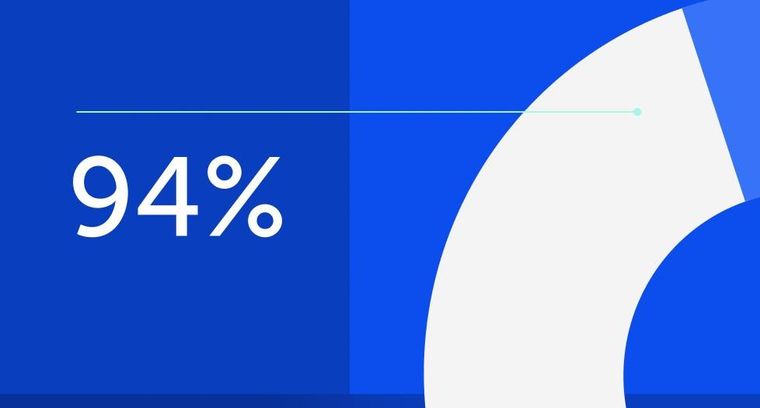
94% of researchers rate our articles as excellent or good
Learn more about the work of our research integrity team to safeguard the quality of each article we publish.
Find out more
BRIEF RESEARCH REPORT article
Front. Energy Res., 15 April 2021
Sec. Electrochemical Energy Storage
Volume 9 - 2021 | https://doi.org/10.3389/fenrg.2021.671345
This article is part of the Research TopicEmerging Technologies for Safe Rechargeable Energy StorageView all 4 articles
Graphene has long been envisioned as a promising material in areas, such as energy storage, electromagnetic shielding, and electrochemical sensor. However, the fabrication of graphene is complicated, time-consuming, and hazardous to environment, and thus can hardly realize industrialization. Although the exfoliation of graphite through electrochemical method was believed as an efficient and green approach, the intense current and non-protective action usually lead to the total destruction of the integralgraphitic electrode. In this work, the graphite foil was well-exfoliated into few-layered graphene with proper electrolyte compositions and electrochemical technique. Moreover, the original three-dimensional (3D) integrity of graphite foil can be maintained with the assistance of space-confined exfoliation strategy. The exfoliation process was systematically investigated in terms of electrolyte, applied potential, cation, and anion. The optimized sample exhibited an almost 8.0-folds of increment of double-layer capacitance in comparison with the pristine graphite foil. Eventually, the chemical simulations were employed to elaborate the mechanisms of advanced exfoliation. The space-confined exfoliation reported here is promising for scalable fabrication of 3D graphene materials.
Graphene, defined as a plane, hexagonal structure of carbon atoms is regarded as one of the most promising carbonaceous materials with superior properties, including mechanical stiffness, high conductivity, large specific surface area, and decent chemical tolerance (Castro Neto, 2010; Molitor et al., 2011; Avouris and Dimitrakopoulos, 2012). Consequently, graphene is considered as a promising candidate in many fields, in which interfacial reactions and ionic/electronic migrations are dominant, such as electrochemical reactions (Raccichini et al., 2015; Cao et al., 2020, 2021; Feng et al., 2020; Yi et al., 2021), biomedical detection (Yang et al., 2013), corrosion protection (Prasai et al., 2012), and so on. Nevertheless, the scalable production of graphene still confronted with a lot of challenges, including the consumption of energy, low yields, production of waste acid, manipulation risk, and easy restacking of graphene (Abdelkader et al., 2015; Chen et al., 2015). The top-to-down strategy was believed to be efficient in the fabrication of graphene through exfoliation of the bulk graphite monolith. Typically, the physical exfoliation can generate defect-free high-quality graphene; yet the low productive yields and small lateral sizes of graphene impede its widespread applications (Coleman, 2013; Leon et al., 2014). Chemical exfoliation, represented by Hummers' method can be utilized to massively produce graphene; however, the strong oxidant involved in the process induces numerous defects on graphene, and meanwhile emits hazardous pollutants into the environment (Dreyer et al., 2010).
