- 1Deanship of Research, Prince Mohammad Bin Fahd University, Al Khobar, Saudi Arabia
- 2College of Engineering, Prince Mohammad Bin Fahd University, Al Khobar, Saudi Arabia
- 3Wahaj Investment L.L.C., Dubai, United Arab Emirates
Introduction
About 95% of the hydrogen presently produced is from natural gas and coal, and the remaining 5% is generated as a by-product from the production of chlorine through electrolysis1. In the hydrogen economy (Crabtree et al., 2004; Penner, 2006; Marbán and Valdés-Solís, 2007), hydrogen is produced entirely from renewable energy. The easiest approach to advance renewable energy production is through solar photovoltaic and electrolysis, a pathway of high technology readiness level (TRL) suffering, however, from two downfalls. First of all, electricity is already an energy carrier, and transformation with a penalty into another energy carrier, hydrogen, is, in principle, flawed. The second problem is that the efficiency of commercial solar panels is relatively low. The cadmium telluride (CdTe) thin-film solar cells have a solar energy conversion efficiency of 17%. Production of hydrogen using the current best processes for water electrolysis has an efficiency of ~70%. As here explained, the concentrated solar energy may be used to produce hydrogen using thermochemical water-splitting cycles at much global higher efficiency (fuel energy to incident sun energy). This research and development (R&D) effort is, therefore, undertaken to increase the TRL of this approach as a viable and economical option.
Thermochemical Water-Splitting Cycles
Solar thermochemical water-splitting cycles (TWSCs) use high-temperature solar heat to drive a series of reactions producing hydrogen with oxygen as a welcomed by-product (Safari and Dincer, 2020). The chemicals used are recycled, creating a closed-loop process utilizing only water as feedstock, plus solar heat. The simplest TWSC is a two-step process. The metal oxide redox reactions include one endothermic reaction and one exothermic reaction. The metal oxide is transformed first into a reduced-valence metal oxide plus oxygen.
The reduced-valence metal oxide then reacts with H2O producing H2, oxygen, and the initial metal oxide.
The metal oxide is, thus, recycled. These cycles require temperatures well above 1,500°C. A TWSC with more than two steps has been designed to deliver better performances at lower temperatures. The general electric sulfur–iodine (S-I) TWSC is the most renowned three-step TWSC (Schultz, 2003; Bhosale et al., 2019). It is made up of two endothermic steps and one intermediate exothermic step.
The intermediate step is exothermic, the other two endothermic. Sink et al. (2009) suggests 850–900°C for the endothermic reaction (3), 400–500°C for the endothermic reaction (5), and 100°C for the exothermic reaction (4). While even lower temperatures have been proposed (Russ, 2009), the efficiency of the cycle increases by increasing the temperature of the reaction (3). It is η~52% at a temperature of 900°C, and η~60% at a temperature of 1,000°C (Schultz, 2003). As a downfall, the environment is, however, corrosive on the reactor side, and the supply of high-temperature solar heat is also challenging. This cycle has been extensively studied (Norman et al., 1982; Anzieu et al., 2006; Vitart et al., 2006; Zhou et al., 2007; Cerri et al., 2010; Zhang et al., 2010; Liberatore et al., 2012; Park et al., 2019), with technological advances still needed. Opposite to direct solar thermochemical water splitting into an integrated receiver/reactor (Chueh et al., 2010) presently featuring a very low TRL, the TRL of the indirect solar thermochemical hydrogen production is medium.
Ayman Solar Concentrator
While the cycle was originally developed for nuclear heat, it is the use of solar heat that may revive the technology, thanks to improvements in solar concentrators' technologies, and the formulation of higher-temperature molten salts/liquid metal heat transfer fluids (HTF)/heat storage fluids (HSF). Thermal energy storage (TES) is mandatory for the dispatchability of electricity and continuous thermochemical hydrogen production.
