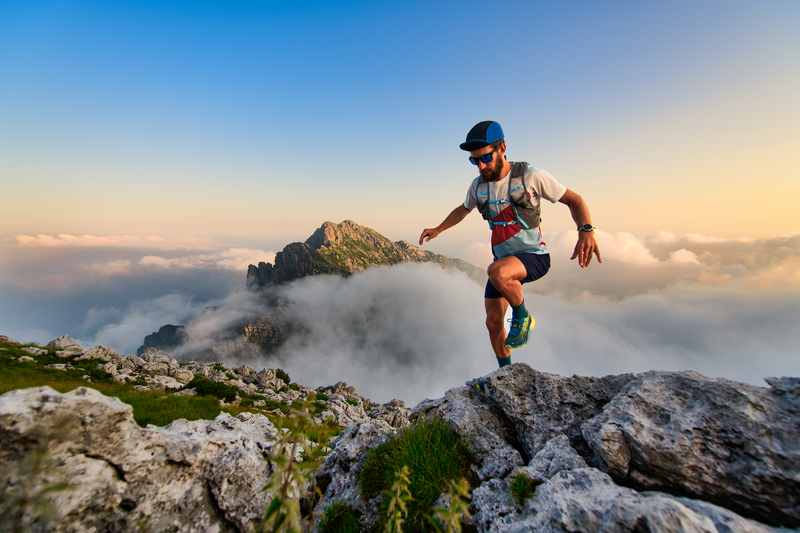
95% of researchers rate our articles as excellent or good
Learn more about the work of our research integrity team to safeguard the quality of each article we publish.
Find out more
ORIGINAL RESEARCH article
Front. Energy Res. , 09 April 2021
Sec. Hydrogen Storage and Production
Volume 9 - 2021 | https://doi.org/10.3389/fenrg.2021.653527
This article is part of the Research Topic Molten Carbonate Fuel Cells for Simultaneous CO2 Capture, Power Generation, and Hydrogen Production View all 8 articles
Here we report the results of a study on active surface species of a pristine and modified (Li-Na)2CO3 eutectic using in situ Raman spectroscopy technique. The effects of gas compositions, temperature, time, and alkaline earth have been systematically studied. The species of CO42–, HCO4–, and C2O52– are identified as the three major active species on the surface of (Li-Na)2CO3 eutectic by a combined Raman spectroscopy and theoretical density functional theory calculations. The results further reveal that CO42–, HCO4–, and C2O52– are preferably formed in the presence of O2, H2O, and high CO2 concentration. With the addition of Ba to the pristine (Li-Na)2CO3 eutectic, the Raman CO42–/HCO4– shifts become more pronounced.
Molten carbonate fuel cells (MCFCs) are a class of energy-efficient and low-emission power generators (Morita et al., 2002; Watanabe et al., 2006; Kawase, 2017). One of their unique features is the use of CO2 and O2 as the cathode gas. The mixture of CO2 and O2 is also a major component in flue gas produced by either coal-fired or natural-gas (NG)-fired power plants. A natural and logical question is if the flue gas can be directly utilized as the oxidant by MCFCs to produce additional power while mitigating the CO2 emission.
The concentration of CO2 emitted by coal-fired power plants is more than twice that emitted by NG-fired power plants, e.g., 10 vs. 4%. The current benchmark MCFCs can operate normally with a CO2 concentration higher than 10%; at lower CO2 concentrations, their performance is significantly limited by diffusion-related concentration polarization. To generate power from MCFCs using low CO2 concentration flue gas produced from NG-fired power plants, the existing MCFC cell/stack technology needs to be further improved to minimize the concentration polarization and meet the performance requirements. In addition, there is a significant amount of H2O, e.g., 10%, in NG flue gas. The effect of H2O on the MCFC performance is not well understood from early studies.
A recent result has confirmed that MCFCs exhibit a high concentration-polarization at low CO2 concentrations and surprisingly enhanced performance in the presence of H2O (Rosen et al., 2020). An electrochemical model based on active species peroxide-ion (O22–) and superoxide (O2–) has been proposed with satisfactory agreement with the experimental data (Cassir et al., 1993). Indeed, an independent Raman spectroscopy study has confirmed the existence of O22– on the surface of a Li2/K2CO3 eutectic (Chen et al., 2004).
A parallel conduction mechanism is also proposed for OH– as a working ion when H2O is present in the oxidant feed. From the water mass collected at both cathode and anode sides, it appears to suggest that the conduction mechanism of OH– in a MC matrix is real (Rosen et al., 2020). However, more experimental evidence related to active species to support water transport is needed.