Electrochemical exfoliation, a novel top-to-down exfoliation strategy has attracted a great attention recently, owing to the high efficiency, low-cost, environmentally benign, and up-scalable production (Low et al., 2013). Typically, the graphitic materials are employed as the working electrode during the electrochemical exfoliation process. Upon application of certain potentials, the ions with opposite charges in the electrolyte are attracted to the graphitic electrode. The ions will intercalate into the galleries of graphite, if the ionic size is comparable with that of the distance of the graphite lattice (Yang et al., 2016). The π-π interactions between the graphitic interlayers dramatically decrease along with the intercalation-induced gallery expansion (Lei et al., 2017; Munuera et al., 2017). The overall exfoliated mechanisms are schematically illustrated in Supplementary Figure 1. It is worthy to note that the exfoliated graphite is mostly in the form of powder (Su et al., 2011; Li et al., 2016; Kamali, 2017; Ossonon and Belanger, 2017; Shi et al., 2018), implying the loss of the structural integrity of the graphitic electrode, which is unfavorable for the fabrication of free-standing functional materials.
In this work, we proposed a space-confined strategy to electrochemically exfoliate graphite foil into a three-dimensional (3D) conductive material. The systematic investigations involving electrolyte compositions, applied potentials, and categories of cations and anions, have been carried out to optimize the exfoliation results. After chemical simulation, we unveiled that Li+ complexed by propylene carbonate (PC) molecules will intercalate into graphite under proper potential. After confining the exfoliation inside the metallic gauze, the original overall 3D architecture of the graphite foil can be well-maintained, which enables the design of free-standing electrodes and stretchable carbonaceous aerogels. This work paves an avenue to fabricate 3D graphene materials with an advanced electrochemical exfoliation method.
Graphite foil with a thickness of 0.127 mm was purchased from Mineral Seal Corporation, AZ, USA. Tetrabutylammonium hydrogen sulfate (TBA HSO4), tetrabutylammoniumtetrafluoroborate (TBATFB), tetramethylammonium perchlorate (TMA ClO4), tetraethylammonium perchlorate (TEA ClO4), and tetrabutylammonium nitrate (TBA NO3) were all purchased from Alfa Aesar, MA, USA. Propylene carbonate (PC), N-methyl-2-pyrrolidone (NMP), tetrahydrofuran (THF), dimethyl formamide (DMF), acetonitrile, potassium nitrate (KNO3), ammonium hydroxide (NH3 H2O), lithium perchlorate (LiClO4), and sodium perchlorate (NaClO4) were purchased from Sinopharm Chemical Reagent, Shanghai, China.
Briefly, the graphite foil with a size of 0.5 × 1.0 cm2 was wrapped with a metallic gauze, followed by immersing into the electrolyte as the reactive section. Electrochemical exfoliation was carried out on a CHI660E electrochemical workstation with typical three-electrode system, wherein the Pt plate, graphite foil, and Ag/AgCl wire were used as the counter, working, and reference electrodes, respectively. Before the electrochemical exfoliation, the electrolyte was saturated with high purity N2, and the N2 was maintained over the whole process. The scan rate during the exfoliation was fixed at 20 mV s−1. The exfoliation results were mainly evaluated by the folds of increment of the double-layer capacitance derived from the integral areas of the cyclic voltammetry (CV) curves.
First of all, we performed the electrochemical exfoliation in an aqueous electrolyte containing 6.0 ml 0.1 M TBA HSO4 + 12.0 ml 0.2 M KNO3. An alternating potential was employed to proceed with the exfoliation at an interval of 20 s.
Various alternating potentials from ± 1.5 to ± 5.5 V were applied to trigger the electrochemical exfoliation in the aqueous electrolyte. Figure 1A shows that the integral areas of CV increase along with the absolute values of the alternating potentials. However, the trends of increment are very limited, if the alternating potentials are further enhanced above 3.5 V (Figure 1B). The folds of increment will be only ca. 4 (vs. pristine graphite foil) when the applied potential reached up to 5.5 V.
Figure 1. (A) The comparison of CV curves before and after applying the alternating potentials; (B) the folds of increment for CV areas as a function of alternating potentials.