To obtain such high temperatures, point-focusing solar concentrators should be used. Until recently, only two types of point solar concentrators are known and investigated. The first type is the parabolic dish, which can reach very high concentration ratios reaching very high temperatures at the focal point. The optical efficiency of the parabolic dish is high. Values from 78 to 89% were already achieved in the 1990s (Stine and Diver, 1994). A more recent study reported a 95% efficiency (Lovegrove et al., 2011). However, there are two disadvantages of the parabolic dish. The first is that its focal point is moving in space as the dish tracks the sun from sunrise to sunset. As such, the high-pressure and high-temperature HTF has to go through flexible pipes or rotary swivel joints to the storage tank, which is not practical at temperatures around 1,000°C. Also, high-temperature thermal storage of energy harvested from the parabolic dish is not practical. Moreover, the solid cross-sectional area of the parabolic dish results in high wind load on its structure pausing structural limitation on its size. Practically, no commercial parabolic dish with a diameter of more than 10 m is on the market. The other known point concentrator is the heliostat field and solar tower technology. Although this technology has a fixed focal point at the top of the tower, it has very low optical efficiency due to its heliostat tracking and reflecting concept. The beam-down variant with a fixed focal point at the ground is not any better. In theory, the optical efficiency is between 40 and 60%, even if some special design has delivered up to 63.9% efficiency (Wang et al., 2019), but in practice, it is even considerably less due to imperfect reflection over large distances. Moreover, the commercially available types can only reach temperatures below 600°C due to inaccuracies in the concentration procedures (receiver outlet temperature 565°C in Gemasolar, Ivanpah, and Crescent Dunes2). However, recently, Heliogen3 has utilized artificial intelligence (AI) technology to increase the concentration accuracy allowing temperatures up to 1,000°C to be reached at the receiver. Nonetheless, the technology still has low optical efficiency and is quite expensive as it is still in the R&D phase.
Recently, Al-Maaitah (2017, 2019) has developed a high flux solar concentrator with a lower focal point fixed to the ground composed of nested conical reflective rings resulting in high solar concentrations and temperatures well above 1,000°C (Al-Maaita, 20204). Since the focal point is fixed on the ground, then the high-temperature and high-pressure HTF can easily be conveyed through solid inexpensive pipes to the storage tank sitting at the ground underneath the receiver or next to it. A 10 m in diameter concentrator was built and tested in Masdar Institute Solar Platform in Abu Dhabi (UAE) where temperatures exceeding 1,000°C have been reached at the fixed focal point even at low DNI (Al-Maaita, 2020; see text footnote 4). One spherical or cylindrical receiver is used to heat HTF to the levels needed for the TWSC. The commercial name given to this concentrator is ASC (Ayman Solar Concentrator). The optical efficiency of the ASC is high and reaches 91% in practice, while it is 100% theoretically like the parabolic dish. Since the wind flows through its conical rings, the wind load of the ASC is about 10% that of the parabolic dish of the same dimensions. A 15-m ASC is designed and can be easily deployed, and a 25-m ASC is under design consideration. The system is modular, and many units can combine their inputs to a fixed storage tank to have a large storage capacity.
ASC With Thermal Energy Storage and Thermochemical Water-Splitting Cycles
Between the different HTFs being considered, one receiving attention is MgCl2-KCl (Ding et al., 2018; Polimeni et al., 2018; Xu et al., 2018; Kurley et al., 2019; Peng, 2019; Vidal and Klammer, 2019; Wu et al., 2019; Fernández and Cabeza, 2020a,b,c). MgCl2-KCl is 37.5% MgCl2 and 62.5% KCl. It can be used between 426°C and 1,412°C. Within this range of temperatures, cp varies between 0.989 and 1.092 kJ kg−1 K−1, ρ between 1,668.5 and 1,124.3 kg m−3, μ between 5.73 · 10−3 and 8.57 · 10−3 Pa s, λ between 0.46 and 0.36 W m−1 K−1, and h between 421,391 and 1,542,352 J kg−1. ρ·cp varies between 1,650.5 and 1,228.1 ρ·cp kJ m−3 K−1.
The sulfur-iodine (S-I) TWSC (i.e. Ross, 2009) is adopted. Other metal oxide three steps are investigated (Charvin et al., 2007; Bhosale, 2020).
The opportunity of using TLC components upstream of the TWSC section and synergy between applications (basically the same design of concentrator and storage applies for the production of electricity or hydrogen) reduce the total cost of R&D, more intense on the TWSC side. Efficiency and durability of reactant materials, and efficient and robust reactor designs compatible with high temperatures and corrosive environments are major challenges.