Raman spectroscopy is a very sensitive technique to detect vibrational modes of C-O-C, C-O, and C = O bonds that are abundant in C-containing species such as CO32– and CO42– and are of interest to this research (Itoh et al., 2004; Mendoza et al., 2004). We have previously used Raman spectroscopy to probe the surface chemistry of an Ag-MC (Molten Carbonate) membrane operated under a flue gas condition containing O2 and CO2 to facilitate our understanding of why enhanced oxygen permeation was observed (Tong et al., 2016). We concluded from both experimental and theoretical data that LiCO4– was the active species on the Ag-MC membrane surface under simulated flue gas conditions and the extra oxygen in CO42– ligand relative to CO32– is the reason for the increased oxygen transport. The in situ Raman spectra of the Ag-MC membrane were collected by a LabRam/HR confocal Raman system (LabRam Invers, Horiba Jobin-Yvon) equipped with a 632.8 nm He-Ne laser and hot stage (Linkam TS1500, 0–1,500°C). From this work, we were able to identify that CO42– is an active species on the surface of an atomic layer deposition Al2O3-coated Ag-MC composite membrane and explain the phenomenon of the enhanced oxygen flux based on the percarbonate mechanism. In a similar work (Mendoza et al., 2004), we were also able to identify the active surface species as C2O52– with in situ Raman spectroscopy when a MC melt was exposed to a pure CO2 atmosphere.
These prior works demonstrate the versatility and capability of in situ Raman spectroscopy in probing active species on the surface of MC. Therefore, in this study, the in situ Raman spectroscopy technology was selected to identify the species of molten carbonate exposed to varies atmospheres at MCFC operating temperatures.
The molten carbonate compositions under this study were Li2CO3-Na2CO3 eutectic with a ratio of 52:48 mol% (MC), and 10 mol%-SrCO3 added MC (Sr-MC) and 10 mol%-BaCO3 added MC (Ba-MC). The reason for selecting Li2CO3-Na2CO3 eutectic was primarily based on the consideration of its higher ionic conductivity than other alkali carbonates eutectics. To prevent MC overflow at high temperatures, an Ag porous matrix was used to contain all MCs. The Ag porous matrix was prepared in the same way as in our previous study (Zhang et al., 2016). Briefly, Ag powders (99.9% metal basis, Alfa Aesar) were mixed with carbon black as a pore former in a volume ratio of 1:1 in ethanol. The dried power mixture was then pressed into pellets under 70 MPa, followed by sintering at 650°C for 2 h in air to remove the carbon pore former and achieve good mechanical strength. Then the porous Ag pellet was soaked into a MC at 650°C for 2 h in air, forming Ag/MC composite.
The Raman system used for this study is a Horiba Jobin-Yvon LabRAM HR800. It features a focal length of 800 mm, spectral length of 100–4,000 cm–1 with a spectral resolution up to 1.5 cm–1, accuracy of wavenumber of 1 cm–1 and spatial resolution up to 1 μm. We use the following operating parameters for Raman spectroscopy study on the surface of a MC:
• Wavelength: 300–1,800 cm–1
• Light source: 632 nm red
• Acquisition time: 20 s
• Accumulation: 1
• Hole: 500
• Filter: 100%.
The system is also adaptable to high-temperature subsystem for in situ measurements. The high temperature unit (or herein called hot stage) is model Linkam TS1500. The hot-stage is connected to a water-cooling system driven by a water circulation pump. The actual temperature was calibrated with the melting point of prepared MC (490°C), and temperature effect was studied at 575 and 625°C. Since the atmosphere effect is a focus of the study, we systematically varied the gas compositions over the MC at a temperature. Table 1 lists all the gas mixtures studied. The total gas flow was fixed at 50 sccm for all measurements.
The equilibrium geometries of species were fully optimized at the B3LYP/6-311G∗ level using the Gaussian 09 program package (Hehre, 2002; Frisch et al., 2009). To better understand the vibrational modes and figure out fingerprints of these species, Raman spectra were simulated using the B3LYP/6-311G∗ basis sets under the harmonic approximation at the same level of geometry optimization. Vibrational analysis showed that all the structures were at minima in the potential energy surface (no imaginary frequencies). Based on the Raman activity obtained from density functional theory (DFT) calculations, the Raman intensity was determined by the GaussSum software (O’Boyle et al., 2008).