It is well-known that the potential window of electrochemical reactions in aqueous solutions is very narrow owing to the facile decomposition of water with intense applied potential (Yang et al., 2017). As a consequence, the aqueous solutions should be replaced by the organic ones, if we would like to further enhance the applied potential. Herein, the NMP was used as the organic solvent, in which 0.1 M TBATFB was dissolved. The period of the applied potential was fixed at 1,000 s. Supplementary Figure 2A displays that the graphite foil cannot be well-exfoliated until the potential lowers down to −1.8 V. It is worthy to note that the graphite foil underwent partial decomposition, if the potential further went down to −2.0 V (Supplementary Figure 2B).
From the aforementioned analysis, the potential of −1.8 V was believed to be the proper potential in this NMP electrolyte system. As a result, we fixed the exfoliation potential of −1.8 V and investigated the double-layer capacitance along with the time. Supplementary Figure 3A shows that the areas of the CV curves increase along with the exfoliation time. The CV curves can still retain a rectangle-like shape and can even prolong the exfoliation above 8,000 s. Supplementary Figure 3B reveals the obvious enhancement of CV areas at the beginning of 400 s period. However, the increment of CV areas is very limited after 800 s, implying that the exfoliation in this system cannot achieve a substantial exposure of new graphitic surface area or even further prolong the exfoliation time. At last, the CV area of the exfoliated sample reaches the 4.02-folds of increment compared with the pristine graphite foil.
Inspired by the Li-PC intercalation system proposed by Wang et al. (2011), the electrolyte was prepared by dissolving LiClO4 into a PC solvent. A CV process was judiciously designed to proceed with the exfoliation mildly, avoiding the departure of the graphite from the electrode. The potential window of the CV was set at a relatively wide range from −3.0 V to 0.3 V at a scan rate of 20 mV s−1 (Figure 2A). There appears a significant anodic peak of the CV curve at around −1.6 V (Figure 2A), wherein the graphite foil underwent a great expansion inside the electrolyte (Figure 2B). The over expansion of the graphite foil leads to partial delamination of the graphitic electrode. Therefore, the graphite foil was wrapped by a metallic gauze for avoiding excessive expansion (Figure 2C). Notably, the metallic gauze can not only maintain sufficient electrolyte infiltration of graphite foil but also facilitate the efficient electronic transportation through the highly conductive metallic shells, which is highly desirable for electrochemical exfoliation. As a result, we obtained a well-defined 3D graphitic hydrogel (Figure 2D). Figures 2E,F display the microstructures of the graphite foil before and after exfoliation, respectively. It is clear that the original stacked graphitic sheets are individually separated after the aforementioned electrochemical exfoliation. Supplementary Figure 4 shows the comparison of X-ray diffraction (XRD) patterns of graphite foil before and after electrochemical exfoliation. The dramatical decline of the (002) peaks after exfoliation indicates a successful delamination of few-layered graphene nanosheets. Just like the pristine graphite foil, the exfoliated one contains the elements including only carbon and oxygen (Supplementary Figure 5). The Raman spectra shown in Supplementary Figure 6 reveals that the D band of the pristine graphite foil is almost negligible, while the D bands that emerge after electrochemical exfoliation indicate that some of the graphitic defects are introduced during the exfoliation process.
Figure 2. (A) The CV curves designed for electrochemical exfoliation; (B) the exfoliated graphitic materials prepared with this CV method; (C) the electrochemical exfoliation conducted with the space confined strategy; (D) the exfoliated graphitic hydrogel obtained by the electrochemical exfoliation by wrapping the metallic gauze; graphite foil (E) before and (F) after exfoliation.
We further investigated the effects of LiClO4concentration on the electrochemical exfoliation. Figures 3A–F show the comparison of the CV curves before and after exfoliation with LiClO4 ranging from 20 to 70 mg ml−1. We can clearly see that the double-layer capacitances were evidently enhanced after exfoliation in each of the concentration. Figure 3G reveals that the value of folds of increment elevates initially and then goes down after 50 mg ml−1. After optimization of the LiClO4 concentration, the folds of increment of the double-layer capacitance can reach to a high value of ca. 8.0.