Figure 1 presents a scheme of the upstream ASC with TES, plus the downstream TWSC. The metallic lenses focus the solar energy on the point receiver. The cold HFT from the cold TES reservoir passes through the receiver to reach the hot TES reservoir. The hot HTF then supplies the thermal energy needed by the TWSC. While the design of the ASC and TES is relatively simple, the design of the TWSC is much more complicated, requiring multiple different reactor sections and heat exchangers. The waste heat may also be recovered.
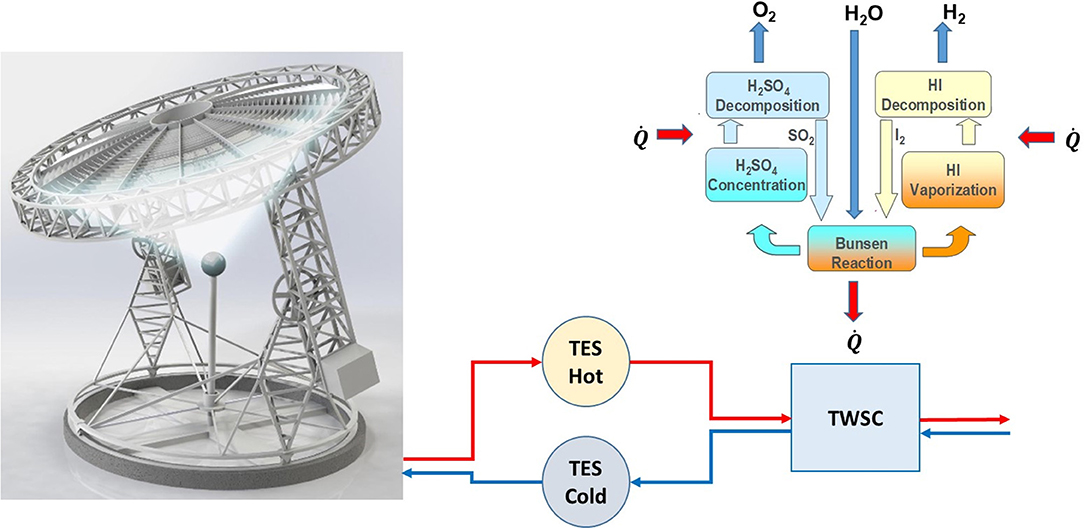
Figure 1. Scheme of the upstream Ayman Solar Concentrator (ASC) with thermal energy storage (TES), plus the downstream thermochemical water-splitting cycle (TWSC).
The ASC has a much better collection efficiency compared with a field of heliostat and a tower receiver [91% (Al-Maaita, 2020; see text footnote 4) compared with, only in special cases, 63.9% (Wang et al., 2019)]. Storage can be done at high temperatures (1,100°C within easy reach). Importantly, it is much less costly to have a demonstration pilot plant than in a concentrated solar power, solar tower, and plant (Boretti et al., 2019). Moreover, the ASC is being developed anyway for high-temperature solar heat production, and thermal energy storage, coupled to continuous electricity production through high-temperature power cycles.
Opposite to the direct solar thermochemical water splitting into an integrated receiver/reactor (Chueh et al., 2010), the proposed solution permits a much more complex, but less challenging, chemical plant, not subjected to thermal transients, receiving heat and not direct solar energy, and without the extremely tight space constraints making the catalytic chemical processes hard to progress.
Discussion and Conclusions
Green hydrogen is booming, and the preferred technology is solar photovoltaic plus electrolysis5. The projected cost is 1.5 US$ per kg of H2 by 2050. By using about the same solar concentrator and collector, plus TES, of high temperature concentrated solar power to feed a TWSC, there is the opportunity to achieve even better costs of 1–1.5 US$ per kg and much earlier. Kayfeci et al. (2019) suggests a cost of 2.17–2.63 US$ per kg of H2 from nuclear thermolysis. Novel solar concentrators may produce thermal energy at a cost less than the cost of nuclear thermal energy. This cost is competitive with the current steam reforming of methane without CO2 capture and storage (Boretti, 2020).
ASC with TES may deliver solar heat continuously at temperatures up to 1,100°C. TWSC three steps, originally developed for nuclear hydrogen production, may return popular for solar thermal.
