min rate, under air for 18 h, to gradually calcinate and remove the remaining Grafoil® off the pellets. The pellets were then manually polished using Buehler® silicon carbide papers of different grain sizes (280, 400, and 1,000 grits) followed by diamond polishing paper to reach a final surface finish of 1 μm.
Specific Surface Area
The specific surface area of the gold particles was determined by the Brunauer-Emmett-Teller (BET) theory using a Micromeritics Gemini V. Prior to measurements, 500 mg of gold were degassed for 1 h at 200°C under N2 gas to remove water.
Powder X-Ray Diffraction (PXRD)
PXRD measurements were carried out on pellets using a Panalytical Empyrean diffractometer with copper X-ray sealed tube (CuKα, operated at 45 kV/40 mA, automatic optics iCore and dCore, reflection transmission spinner and a hybrid pixel detector PIXcel 3D). PXRD measurements were collected at a 0.4°/min rate from 10 to 80° in 2θ.
Scanning Electron Microscopy (SEM)
Analyses of the stacks were carried out on an Oxford Instrument JEOL JSM-7600TFE microscope equipped with a field emission gun (FEG). Samples were mounted in epoxy resin, polished with Buehler® silicon carbide papers of different grain sizes (280, 400, and 1,000 grits) followed by diamond polishing paper to reach a final surface finish of 1 μm. The samples were then sputtered with chromium (10 nm). Views were taken with zooms ranging from ×30 to ×10k. SEM analyses were coupled to energy-dispersive X-ray spectroscopy (EDS) measurements using an Oxford Instruments X-Max N 80 with an 80 mm2 silicon drift detector (SDD).
Electrochemical Measurements
The SE-Au/SE stacks were tested in electrochemistry using two-electrode stainless steel Swagelok cells. All the stacks and Swagelok parts were thoroughly dried at 140°C before mounting them inside an argon-filled glovebox (4 ppm O2 and 0.1 ppm H2O). SE-Au/SE stacks acted as working electrode and electrolyte. A lithium metal electrode protected with a thin layer of polyethylene oxide (PEO) polymer-based electrolyte was placed against the SE part of the stack. A protective teflon ring was introduced to impede the PEO or Li metal from coming in contact with the positive electrode in case of POE creeping. PEO electrolyte was prepared by mixing PEO (5M, Sigma Aldrich) with Lithium Bis(trifluoromethylsulfonyl)amine (Solvionics) using a [EO]:[Li] ratio of 20:1 in a closed internal mixer Brabender® (30 ml volume of the working chamber) at 170 ± 5°C. The blend is then laminated between steel plates. The polymer synthesis was carried out in an argon-filled glovebox to minimize exposure to air and water (Foran et al., 2020; Mankovsky et al., 2020). The electrochemical tests were performed at 60°C on a VMP2 series multichannel potentiostat (Bio-Logic Science Instruments). The ESWs were measured using PITT, small voltage increments of 50 mV were applied to cycle the stacks from Eoc down to 0 V vs.
O2 Sensing
Oxygen sensing measurements were performed using a southland sensing OMD-501D oxygen sensor. The setup combines O2 sensing and accelerated PITT cycling on SE-Au/SE stacks for an operando measure of O2. The O2 sensor was assembled using a T-Swagelok cell under argon. The electrochemical cells were left at open-circuit potential for at least 6 h prior to cycling in order to establish an O2 baseline.
Transmission Electron Microscopy
Milling of samples by FIB was performed using an Hitachi FB 2000A focused ion beam (FIB) system operating at 30 keV with a 50–100 nm resolution. An intense beam of Ga ions is produced using a high-brightness liquid–metal ion source and a double lens focusing system. A gun to deposit tungsten was used to cover and protect areas selected for characterization. An in situ lift-out FIB technique was used to prepare TEM samples, it was used to extract a thin lamella, which was then fixed to a Cu half-disk TEM support using tungsten deposition and welding. TEM observations were carried out using an Oxford Instruments JEOL JEM 2100F FEG-TEM microscope, operated at 200 kV. Image acquisition was done on bright field mode.
Results and Discussion
Grand Potential Phase Diagram
Table 2 shows the ESWs for LAGP and LATP computed in this study and compare them to other values found in the literature. LAGP was found stable between 2.72 and 4.29 V vs.
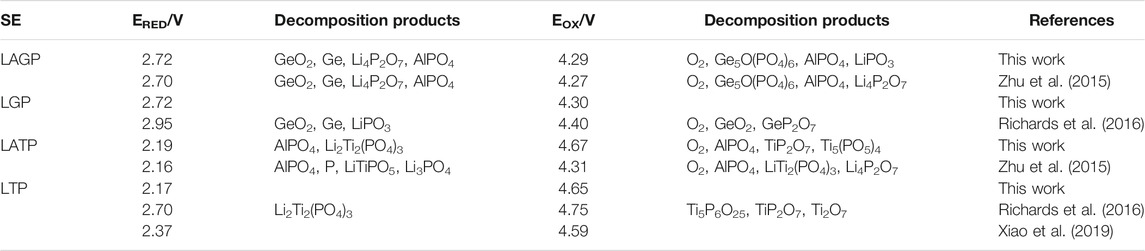
TABLE 2. Computed electrochemical stability windows for LAGP and LATP using the GPPD and their computed decomposition products.
Synthesis and Sintering of SE-Au/SE Stacks
Prior to any electrochemical measurement, it is essential to validate the quality of the stack preparation both from a chemical and a mechanical point of view. Unexpected reactions between SE and gold particles are likely to occur during the sintering at high temperature (Delaizir et al., 2012). Figure 1 displays the PXRD patterns of the pristine materials used in the present study, after sintering the solid electrolyte pellets and after sintering the composite electrodes. In Figure 1A, the PXRD pattern of the sintered LAGP pellet overlaps perfectly with the pattern of the pristine material (non-sintered) and corresponds to the NASICON-type structure crystallizing in the R-3c space group. No other peak is visible on the pattern confirming that, based on PXRD analyses, no impurity is present, and no side reaction occurred after the sintering of LAGP. The sintered stack was also analyzed, the pattern of the SE-Au side is presented in the figure. It matches exactly that of the sintered LAGP except for four peaks ascribed to Au (at 38.26°, 44.46°, 64.64°, and 77.62° for Au crystallized in a cubic Fm-3m space group). This indicates that no reaction between LAGP and gold particles has occurred during sintering. No peak shift is neither detected, meaning that no elemental interdiffusion occurred at the interface between LAGP and Au. In Figure 1B, the patterns of pristine LATP powder, sintered LATP pellet and sintered LATP-Au composite are presented. As observed for LAGP, the PXRD pattern of the sintered LATP pellet coincides perfectly with the pattern of the pristine LATP powder. It presents only peaks expected from the R-3c space group. The peaks observed on the pattern of the LATP-Au composite electrode fit with that of the sintered LATP and Au structures. The presence of a smooth baseline and sharp peaks on the sintered LATP and LAGP pellets discards the possibility of forming an amorphous phase during sintering. Based on these PXRD analyses, we confirm that SPS does not affect the structural integrity of LATP nor LAGP and that gold particles do not interact with the SEs at any point of the sintering process.