Figure 3. (A–F) Comparison of electrochemical exfoliation for graphite foil with various concentrations of LiClO4 in PC solvents; (G) the folds of increment of the double-layer capacitance along with the concentration of LiClO4.
Since LiClO4 presents significant effects on the exfoliation of graphite foil, we tried to search for other electrolyte compositions with various cations and anions. Herein, PC will be still acting as the solvent, and the cation is fixed to the typical TBA+. The areas of CV curves before and after exfoliation show very tiny differences with the anions of TFB−, , , and , implying the limited effects of anions on the exfoliation behaviors (Supplementary Figure 7).
We also investigated the effects of cations toward electrochemical exfoliation when the anion is fixed as . Supplementary Figure 8 shows that the Li+ exhibits a predominant advantage for the enhancement of the double-layer capacitance, with a fold of increment approaching 8.0. However, the other cations investigated present very low values (below 2.0) even for Na which is the analogous element of Li. The huge differences between Li and Na, including the atomic size, electronegativity, and reduction potential give rise to different contributions to the exfoliation efficiency.
In order to investigate the exfoliation mechanism of LiClO4 in PC solvent, the chemical simulations have been performed for the calculation of the interaction energies of the optimized configurations. Figure 4 reveals the Li-PC complex configurations before and after simulative optimization. In contrast to the original value of 0.2258 nm, the bond distance between O7 and Li11 was shortened to 0.1, 7,622 nm after optimization, owing to the as-formed strong interaction.
Figure 4. Li-PC complex configurations (A) before and (B) after optimization through chemical simulation method.
The interaction energy was calculated to determine the stability of the Li-PC complex and expressed as follows:
wherein, ΔE represents the interaction energy, while the ELi·PC,ELi and EPC represent the single-point energies of Li-PC system, Li+ ions, and PC molecules, respectively. The interaction energy (ΔE) was calculated to be −203.87375 kJ mol−1, which enables the formation of stable Li-PC complex. It is worthy to note that the positively charged Li-PC complex can intercalate into the galleries of the graphite in case of applying a negative potential, and the graphitic materials will be well-exfoliated under repeated intercalation and deintercalation cycles.
In this work, we proposed a space-confined electrochemical strategy to exfoliate the graphitic materials with the merits of highly efficient, environmentally benign, and up-scalable production. A systematic investigation, in terms of applied potentials, periods, and electrolyte categories has been performed to search for the most effective exfoliation conditions. Eventually, the Li-PC electrolyte system was screened as the optimal complex, with which the exfoliated graphite foil can deliver ca. 8.0-folds of increments of the double-layer capacitance compared with the pristine one. Besides, the graphite foil can still maintain the overall 3D architecture after electrochemical exfoliation with the space-confined method. The mechanism of exfoliation in Li-PC electrolyte was well-elaborated via chemical simulation. The strong interaction between Li+ ions and PC molecules was deemed to form a Li-PC complex, and thereby intercalated integrally into the interlayers of the graphitic materials. This work paves an avenue to fabricate the 3D exfoliated graphite with advanced electrochemical strategy.
The raw data supporting the conclusions of this article will be made available by the authors, without undue reservation.
YH: writing the original draft, review and editing, data curation, and formal analysis. XX: writing the original draft, data curation, and visualization. YW: investigation and data curation. WZhu: conceptualization, supervision, writing, review, and editing. WZhua: data curation and visualization. MS: conceptualization, funding acquisition, project administration, and supervision. All authors contributed to the article and approved the submitted version.
This work was supported by National Natural Science Foundation of China (51702162 and 21703194), Natural Science Foundation of Jiangsu Province (BK20171169), the Natural Science Foundation of the Jiangsu Higher Education Institutions of China (19KJA430020), and the College Blue Project of Jiangsu Province.
The authors declare that the research was conducted in the absence of any commercial or financial relationships that could be construed as a potential conflict of interest.