Synergies with power generation are beneficial. The upstream section of solar energy collection and storage is unaltered. The downstream section is specialized for electricity production, hydrogen production, or other high-temperature thermal processes. The three-step S-I already was developed up to a medium-high technology readiness level (TRL) (Boretti, 2021) when the interest in nuclear hydrogen dropped following the Fukushima Daiichi nuclear accident of 2011. By assuming the development of a high-temperature solar concentrator and collector, plus TES will progress independently for power generation, the only research and development effort is the one needed to further optimize the TWSC.
Author Contributions
AB: conceptualization. AB, JN, and AA-M: writing—original draft preparation and writing—review and editing. AA-M and AB: visualization. All authors have read and agreed to the published version of the manuscript.
Conflict of Interest
AA-M is one of the owners of the company Wahaj Investment L.L.C.
The remaining authors declare that the research was conducted in the absence of any commercial or financial relationships that could be construed as a potential conflict of interest.
Footnotes
1. ^www.irena.org/-/media/Files/IRENA/Agency/Publication/2019/Sep/IRENA_Hydrogen_2019.pdf.
2. ^solarpaces.nrel.gov/by-technology/power-tower.
3. ^heliogen.com.
4. ^www.cmimarseille.org/menacspkip/wp-content/uploads/2018/09/8.B.-Wahaj-Invest-Ayman-Al-Maaitah.pdf.
5. ^hydrogencouncil.com/wp-content/uploads/2021/02/Hydrogen-Insights-2021-Report.pdf.
References
Al-Maaita, A. A. (2020). “Design and demonstration of a 10-meter metallic reflector-based fresnel lens, with lower focal point fixed to the ground,” in Proceedings of the 26th SolarPACES Conference, Sept. 28-October 2nd, 2020. Available online: cms2020.solarpaces-conference.org/file/display_attachment/ab37be5b91c0133a162b848b87bcc27?filename=Ayman+Al-Maaitah+-+Paper.pdf (accessed February 01, 2021).
Al-Maaitah, A. A. (2017). Method and Apparatus for Tracking and Concentrating Electromagnetic Waves Coming From a Moving Source to a Fixed Focal Point. U.S. Patent No. 9,772,121B1, Alexandria, VA: United States Patent and Trademark Office (USPTO).
Al-Maaitah, A. A. (2019). Systemfor Collecting Radiant Energy with a Non-Imaging Solar Concentrator. U.S. Patent No. US 10,436,182B2, Alexandria, VA: United States Patent and Trademark Office (USPTO).
Anzieu, P., Carles, P., Duigou, A. L., Vitart, X., and Lemort, F. (2006). The sulphur–iodine and other thermochemical process studies at CEA. Int. J. Nuclear Hydrog. Product. Appl. 1, 144–153. doi: 10.1504/IJNHPA.2006.011248
Bhosale, R. R. (2020). A novel three-step GeO2/GeO thermochemical water splitting cycle for solar hydrogen production. Int. J. Hydrog. Energy 45, 5816–5828. doi: 10.1016/j.ijhydene.2019.05.190
Bhosale, R. R., Takalkar, G., Sutar Kumar, A., AlMomani, F., and Khraisheh, M. (2019). A decade of ceria based solar thermochemical H2O/CO2 splitting cycle. Int. J. Hydrog. Energy 44, 34–60. doi: 10.1016/j.ijhydene.2018.04.080
Boretti, A. (2020). Perspectives of production of hydrogen for export from wind and solar energy, natural gas, and coal in Australia. Int. J. Hydrogen Energy 47, 3899–3904.