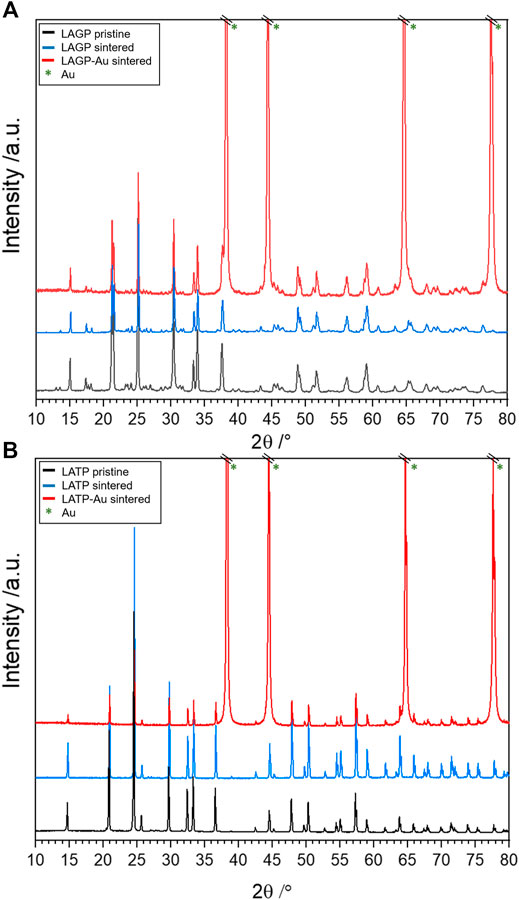
FIGURE 1. PXRD pattern of (A) LAGP and (B) LATP pristine powders compared to the sintered pellets, with and without gold particles, using SPS.
The SPS technique allows the densification of ceramic pellets from 80% up to 95% compacity (measured density/theoretical density × 100). Such high densities are required to ensure grain cohesion and minimize the cell polarization during electrochemical cycling (Aboulaich et al., 2011). Grain cohesion is important to provide proper electronic conductivity in the composite and proper ionic conductivity throughout the whole system. In this regard, the electronic conductivity of our system was measured using a Keithley 2401 multimeter in “in plane 4-points” measuring mode. It was found to be around 5 S/cm for the SE-Au composite, proving that the sintering of gold particles successfully formed an electronic network in the composite. The complete SE-Au/SE stack was insulating (infinite resistance), proving that the sintering did not affect the insulating properties of the solid electrolyte. After sintering, the resulting pellet is shown in Figure 2A. It consists of a bilayered pellet with a clear separation line, visible all around the pellet’s circumference, between the electrolyte (white) and the composite (golden) parts. To observe the SE-Au/SE interface in more details, the stack was polished in its cross-section and ran through SEM. A large scale view of the cross section is displayed in Figure 2B where both layers of the stacks are still visible and appear clean and homogeneous across several millimeters. The electrolyte side appears less bright due to its electronic insulating character. In contrast, the composite is bright all over the area owing to the electronic conduction of the gold particles. In Figure 2C, higher magnifications are used and allow us to determine the thickness of each part of the bilayer. Thicknesses of 200 and 400 μm were measured for SE-Au and SE sections respectively for this sample. All the sintered pellets displayed roughly the same dimensions, although the SE layer of some samples was further polished to a thickness of 150 μm before undergoing electrochemical tests. Moreover, very low internal porosity is observed on the micrographs indicating that the stack is sufficiently dense. EDS analyses were run with a highlight on Ge and Au elemental distributions over the surface of the SE-Au/SE interface. It shows a homogeneous distribution of Au in the SE-Au composite. For the sake of conciseness, only micrographs of LAGP-Au/LAGP stacks were shown, but the exact same construction is observed for LATP-Au/LATP stacks.
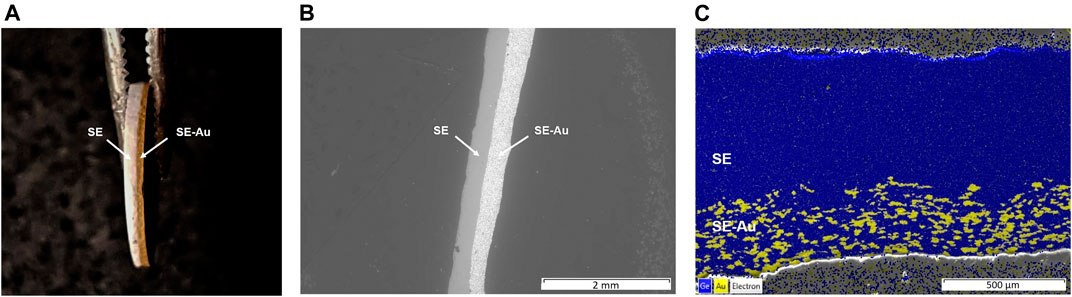
FIGURE 2. (A) Picture of a SE-Au/SE stack after completing sintering, calcination and polishing processes, (B) SEM micrograph of an LAGP-Au/LAGP stack cross section (×30), gold particles appear in light gray, (C) SEM micrograph and corresponding EDS analysis map of an LAGP-Au/LAGP stack cross section (×120), Au is represented in yellow, Ge in blue.
SPS allows the sintering of SE-Au and SE pellets into one compact stack while avoiding any side reaction between the SE and Au components. This confirms the suitability of SPS for producing optimal ceramic stacks for the determination of solid electrolytes ESWs. The specific surface area of the gold powder was measured using BET, it was estimated to be 0.4165 m2/g. The sintered SE-Au pellets contain 100 mg of gold resulting in a contact area between SE and Au largely superior to 0.5 cm2, which is the contact surface calculated for an 8 mm diameter SE pellet covered by an Au layer. Sintered SE and Au composite electrodes offer more electrochemical surface than conventional Au-coating on SE pellets, ensuring greater decomposition currents.