The Supplementary Material for this article can be found online at: https://www.frontiersin.org/articles/10.3389/fenrg.2021.671345/full#supplementary-material
Abdelkader, A. M., Cooper, A. J., Dryfe, R. A. W., and Kinloch, I. A. (2015). How to get between the sheets: a review of recent works on the electrochemical exfoliation of graphene materials from bulk graphite. Nanoscale 7, 6944–6956. doi: 10.1039/C4NR06942K
Avouris, P., and Dimitrakopoulos, C. (2012). Graphene: synthesis and applications. Mater. Today 15, 86–97. doi: 10.1016/S1369-7021(12)70044-5
Cao, J., Deng, L., Wang, X., Li, W., Xie, Y., Zhang, J., et al. (2020). Stable lithium metal anode achieved by in situ grown CuO nanowire arrays on Cu foam. Energy Fuels 34, 7684–7691. doi: 10.1021/acs.energyfuels.0c01180
Cao, J., Xie, Y., Li, W., Wang, X., Yang, Y., Zhang, Q., et al. (2021). Rationally optimized carbon fiber cloth as lithiophilic host for highly stable Li metal anodes. Mater. Today Energy 20:100663. doi: 10.1016/j.mtener.2021.100663
Castro Neto, A. H. (2010). The carbon new age. Mater. Today 13, 12–17. doi: 10.1016/S1369-7021(10)70029-8
Chen, C.-H., Yang, S.-W., Chuang, M.-C., Woon, W.-Y., and Su, C.-Y. (2015). Towards the continuous production of high crystallinity graphene via electrochemical exfoliation with molecular in situ encapsulation. Nanoscale 7, 15362–15373. doi: 10.1039/C5NR03669K
Coleman, J. N. (2013). Liquid exfoliation of defect-free graphene. Acc. Chem. Res. 46, 14–22. doi: 10.1021/ar300009f
Dreyer, D. R., Park, S., Bielawski, C. W., and Ruoff, R. S. (2010). The chemistry of graphene oxide. Chem. Soc. Rev. 39, 228–240. doi: 10.1039/B917103G
Feng, Y., Chen, S., Wang, J., and Lu, B. (2020). Carbon foam with microporous structure for high performance symmetric potassium dual-ion capacitor. J. Energy Chem. 43, 129–138. doi: 10.1016/j.jechem.2019.08.013
Kamali, A. R. (2017). Scalable fabrication ofhighly conductive 3D graphene by electrochemical exfoliation of graphite in molten NaCl under Ar/H2 atmosphere. J. Indust. Eng. Chem. 52, 18–27. doi: 10.1016/j.jiec.2017.03.013
Lei, H., Tu, J., Yu, Z., and Jiao, S. (2017). Exfoliation mechanism of graphite cathode in ionic liquids. ACS Appl. Mater. Interfaces 9, 36702–36707. doi: 10.1021/acsami.7b03306
Leon, V., Rodriguez, A. M., Prieto, P., Prato, M., and Vazquez, E. (2014). Exfoliation of graphite with triazinederivatives under ball-milling conditions: preparation of few-layer graphene via selective noncovalent interactions. ACS Nano 8, 563–571. doi: 10.1021/nn405148t
Li, C., Xu, Y.-T., Zhao, B., Jiang, L., Chen, S.-G., Xu, J.-B., et al. (2016). Flexible graphene electrothermal films made from electrochemically exfoliated graphite. J. Mater. Sci. 51, 1043–1051. doi: 10.1007/s10853-015-9434-x
Low, C. T. J., Walsh, F. C., Chakrabarti, M. H., Hashim, M. A., and Hussain, M. A. (2013). Electrochemical approaches to the production of graphene flakes and their potential applications. Carbon 54, 1–21. doi: 10.1016/j.carbon.2012.11.030
Molitor, F., Guettinger, J., Stampfer, C., Droescher, S., Jacobsen, A., Ihn, T., et al. (2011). Electronic properties of graphene nanostructures. J. Phys. Condens. Matter 23:243201. doi: 10.1088/0953-8984/23/24/243201
Munuera, J. M., Paredes, J. I., Villar-Rodil, S., Martinez-Alonso, A., and Tascon, J. M. D. (2017). A simple strategy to improve the yield of graphene nanosheets in the anodic exfoliation of graphite foil. Carbon 115, 625–628. doi: 10.1016/j.carbon.2017.01.038