Boretti, A. (2021). Technology readiness level of solar thermochemical splitting cycles. ACS Energy Lett. 6, 1170–1174. doi: 10.1021/acsenergylett.1c00181
Boretti, A., Castelletto, S., and Al-Zubaidy, S. (2019). Concentrating solar power tower technology: present status and outlook. Nonl. Eng. 8, 10–31. doi: 10.1515/nleng-2017-0171
Cerri, G., Salvini, C., Corgnale, C., Giovannelli, A., Manzano, D. D. L., Martinez, A. O., et al. (2010). Sulfur–Iodine plant for large scale hydrogen production by nuclear power. Int. J. Hydrog. Energy 35, 4002–4014. doi: 10.1016/j.ijhydene.2010.01.066
Charvin, P., Abanades, S., Lemort, F., and Flamant, G. (2007). Hydrogen production by three-step solar thermochemical cycles using hydroxides and metal oxide systems. Energy Fuels 21, 2919–2928. doi: 10.1021/ef0701485
Chueh, W. C., Falter, C., Abbott, M., Scipio, D., Furler, P., Haile, S. M., et al. (2010). High-flux solar-driven thermochemical dissociation of CO2 and H2O using nonstoichiometric ceria. Science 330, 1797–1801. doi: 10.1126/science.1197834
Crabtree, G. W., Dresselhaus, M. S., and Buchanan, M. V. (2004). The hydrogen economy. Phys. Today 57, 39–44. doi: 10.1063/1.1878333
Ding, W., Bonk, A., and Bauer, T. (2018). Corrosion behavior of metallic alloys in molten chloride salts for thermal energy storage in concentrated solar power plants: a review. Front. Chem. Sci. Eng. 12, 564–576. doi: 10.1007/s11705-018-1720-0
Fernández, A. G., and Cabeza, L. F. (2020a). Corrosion evaluation of eutectic chloride molten salt for new generation of CSP plants. Part 1: thermal treatment assessment. J. Energy Storage 27, 101125. doi: 10.1016/j.est.2019.101125
Fernández, A. G., and Cabeza, L. F. (2020b). Corrosion evaluation of eutectic chloride molten salt for new generation of CSP plants. Part 2: materials screening performance. J Energy Storage 29:101381. doi: 10.1016/j.est.2020.101381
Fernández, A. G., and Cabeza, L. F. (2020c). Cathodic protection using aluminum metal in chloride molten salts as thermal energy storage material in concentrating solar power plants. Appl. Sci. 10:3724. doi: 10.3390/app10113724
Kayfeci, M., Keçebaş, A., and Bayat, M. (2019). “Hydrogen production,” in Solar Hydrogen Production (Cambridge, MA: Academic Press), 45–83. doi: 10.1016/B978-0-12-814853-2.00003-5
Kurley, J. M., Halstenberg, W., McAlister, A., Raiman, S., Dai, S., and Mayes, R. T. (2019). Enabling chloride salts for thermal energy storage: implications of salt purity. RSC Adv. 9, 25602–25608. doi: 10.1039/C9RA03133B
Liberatore, R., Lanchi, M., Caputo, G., Felici, C., Giaconia, A., Sau, S., et al. (2012). Hydrogen production by flue gas through sulfur–iodine thermochemical process: economic and energy evaluation. Int. J. Hydrog. Energy 37, 8939–8953. doi: 10.1016/j.ijhydene.2012.02.163
Lovegrove, K., Burgess, G., and Pye, J. (2011). A new 500 m2 paraboloidal dish solar concentrator. Solar Energy 85, 620–626. doi: 10.1016/j.solener.2010.01.009
Marbán, G., and Valdés-Solís, T. (2007). Towards the hydrogen economy? Int. J. Hydrog. Energy 32, 1625–1637. doi: 10.1016/j.ijhydene.2006.12.017
Norman, J. H., Mysels, K. J., Sharp, R., and Williamson, D. (1982). Studies of the sulfur-iodine thermochemical water-splitting cycle. Int. J. Hydrog. Energy 7, 545–556. doi: 10.1016/0360-3199(82)90035-0
Park, J., Lee, S. Y., and Lee, I. B. (2019). Study of alternative reactor–separator network in bunsen process of sulfur-iodine cycle for hydrogen production. J. Chem. Eng. Jap. 52, 638–649. doi: 10.1252/jcej.18we078
Peng, Y. (2019). High-Temperature Corrosion Study of Alloys in Molten MgCl2-KCl Eutectic Salt. Tuscaloosa, AL: The University of Alabama.
Penner, S. S. (2006). Steps toward the hydrogen economy. Energy 31, 33–43. doi: 10.1016/j.energy.2004.04.060
Polimeni, S., Binotti, M., Moretti, L., and Manzolini, G. (2018). Comparison of sodium and KCl-MgCl2 as heat transfer fluids in CSP solar tower with sCO2 power cycles. Sol Energy 162, 510–524. doi: 10.1016/j.solener.2018.01.046
Russ, B. (2009). Sulfur Iodine Process Summary for the Hydrogen Technology Down-Selection. Process Performance Package [No. INL/EXT-12-25772]. Idaho Falls, ID: Idaho National Laboratory [INL].