Electrochemical Measurements
To determine the electrochemical stability windows, LAGP-Au/LAGP and LATP-Au/LATP Swagelok cells were assembled. Two cells of each were used for the study of the oxidation and reduction separately. The cells were cycled using PITT, a widely used method in electrochemistry that allows the system to remain close to the thermodynamic equilibrium and to neglect interfacial resistances. This method is also used, along with galvanostatic intermittent titration technique (GITT), for determining the diffusion coefficient in electrochemical materials, such as lithium diffusion in lithium-ion battery electrodes (Wen et al., 1979). In the context of this study, PITT is used to observe the current response at each potential step after the system returns to equilibrium. Using PITT allows the observation of the first redox currents generated by LAGP and LATP, which provides us with a precise ESW. The same approach was recently used by Zhang et al. (2021) to determine the electrochemical stability of single-ion conducting polymer electrolytes. The experiment starts by recording the Eoc of the battery. A potential increment of 50 mV is applied for oxidation and reduction respectively. During oxidation and reduction, the SE-Au layer acts as a positive electrode and POE protected lithium metal as negative electrode. PITT curves for LAGP are given in Figure 3. The graph is presented as a current response vs. potential for the ease of reading, however the typical PITT response i.e. I vs. t, is displayed in Supplementary Figure S1. Observing the reduction curve, each potential step from the Eoc at ca. 3.0 to 1.85 V vs.
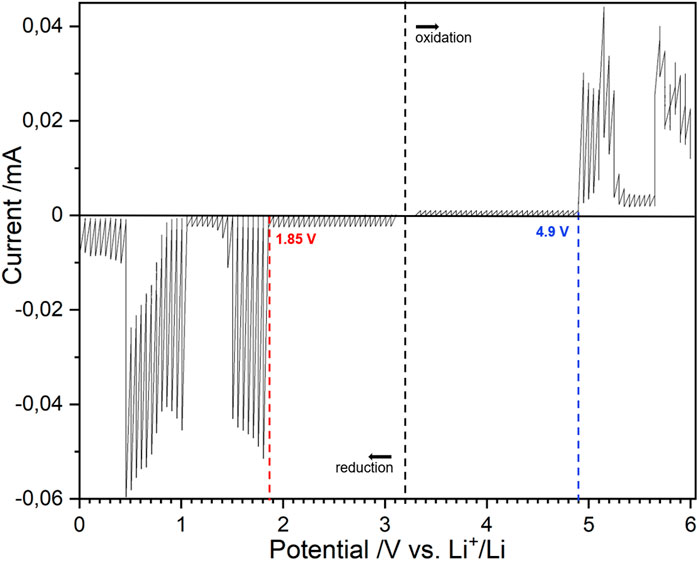
FIGURE 3. PITT measurements on a stack LAGP-Au/LAGP represented using the current response as a function of potential. The two measures were taken on two distinct stacks at 60°C, from Eoc to 6 V for oxidation and from Eoc to 0 V vs. Li+/Li for reduction. A potential step of 50 mV, a current limit of 10 nA and a time limit of 3 h were used.
Similarly, the electrochemical stability window for LATP is presented in Figure 4 (i.e. I vs. t, is displayed in Supplementary Figure S2). A noticeable reduction current is observed as early as 2.65 V and becomes more significant at 2.5 V vs.
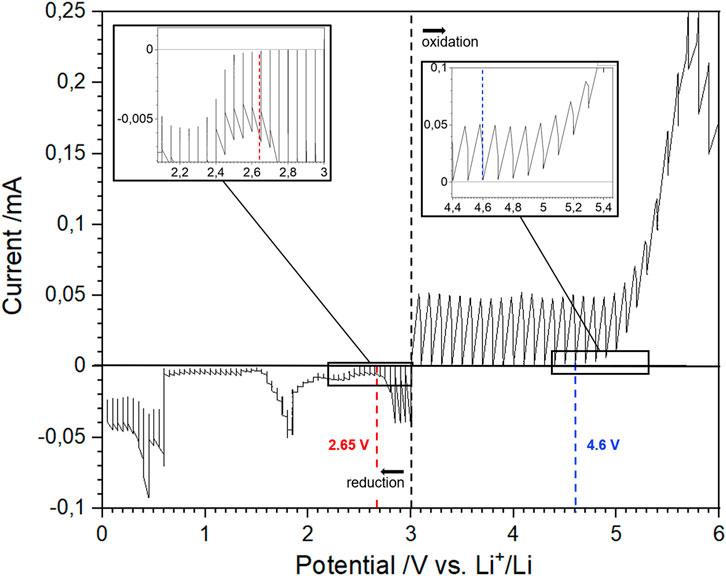
FIGURE 4. PITT measurements on a stack LATP-Au/LATP. The two measures were taken on two distinct stacks at 60°C, from Eoc to 6 V for oxidation and from Eoc to 0 V vs. Li+/Li for reduction. A potential step of 50 mV, a current limit of 10 nA and a time limit of 3 h were used.
O2 Sensing Measurements
Decomposition products upon reduction, especially against lithium metal, were widely investigated for LAGP (Paolella et al., 2020; Sun et al., 2020) and LATP (Hartmann et al., 2013; Chung and Kang, 2017; Inozemtseva et al., 2020; Zhu et al., 2020; Hou et al., 2020). However, experimental studies on their decomposition upon oxidation are nonexistent. First-principles calculations have been used to foresee these products but to the best of our knowledge, no experimental study has been successful yet in the determination of such decomposition products at high potential. O2 being among the decomposition products predicted by first-principles calculations (Table 2), we used an oxygen sensing probe to investigate the possibility of O2 production at LATP and LAGP oxidation potentials. The setup is presented in Figure 5, the electrochemical cell is coupled to the sensing probe so that the amount of O2 produced is detected operando. Results of this experiment are shared in Figure 6. The objective of this experiment was to correlate the amount of charge going through the system with the amount of O2 released, as expected from our calculations. Accelerated PITT was used to avoid diluting the O2 signal in extended relaxation times. Done this way, it is possible to observe a sharper signal for O2 generated as a function of time. The ESW might be slightly altered by this change of parameters but the experiment is focused primarily on detecting a clear evolution of O2 upon oxidation. For both LAGP and LATP, the amount of O2 detected follows a smooth baseline during the relaxation period (left at Eoc for 6 h) and during the beginning of the accelerated PITT, confirming that no O2-producing reaction is occurring in this potential range, When the oxidation potentials are reached at 4.9 and 4.6 V vs.