Ossonon, B. D., and Belanger, D. (2017). Functionalization of graphene sheets by the diazonium chemistry during electrochemical exfoliation of graphite. Carbon 111, 83–93. doi: 10.1016/j.carbon.2016.09.063
Prasai, D., Tuberquia, J. C., Harl, R. R., Jennings, G. K., and Bolotin, K. I. (2012). Graphene: corrosion-inhibiting coating. ACS Nano 6, 1102–1108. doi: 10.1021/nn203507y
Raccichini, R., Varzi, A., Passerini, S., and Scrosati, B. (2015). The role of graphene for electrochemical energy storage. Nat. Mater. 14, 271–279. doi: 10.1038/nmat4170
Shi, P. C., Guo, J. P., Liang, X., Cheng, S., Zheng, H., Wang, Y., et al. (2018). Large-scale production of high-quality graphene sheets by a non-electrified electrochemical exfoliation method. Carbon 126, 507–513. doi: 10.1016/j.carbon.2017.10.071
Su, C.-Y., Lu, A.-Y., Xu, Y., Chen, F.-R., Khlobystov, A. N., and Li, L.-J. (2011). High-quality thin graphene films from fast electrochemical exfoliation. ACS Nano 5, 2332–2339. doi: 10.1021/nn200025p
Wang, J., Manga, K. K., Bao, Q., and Loh, K. P. (2011). High-yield synthesis of few-layer graphene flakes through electrochemical expansion of graphite in propylene carbonate electrolyte. J. Am. Chem. Soc. 133, 8888–8891. doi: 10.1021/ja203725d
Yang, S., Lohe, M. R., Muellen, K., and Feng, X. (2016). New-generation graphene from electrochemical approaches: production and applications. Adv. Mater. 28, 6213–6221. doi: 10.1002/adma.201505326
Yang, S., Ricciardulli, A. G., Liu, S., Dong, R., Lohe, M. R., Becker, A., et al. (2017). Ultrafast delamination of graphite into high-quality graphene using alternating currents. Angew. Chem. Int. Ed. 56, 6669–6675. doi: 10.1002/anie.201702076
Yang, Y. Q., Asiri, A. M., Tang, Z. W., Du, D., and Lin, Y. H. (2013). Graphene based materials for biomedical applications. Mater. Today 16, 365–373. doi: 10.1016/j.mattod.2013.09.004
Keywords: graphene, industrialization, electrochemical exfoliation, graphite foil, electrolyte, chemical simulation
Citation: Huang Y, Xu X, Wang Y, Zhu W, Zhuang W and Song M (2021) Construction of Three-Dimensional Conductive Graphitic Architecture by Highly Efficient Electrochemical Exfoliation. Front. Energy Res. 9:671345. doi: 10.3389/fenrg.2021.671345
Received: 23 February 2021; Accepted: 12 March 2021;
Published: 15 April 2021.
Edited by:
Xinghui Wang, Fuzhou University, ChinaCopyright © 2021 Huang, Xu, Wang, Zhu, Zhuang and Song. This is an open-access article distributed under the terms of the Creative Commons Attribution License (CC BY). The use, distribution or reproduction in other forums is permitted, provided the original author(s) and the copyright owner(s) are credited and that the original publication in this journal is cited, in accordance with accepted academic practice. No use, distribution or reproduction is permitted which does not comply with these terms.
*Correspondence: Ming Song, bWluZ3NAeHppdC5lZHUuY24=; Wenyou Zhu, end5b3VAeHppdC5lZHUuY24=
Disclaimer: All claims expressed in this article are solely those of the authors and do not necessarily represent those of their affiliated organizations, or those of the publisher, the editors and the reviewers. Any product that may be evaluated in this article or claim that may be made by its manufacturer is not guaranteed or endorsed by the publisher.
Research integrity at Frontiers
Learn more about the work of our research integrity team to safeguard the quality of each article we publish.