Safari, F., and Dincer, I. (2020). A review and comparative evaluation of thermochemical water splitting cycles for hydrogen production. Energy Convers. Manage. 205:112182. doi: 10.1016/j.enconman.2019.112182
Schultz, K. (2003). Thermochemical Production of Hydrogen from Solar and Nuclear Energy. Presentation to the Stanford Global Climate and Energy Project. Available online at: web.stanford.edu/group/gcep/pdfs/hydrogen_workshop/Schultz.pdf (accessed February 01, 2021).
Sink, C., Sakaba, N., Yvon, P., Shin, Y. J., Dominguez, M. T., and Suppiah, S. (2009). “For the very high temperature reactor system,” in GIF Symposium (Paris), 109.
Stine, W. B., and Diver, R. B. (1994). A Compendium of Solar Dish/Stirling Technology. SAND-93-7026. Albuquerque, NM: Sandia National Laboratories. doi: 10.2172/10130410
Vidal, J. C., and Klammer, N. (2019). “Molten chloride technology pathway to meet the US DOE sunshot initiative with Gen3 CSP,” in AIP Conference Proceedings, Vol. 2126 (Melville, NY: AIP Publishing LLC). doi: 10.1063/1.5117601
Vitart, X., Le Duigou, A., and Carles, P. (2006). Hydrogen production using the sulfur–iodine cycle coupled to a VHTR: an overview. Energy Conv. Manage. 47, 2740–2747. doi: 10.1016/j.enconman.2006.02.010
Wang, W. Q., Qiu, Y., Li, M. J., Cao, F., and Liu, Z. B. (2019). Optical efficiency improvement of solar power tower by employing and optimizing novel fin-like receivers. Energy Convers. Manage. 184, 219–234. doi: 10.1016/j.enconman.2018.12.029
Wu, F., Roy, S., Ivanov, A. S., Gill, S. K., Topsakal, M., Dooryhee, E., et al. (2019). Elucidating ionic correlations beyond simple charge alternation in molten MgCl2-KCl mixtures. J. Phys. Chem. Lett. 10, 7603–7610. doi: 10.1021/acs.jpclett.9b02845
Xu, X., Wang, X., Li Li, Y., Hao, Q., Xiao, B., Elsentriecy, H., et al. (2018). Experimental test of properties of KCl–MgCl2 eutectic molten salt for heat transfer and thermal storage fluid in concentrated solar power systems. J. Sol. Energy Eng. 140:051011. doi: 10.1115/1.4040065
Zhang, P., Chen, S. Z., Wang, L. J., Yao, T. Y., and Xu, J. M. (2010). Study on a lab-scale hydrogen production by closed cycle thermo-chemical iodine–sulfur process. Int. J. Hydrog. Energy 35, 10166–10172. doi: 10.1016/j.ijhydene.2010.07.150
Keywords: concentrated solar energy, thermochemical water splitting cycles, hydrogen, thermal energy storage, S-I cycle
Citation: Boretti A, Nayfeh J and Al-Maaitah A (2021) Hydrogen Production by Solar Thermochemical Water-Splitting Cycle via a Beam Down Concentrator. Front. Energy Res. 9:666191. doi: 10.3389/fenrg.2021.666191
Received: 09 February 2021; Accepted: 10 March 2021;
Published: 14 May 2021.
Edited by:
Emanuela Mastronardo, University of Messina, ItalyReviewed by:
Francesco Rovense, Rey Juan Carlos University, SpainCopyright © 2021 Boretti, Nayfeh and Al-Maaitah. This is an open-access article distributed under the terms of the Creative Commons Attribution License (CC BY). The use, distribution or reproduction in other forums is permitted, provided the original author(s) and the copyright owner(s) are credited and that the original publication in this journal is cited, in accordance with accepted academic practice. No use, distribution or reproduction is permitted which does not comply with these terms.
*Correspondence: Alberto Boretti, deanshipofresearch@pmu.edu.sa