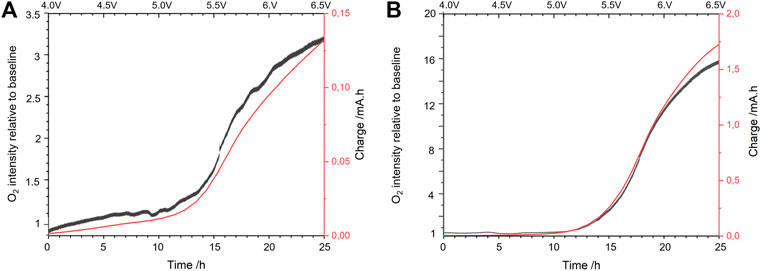
FIGURE 6. Operando O2 sensing measurements for (A) LAGP and (B) LATP coupled to accelerated PITT in oxidation. SE-Au/SE stacks of LAGP and LATP were used within three-outlet Swagelok cells. The accelerated PITT is presented as the charge Q as a function of time. A potential step of 100 mV, a current limit of 10 nA and a time limit of 1 h were used.
Using PXRD, a first attempt to characterize the decomposition products predicted by our computations failed to show the presence of crystalline phases such as AlPO4, Ge5O(PO4)6, TiP2O7, and LiPO3 after LAGP or LATP oxidations. Our hypotheses are that the decomposition products are 1) amorphous and/or 2) present in very small amount due the solid character of our compounds. Indeed, only the grains of SE that are directly in contact with gold particles get to react. The effective potential vehicled by the gold particles reaches this first layer of SE. However, assuming that the SE particles and their decomposition products are electronic insulators, the second layer of SE grains will not be affected by the potential applied on the gold particles. This system impedes the effective potential to reach the rest of the SE layers which are, therefore, not oxidized.
Transmission Electron Microscopy
TEM observations were carried out to investigate the oxidation reaction of LAGP within the LAGP-Au composite before and after cycling. The micrograph presented in Figure 7A displays a sintered LAGP-Au thin lamella. The conductive gold particles (in black) are surrounded by LAGP ceramic grains (in gray). It was possible to measure the size of LAGP particles, they lie between 500 and 800 nm (Supplementary Figure S3A). Selected area electron diffraction (SAED) measurements confirmed the crystalline nature of Au and LAGP particles. The selected patterns show interplanar spacings of 2.36 Å for Au-(111) (Dilshad et al., 2012) and 3.66 Å for LAGP-(113) (Wang et al., 2020) (Supplementary Figures S4A,B). Very few cavities are observed between the grains (except the ones created by the ion beam), confirming the good sintering of the particles. Micrographs collected for the LAGP-Au composite after oxidation are displayed in Figure 7B. The same distribution is observed for LAGP grains with sizes ranging from 500 to 800 nm. A layer is observed at the LAGP-Au interface with a thickness ranging from 10 to 30 nm (Supplementary Figures S3B–D), the bright white color seen on the TEM micrograph and the continuous circle observed from SAED measurements point out to an amorphous phase (Supplementary Figure S4C). Information collected through TEM and SAED measurements indicate that the LAGP layer in contact with Au underwent a phase transformation leading to the formation of amorphous decomposition products, possibly Ge5O(PO4)6, AlPO4, and LiPO3 predicted by first-principles calculations, but which is difficult to confirm experimentally at this stage.
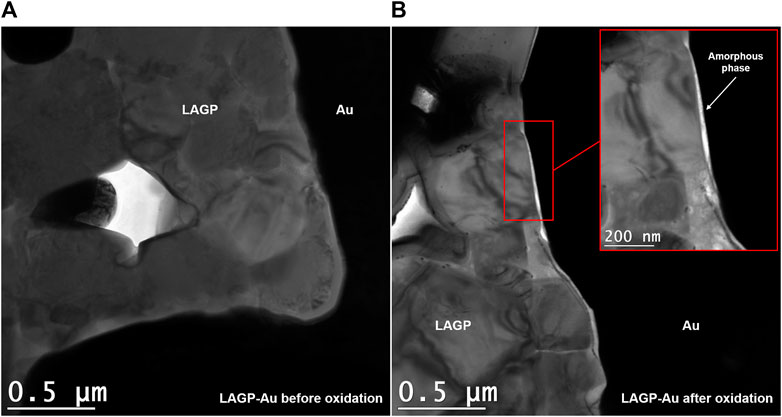
FIGURE 7. Bright-field TEM micrographs (×10k) of two LAGP-Au composite samples (A) before and (B) after oxidation. The inset was observed at ×25k.
EIS measurements were carried out from Eoc to 5.6 V and are presented in Supplementary Figure S5. The first semi-circle observed at high frequencies (7 kHz) is attributed to the bulk SE and remains constant, independently of the applied potential. At lower frequencies, we observe a tail, which is attributed to the charge accumulation at the Au blocking electrode from Eoc to 4.9 V. Passed this potential, another large semi-circle (i.e. associated resistance above 4 MΩ) appears, which confirms that a sluggish charge transfer process now occurs at the SE-Au interface above 4.9 V vs.
Conclusion
The experimental setup used in this study enabled the determination of accurate ESWs for LAGP and LATP solid electrolytes with carefully selected parameters: using inert gold particles instead of carbon black singled out the redox mechanisms of the solid electrolytes, sintering SEs with gold particles into composites enhanced the redox current responses and using PITT allowed to work close to the thermodynamic equilibrium of the system. The electrochemical stability windows were found to be [1.85–4.9 V] vs.
Data Availability Statement
The original contributions presented in the study are included in the article/Supplementary Material, further inquiries can be directed to the corresponding author.
Author Contributions
YB, SR, and MD conceived the project. YB carried out the experiments, performed the computations and wrote the manuscript with support from SR. MR carried out the syntheses. GH supervised the computational part. MD supervised the experimental part. GH and MD reviewed the article and provided funding for the present study.
Funding
YB is grateful for the financial support of the Fonds de la Recherche Scientifique – FNRS of Belgium through a FRIA grant (FC31481) and the Natural Sciences and Engineering Research Council of Canada - NSERC through a BESC D grant (BESCD3-534841-2019). MR, SR, and MD acknowledge the financial support received from the Natural Sciences and Engineering Research Council of Canada (NSERC RDCPJ 528052-18).
Conflict of Interest
The authors declare that the research was conducted in the absence of any commercial or financial relationships that could be construed as a potential conflict of interest.
Acknowledgments
We thank D. Lepage for his valuable insight into the experimental part, G. Bokas and S. Posada-Pérez for their help on the computational section. We acknowledge the contribution of J.-P. Masse to the analysis and interpretation of the TEM results. The present research benefited from computational resources provided by the Université catholique de Louvain (CISM/UCLouvain) and by the Consortium des Équipements de Calcul Intensif en Fédération Wallonie-Bruxelles (CÉCI).
Supplementary Material
The Supplementary Material for this article can be found online at: https://www.frontiersin.org/articles/10.3389/fenrg.2021.682008/full#supplementary-material
References
Aboulaich, A., Bouchet, R., Delaizir, G., Seznec, V., Tortet, L., Morcrette, M., et al. (2011). A New Approach to Develop Safe All-Inorganic Monolithic Li-Ion Batteries. Adv. Energ. Mater. 1 (2), 179–183. doi:10.1002/aenm.201000050
Alpen, U. v., Bell, M. F., Wichelhaus, W., Cheung, K. Y., and Dudley, G. J. (1978). Ionic Conductivity of Li14Zn(GeO44 (Lisicon). Electrochimica Acta 23 (12), 1395–1397. doi:10.1016/0013-4686(78)80023-1
Aurbach, D., Zinigrad, E., Cohen, Y., and Teller, H. (2002). A Short Review of Failure Mechanisms of Lithium Metal and Lithiated Graphite Anodes in Liquid Electrolyte Solutions. Solid State Ionics 148 (3), 405–416. doi:10.1016/S0167-2738(02)00080-2
Auvergniot, J., Cassel, A., Ledeuil, J.-B., Viallet, V., Seznec, V., and Dedryvère, R. (2017). Interface Stability of Argyrodite Li6PS5Cl toward LiCoO2, LiNi1/3Co1/3Mn1/3O2, and LiMn2O4 in Bulk All-Solid-State Batteries. Chem. Mater. 29 (9), 3883–3890. doi:10.1021/acs.chemmater.6b04990
Bag, S., and Thangadurai, V. (2020). CHAPTER 3 Electrolyte Development for Solid-State Lithium Batteries. In Energy Storage and Conversion Materials. London, UK: The Royal Society of Chemistry, 100–135.
Bard, A. J., and Faulkner, L. R. (2000). Electrochemical Methods and Applications. New York; London: Wiley-Interscience.
Birke, P., Salam, F., Döring, S., and Weppner, W. (1999). A First Approach to a Monolithic All Solid State Inorganic Lithium Battery. Solid State Ionics 118 (1), 149–157. doi:10.1016/S0167-2738(98)00462-7
Blöchl, P. E. (1994). Projector Augmented-Wave Method. Phys. Rev. B 50 (24), 17953–17979. doi:10.1103/PhysRevB.50.17953
Chen, S., Xie, D., Liu, G., Mwizerwa, J. P., Zhang, Q., Zhao, Y., et al. (2018). Sulfide Solid Electrolytes for All-Solid-State Lithium Batteries: Structure, Conductivity, Stability and Application. Energ. Storage Mater. 14, 58–74. doi:10.1016/j.ensm.2018.02.020
Chung, H., and Kang, B. (2017). Mechanical and Thermal Failure Induced by Contact between a Li1.5Al0.5Ge1.5(PO4)3 Solid Electrolyte and Li Metal in an All Solid-State Li Cell. Chem. Mater. 29 (20), 8611–8619. doi:10.1021/acs.chemmater.7b02301
Cui, Y., Rohde, M., Reichmann, T. L., Mahmoud, M. M., Ziebert, C., and Seifert, H. J. (2016). Ionic Conductivity and Stability of the Lithium Aluminum Germanium Phosphate. ECS Trans. 72 (8), 139–146. doi:10.1149/07208.0139ecst
Delaizir, G., Viallet, V., Aboulaich, A., Bouchet, R., Tortet, L., Seznec, V., et al. (2012). The Stone Age Revisited: Building a Monolithic Inorganic Lithium-Ion Battery. Adv. Funct. Mater. 22 (10), 2140–2147. doi:10.1002/adfm.201102479
Delmas, C., Nadiri, A., and Soubeyroux, J. L. (1988). The Nasicon-type Titanium Phosphates Ati2(PO4)3 (A=Li, Na) as Electrode Materials. Solid State Ionics 28-30, 419–423. doi:10.1016/S0167-2738(88)80075-4
Dewey, C. S., Lefforge, P. K., and Cabot, G. L. (1932). Moisture Sorption by Carbon Black. Ind. Eng. Chem. 24 (9), 1045–1050. doi:10.1021/ie50273a019
Dilshad, N., Ansari, M. S., Beamson, G., and Schiffrin, D. J. (2012). Amines as Dual Function Ligands in the Two-phase Synthesis of Stable AuxCu(1−x) Binary Nanoalloys. J. Mater. Chem. 22 (21), 10514–10524. doi:10.1039/C2JM31709E
Feng, J. K., Lu, L., and Lai, M. O. (2010). Lithium Storage Capability of Lithium Ion Conductor Li1.5Al 0.5Ge1.5(PO4)3.
Feng, J. K., Yan, B. G., Liu, J. C., Lai, M. O., and Li, L. (2013). All Solid State Lithium Ion Rechargeable Batteries Using NASICON Structured Electrolyte. Mater. Technol. 28 (5), 276–279. doi:10.1179/1753555713Y.0000000085
Foran, G., Mankovsky, D., Verdier, N., Lepage, D., Prébé, A., Aymé-Perrot, D., et al. (2020). The Impact of Absorbed Solvent on the Performance of Solid Polymer Electrolytes for Use in Solid-State Lithium Batteries. iScience 23 (10), 101597. doi:10.1016/j.isci.2020.101597
Fu, J. (1997). Fast Li+ Ion Conduction in Li2O-Al2O3-TiO2-SiO2-P2o2 Glass-Ceramics. J. Am. Ceram. Soc. 80 (7), 1901–1903. doi:10.1111/j.1151-2916.1997.tb03070.x
Gao, Z., Sun, H., Fu, L., Ye, F., Zhang, Y., Luo, W., et al. (2018). Promises, Challenges, and Recent Progress of Inorganic Solid-State Electrolytes for All-Solid-State Lithium Batteries. Adv. Mater. 30 (17), 1705702. doi:10.1002/adma.201705702
Han, F., Zhu, Y., He, X., Mo, Y., and Wang, C. (2016). Electrochemical Stability of Li10 GeP2 S12 and Li7 La3 Zr2 O12 Solid Electrolytes. Adv. Energ. Mater. 6 (8), 1501590. doi:10.1002/aenm.201501590
Hartmann, P., Leichtweiss, T., Busche, M. R., Schneider, M., Reich, M., Sann, J., et al. (2013). Degradation of NASICON-type Materials in Contact with Lithium Metal: Formation of Mixed Conducting Interphases (MCI) on Solid Electrolytes. J. Phys. Chem. C 117 (41), 21064–21074. doi:10.1021/jp4051275
Hasegawa, S., Imanishi, N., Zhang, T., Xie, J., Hirano, A., Takeda, Y., et al. (2009). Study on Lithium/air Secondary Batteries-Stability of NASICON-type Lithium Ion Conducting Glass-Ceramics with Water. J. Power Sourc. 189 (1), 371–377. doi:10.1016/j.jpowsour.2008.08.009
Hou, M., Liang, F., Chen, K., Dai, Y., and Xue, D. (2020). Challenges and Perspectives of NASICON-type Solid Electrolytes for All-Solid-State Lithium Batteries. Nanotechnology 31 (13), 132003. doi:10.1088/1361-6528/ab5be7
Hupfer, T., Bucharsky, E. C., Schell, K. G., Senyshyn, A., Monchak, M., Hoffmann, M. J., et al. (2016). Evolution of Microstructure and its Relation to Ionic Conductivity in Li1+xAlxTi2−x(PO4)3. Solid State Ionics 288, 235–239. doi:10.1016/j.ssi.2016.01.036
Imanishi, N., Hasegawa, S., Zhang, T., Hirano, A., Takeda, Y., and Yamamoto, O. (2008). Lithium Anode for Lithium-Air Secondary Batteries. J. Power Sourc. 185 (2), 1392–1397. doi:10.1016/j.jpowsour.2008.07.080
Inozemtseva, A. I., Vizgalov, V. A., Kapitanova, O. O., Panin, G., Velasco Vélez, J. J., Itkis, D. M., et al. (2020). In Situ XPS Studies of Solid Electrolyte Electroreduction through Graphene Electrode. J. Electrochem. Soc. 167 (11), 110533. doi:10.1149/1945-7111/aba370
Jain, A., Ong, S. P., Hautier, G., Chen, W., Richards, W. D., Dacek, S., et al. (2013). Commentary: The Materials Project: A Materials Genome Approach to Accelerating Materials Innovation. APL Mater. 1 (1), 011002. doi:10.1063/1.4812323
Jiang, P., Porsgaard, S., Borondics, F., Köber, M., Caballero, A., Bluhm, H., et al. (2010). Room-Temperature Reaction of Oxygen with Gold: An In situ Ambient-Pressure X-Ray Photoelectron Spectroscopy Investigation. J. Am. Chem. Soc. 132 (9), 2858–2859. doi:10.1021/ja909987j
Kamaya, N., Homma, K., Yamakawa, Y., Hirayama, M., Kanno, R., Yonemura, M., et al. (2011). A Lithium Superionic Conductor. Nat. Mater 10 (9), 682–686. doi:10.1038/nmat3066
Knauth, P. (2009). Inorganic Solid Li Ion Conductors: An Overview. Solid State Ionics 180 (14), 911–916. doi:10.1016/j.ssi.2009.03.022
Kotobuki, M., Hoshina, K., and Kanamura, K. (2011). Electrochemical Properties of Thin TiO2 Electrode on Li1+xAlxGe2−x(PO4)3 Solid Electrolyte. Solid State Ionics 198 (1), 22–25. doi:10.1016/j.ssi.2011.07.003
Kresse, G., and Furthmüller, J. (1996). Efficient Iterative Schemes Forab Initiototal-Energy Calculations Using a Plane-Wave Basis Set. Phys. Rev. B 54 (16), 11169–11186. doi:10.1103/PhysRevB.54.11169
Lau, J., DeBlock, R. H., Butts, D. M., Ashby, D. S., Choi, C. S., and Dunn, B. S. (2018). Sulfide Solid Electrolytes for Lithium Battery Applications. Adv. Energ. Mater. 8 (27), 1800933. doi:10.1002/aenm.201800933
Li, W. (1997). Morphology Effects on the Electrochemical Performance of LiNi[sub 1−x]Co[sub x]O[sub 2]. J. Electrochem. Soc. 144 (8), 2773. doi:10.1149/1.1837894
Liang, X., Han, D., Wang, Y., Lan, L., and Mao, J. (2018). Preparation and Performance Study of a PVDF-LATP Ceramic Composite Polymer Electrolyte Membrane for Solid-State Batteries. RSC Adv. 8 (71), 40498–40504. doi:10.1039/C8RA08436J
Lin, D., Liu, Y., and Cui, Y. (2017). Reviving the Lithium Metal Anode for High-Energy Batteries. Nat. Nanotech 12 (3), 194–206. doi:10.1038/nnano.2017.16
Liu, L., Tan, S., Horikawa, T., Do, D. D., Nicholson, D., and Liu, J. (2017). Water Adsorption on Carbon - A Review. Adv. Colloid Interf. Sci. 250, 64–78. doi:10.1016/j.cis.2017.10.002
Liu, Z., Yu, A., and Lee, J. Y. (1999). Synthesis and Characterization of LiNi1−x−yCoxMnyO2 as the Cathode Materials of Secondary Lithium Batteries. J. Power Sourc. 81-82, 416–419. doi:10.1016/S0378-7753(99)00221-9
Mankovsky, D., Lepage, D., Lachal, M., Caradant, L., Aymé-Perrot, D., and Dollé, M. (2020). Water Content in Solid Polymer Electrolytes: the Lost Knowledge. Chem. Commun. 56 (70), 10167–10170. doi:10.1039/D0CC03556D
Meesala, Y., Jena, A., Chang, H., and Liu, R.-S. (2017). Recent Advancements in Li-Ion Conductors for All-Solid-State Li-Ion Batteries. ACS Energ. Lett. 2 (12), 2734–2751. doi:10.1021/acsenergylett.7b00849
Holzapfel, G. W., Nuspl, G., and Busl, S. (2012). Phase-Pure Lithium Aluminium Titanium Phosphate And Method For Its Production And Use.
Muramatsu, H., Hayashi, A., Ohtomo, T., Hama, S., and Tatsumisago, M. (2011). Structural Change of Li2S-P2s5 Sulfide Solid Electrolytes in the Atmosphere. Solid State Ionics 182 (1), 116–119. doi:10.1016/j.ssi.2010.10.013
Ong, S. P., Mo, Y., Richards, W. D., Miara, L., Lee, H. S., and Ceder, G. (2013). Phase Stability, Electrochemical Stability and Ionic Conductivity of the Li10±1MP2X12(M = Ge, Si, Sn, Al or P, and X = O, S or Se) Family of Superionic Conductors. Energy Environ. Sci. 6 (1), 148–156. doi:10.1039/C2EE23355J
Ong, S. P., Richards, W. D., Jain, A., Hautier, G., Kocher, M., Cholia, S., et al. (2013). Python Materials Genomics (Pymatgen): A Robust, Open-Source python Library for Materials Analysis. Comput. Mater. Sci. 68, 314–319. doi:10.1016/j.commatsci.2012.10.028
Ong, S. P., Wang, L., Kang, B., and Ceder, G. (2008). Li−Fe−P−O2 Phase Diagram from First Principles Calculations. Chem. Mater. 20 (5), 1798–1807. doi:10.1021/cm702327g
Orsini, F., Du Pasquier, A., Beaudoin, B., Tarascon, J. M., Trentin, M., Langenhuizen, N., et al. (1998). In situ Scanning Electron Microscopy (SEM) Observation of Interfaces within Plastic Lithium Batteries. J. Power Sourc. 76 (1), 19–29. doi:10.1016/S0378-7753(98)00128-1
Paolella, A., Zhu, W., Xu, G. L., La Monaca, A., Savoie, S., Girard, G., et al. (2020). Understanding the Reactivity of a Thin Li 1.5 Al 0.5 Ge 1.5 (PO 4 ) 3 Solid-State Electrolyte toward Metallic Lithium Anode. Adv. Energ. Mater. 10 (32), 2001497. doi:10.1002/aenm.202001497
Perdew, J. P., Burke, K., and Ernzerhof, M. (1996). Generalized Gradient Approximation Made Simple. Phys. Rev. Lett. 77 (18), 3865–3868. doi:10.1103/PhysRevLett.77.3865
Plummer, W. B. (1930). Moisture Content of Carbon Blacks. Rubber Chem. Technol. 3 (2), 185–189. doi:10.5254/1.3535472
Richards, W. D., Miara, L. J., Wang, Y., Kim, J. C., and Ceder, G. (2016). Interface Stability in Solid-State Batteries. Chem. Mater. 28 (1), 266–273. doi:10.1021/acs.chemmater.5b04082
Sakuda, A., Hayashi, A., and Tatsumisago, M. (2010). Interfacial Observation between LiCoO2 Electrode and Li2S−P2S5 Solid Electrolytes of All-Solid-State Lithium Secondary Batteries Using Transmission Electron Microscopy. Chem. Mater. 22 (3), 949–956. doi:10.1021/cm901819c
Schwietert, T. K., Arszelewska, V. A., Wang, C., Yu, C., Vasileiadis, A., de Klerk, N. J. J., et al. (2020). Clarifying the Relationship between Redox Activity and Electrochemical Stability in Solid Electrolytes. Nat. Mater. 19, 428–435. doi:10.1038/s41563-019-0576-0
Shi, X., Ma, N., Wu, Y., Lu, Y., Xiao, Q., Li, Z., et al. (2018). Fabrication and Electrochemical Properties of LATP/PVDF Composite Electrolytes for Rechargeable Lithium-Ion Battery. Solid State Ionics 325, 112–119. doi:10.1016/j.ssi.2018.08.010
Sun, Q., He, L., Zheng, F., Wang, Z., An Oh, S. J., Sun, J., et al. (2020). Decomposition Failure of Li1.5Al0.5Ge1.5(PO4)3 Solid Electrolytes Induced by Electric Field: A Multi-Scenario Study Using Scanning Probe Microscopy-Based Techniques. J. Power Sourc. 471, 228468. doi:10.1016/j.jpowsour.2020.228468
Thackeray, M. M., David, W. I. F., Bruce, P. G., and Goodenough, J. B. (1983). Lithium Insertion into Manganese Spinels. Mater. Res. Bull. 18 (4), 461–472. doi:10.1016/0025-5408(83)90138-1
Thokchom, J. S., and Kumar, B. (2008). Composite Effect in Superionically Conducting Lithium Aluminium Germanium Phosphate Based Glass-Ceramic. J. Power Sourc. 185 (1), 480–485. doi:10.1016/j.jpowsour.2008.07.009
Tian, Y., Shi, T., Richards, W. D., Li, J., Kim, J. C., Bo, S.-H., et al. (2017). Compatibility Issues between Electrodes and Electrolytes in Solid-State Batteries. Energ. Environ. Sci. 10 (5), 1150–1166. doi:10.1039/C7EE00534B
Vetter, K. J., and Berndt, D. (1958). Stromdichte- und pH-Abhängigkeit des elektrochemischen Auf- und Abbaus von Oxydschichten auf Pt, Pd und Au. Zeitschrift für Elektrochemie. Berichte der Bunsengesellschaft für physikalische Chem. 62 (3), 378–386. doi:10.1002/bbpc.19580620329
Wang, J., Huang, G., Yan, J.-M., Ma, J.-L., Liu, T., Shi, M.-M., et al. (2020). Hybrid Solid Electrolyte Enabled Dendrite-free Li Anodes for High-Performance Quasi-Solid-State Lithium-Oxygen Batteries. Natl. Sci. Rev. 8 (2). doi:10.1093/nsr/nwaa150
Weber, D. A., Senyshyn, A., Weldert, K. S., Wenzel, S., Zhang, W., Kaiser, R., et al. (2016). Structural Insights and 3D Diffusion Pathways within the Lithium Superionic Conductor Li10GeP2S12. Chem. Mater. 28 (16), 5905–5915. doi:10.1021/acs.chemmater.6b02424
Wen, C. J., Boukamp, B. A., Huggins, R. A., and Weppner, W. (1979). Thermodynamic and Mass Transport Properties of “LiAl”. J. Electrochem. Soc. 126 (12), 2258–2266. doi:10.1149/1.2128939
Wenzel, S., Leichtweiss, T., Krüger, D., Sann, J., and Janek, J. (2015). Interphase Formation on Lithium Solid Electrolytes-An In Situ Approach to Study Interfacial Reactions by Photoelectron Spectroscopy. Solid State Ionics 278, 98–105. doi:10.1016/j.ssi.2015.06.001
Wenzel, S., Randau, S., Leichtweiß, T., Weber, D. A., Sann, J., Zeier, W. G., et al. (2016). Direct Observation of the Interfacial Instability of the Fast Ionic Conductor Li10GeP2S12 at the Lithium Metal Anode. Chem. Mater. 28 (7), 2400–2407. doi:10.1021/acs.chemmater.6b00610
Winter, M., and Besenhard, J. O. (1999). Wiederaufladbare Batterien. Chem. Unserer Zeit 33 (6), 320–332. doi:10.1002/ciuz.19990330603
Xiao, W., Wang, J., Fan, L., Zhang, J., and Li, X. (2019). Recent Advances in Li1+xAlxTi2−x(PO4)3 Solid-State Electrolyte for Safe Lithium Batteries. Energ. Storage Mater. 19, 379–400. doi:10.1016/j.ensm.2018.10.012
Xiao, Y., Miara, L. J., Wang, Y., and Ceder, G. (2019). Computational Screening of Cathode Coatings for Solid-State Batteries. Joule 3 (5), 1252–1275. doi:10.1016/j.joule.2019.02.006
Xu, X., Wen, Z., Wu, X., Yang, X., and Gu, Z. (2007). Lithium Ion-Conducting Glass? Ceramics of Li1.5Al0.5Ge1.5(PO4)3?xLi2O (x=0.0?0.20) with Good Electrical and Electrochemical Properties. J. Am. Ceram. Soc. 90 (9), 2802–2806. doi:10.1111/j.1551-2916.2007.01827.x
Xu, X., Wen, Z., Yang, X., Zhang, J., and Gu, Z. (2006). High Lithium Ion Conductivity Glass-Ceramics in Li2O-Al2O3-TiO2-P2o5 from Nanoscaled Glassy Powders by Mechanical Milling. Solid State Ionics 177 (26), 2611–2615. doi:10.1016/j.ssi.2006.04.010
Yoshio, M., Noguchi, H., Itoh, J.-i., Okada, M., and Mouri, T. (2000). Preparation and Properties of LiCoyMnxNi1−x−yO2 as a Cathode for Lithium Ion Batteries. J. Power Sourc. 90 (2), 176–181. doi:10.1016/S0378-7753(00)00407-9
Zhang, Q., Cao, D., Ma, Y., Natan, A., Aurora, P., and Zhu, H. (2019). Sulfide-Based Solid-State Electrolytes: Synthesis, Stability, and Potential for All-Solid-State Batteries. Adv. Mater. 31 (44), 1901131. doi:10.1002/adma.201901131
Zhang, W., Feng, S., Huang, M., Qiao, B., Shigenobu, K., Giordano, L., et al. (2021). Molecularly Tunable Polyanions for Single-Ion Conductors and Poly(solvate Ionic Liquids). Chem. Mater. 33 (2), 524–534. doi:10.1021/acs.chemmater.0c03258
Zhang, Z., Shao, Y., Lotsch, B., Hu, Y.-S., Li, H., Janek, J., et al. (2018). New Horizons for Inorganic Solid State Ion Conductors. Energ. Environ. Sci. 11 (8), 1945–1976. doi:10.1039/C8EE01053F
Zhang, Z., Zhang, L., Liu, Y., Yu, C., Yan, X., Xu, B., et al. (2018). Synthesis and Characterization of Argyrodite Solid Electrolytes for All-Solid-State Li-Ion Batteries. J. Alloys Compd. 747, 227–235. doi:10.1016/j.jallcom.2018.03.027
Zhang, Z., Zhao, Y., Chen, S., Xie, D., Yao, X., Cui, P., et al. (2017). An Advanced Construction Strategy of All-Solid-State Lithium Batteries with Excellent Interfacial Compatibility and Ultralong Cycle Life. J. Mater. Chem. A. 5 (32), 16984–16993. doi:10.1039/C7TA04320A
Zheng, F., Kotobuki, M., Song, S., Lai, M. O., and Lu, L. (2018). Review on Solid Electrolytes for All-Solid-State Lithium-Ion Batteries. J. Power Sourc. 389, 198–213. doi:10.1016/j.jpowsour.2018.04.022
Zhu, J., Zhao, J., Xiang, Y., Lin, M., Wang, H., Zheng, B., et al. (2020). Chemomechanical Failure Mechanism Study in NASICON-type Li1.3Al0.3Ti1.7(PO4)3 Solid-State Lithium Batteries. Chem. Mater. 32 (12), 4998–5008. doi:10.1021/acs.chemmater.9b05295
Zhu, Y., He, X., and Mo, Y. (2016). First Principles Study on Electrochemical and Chemical Stability of Solid Electrolyte-Electrode Interfaces in All-Solid-State Li-Ion Batteries. J. Mater. Chem. A. 4 (9), 3253–3266. doi:10.1039/C5TA08574H
Keywords: electrochemical stability window, potentiostatic intermittent titration technique, spark plasma sintering, solid electorolyte, all-solid-state lithium batteries, grand potential phase diagram, NASICON
Citation: Benabed Y, Rioux M, Rousselot S, Hautier G and Dollé M (2021) Assessing the Electrochemical Stability Window of NASICON-Type Solid Electrolytes. Front. Energy Res. 9:682008. doi: 10.3389/fenrg.2021.682008
Received: 17 March 2021; Accepted: 03 May 2021;
Published: 27 May 2021.
Edited by:
Chi Chen, University of California, San Diego, United StatesReviewed by:
Weike Ye, University of California, San Diego, United StatesXingyu Guo, University of California, San Diego, United States
Liqiang Mai, Wuhan University of Technology, China
Copyright © 2021 Benabed, Rioux, Rousselot, Hautier and Dollé. This is an open-access article distributed under the terms of the Creative Commons Attribution License (CC BY). The use, distribution or reproduction in other forums is permitted, provided the original author(s) and the copyright owner(s) are credited and that the original publication in this journal is cited, in accordance with accepted academic practice. No use, distribution or reproduction is permitted which does not comply with these terms.
*Correspondence: Mickaël Dollé, bWlja2FlbC5kb2xsZUB1bW9udHJlYWwuY2E=