Figure 1 compares the Raman spectra at 575°C in pure N2 (gas #1), 2% CO2 (gas #2), and 10% CO2 (gas #3) atmospheres. The lower intensity of the Raman spectra in 10% CO2 is due to the presence of Ag in the Ag-MC sample used to prevent the overflow of the MC. In the following studies, all Raman spectra were collected with the Ag-MC sample. As shown in the Figure 1, the main band at 1,072 cm–1 is assigned to symmetric stretching (v1) of O-C-O, where the bands at 707, 885, 1,391, 1,500, and 1,762 cm–1 correspond to the in-plane bending (v4) of C-O, out-of-plane bending (v2) of O-C-O, doubly degenerate asymmetric stretching (v3) of O-C-O, and the overtone of the out-of-plane bending mode (2v2) vibrations, respectively (Bates et al., 1972; Chen et al., 2002; Zhang et al., 2013). Clearly, there is no difference in these Raman shifts, implying that dry N2 and CO2 conditions do not invoke new species other than CO32–. Similar phenomenon was also observed in our previous study, where there is no new peak in the dry gas conditions with Ag-MC sample (Tong et al., 2016).
Figure 1. The Raman spectra of the MC/Ag-MC samples at 575°C in dry gas atmospheres with different CO2 concentration. (N2: gas #1, 2% CO2: gas #2, 10% CO2: gas #3).
The temperature and time effect on the Raman spectra of the Ag-MC samples in dry 10% CO2 atmosphere (gas #3) are compared in Figure 2. Figures 2A,B show the Raman spectra of the Ag-MC sample at 575 and 625°C, respectively. These results confirm that there is no difference in spectrum in dry gas at both temperatures.
Figure 2. The effects of temperature and time on Raman spectra of the Ag-MC sample in dry 10% CO2 gas (gas #3). (A) 575°C and (B) 625°C.
To investigate why steam promotes the MCFC performance, we also conducted the Raman spectroscopy of the Ag-MC sample in wet gas (10% H2O) with different CO2 concentrations. For an easy comparison, the Raman spectra in dry N2 is also shown in Figure 3. Some new shift between 400 and 607 cm–1 appear in wet CO2 atmospheres. To identify these new shifts, DFT calculations were performed. From the DFT calculations, the weak but new shifts at 409 and 567 cm–1 seem to relate to HCO4–, while the weak but new shifts at 607 cm–1 can be assigned to CO42–. These two species are observed in CO2 concentration gases from 2 to 45% CO2, while at high CO2-concentraiton, e.g., 90% CO2, C2O52– species appears at 455 cm–1. The observation of C2O52– species at high CO2 concentration is reasonable given the fact that CO2 dissolution into MC to form C2O52– by CO2+CO32– = C2O52– (Claes et al., 1996).
Figure 3. Raman spectra, along with DFT calculations, of the Ag-MC sample collected at 625°C in different CO2 concentration gas conditions: dry N2 (gas #1), 2% CO2 (gas #4), 9% CO2 (gas #5), 45% CO2 (gas #6), 90% CO2 (gas #7).
When the gas atmosphere changed from dry N2 to wet 2% CO2, the weak but new bands at 567 and 607 cm–1 seem to emerge, which correspond to HCO4– and CO42–, respectively. (Note: the wet 2% CO2 gas also contains 9% O2, see Table 1, gas #4). Then the intensity of the new bands slightly increased by increasing CO2 concentration to 9 and 45%. The formation mechanisms for the observed HCO4– and CO42– are (Zhang et al., 2014; Tong et al., 2016):
From these reactions, H2O is essential to form HCO4–, but not for CO42– species. However, it is interesting to see that CO42– appears in wet flue gas but not in dry flue gas (i.e., ∼10% CO2-10% O2), see Figure 2B. A possible reason is that the steam facilitates the dissolution of O2 into MC. From Figure 3, HCO4– and CO42– bands disappear and C2O52– band appear with further increasing CO2 concentration to 90% (no O2), which is consistent with our previous study (Zhang et al., 2013).
To further confirm that the formation of HCO4– and CO42– is highly influenced by the solubility of molecular oxygen, and therefore, the basicity in MCs. We measured the Raman spectra of alkaline earth-addition MC, since it has been reported that the alkaline earth metals can enhance the oxygen solubility of the molten carbonate (Scaccia and Frangini, 2009). At first, the Raman spectra of Ba- and Sr-added MC under in 45% CO2-10% O2-10% H2O-N2 atmosphere (gas #6) were collected and are shown in Figure 4. It seems that Sr-doped MC sample show similar new bands with the pristine MC sample. However, the intensities of HCO4– and CO42– bands of Ba-added MC samples are increased appreciably. In addition, a couple of more pronounced new peaks at ∼360 and 770 cm–1 are observed of the Ba-added sample. Compared to DFT-calculated Raman bands, it is determined that the new shift is related to CO42–. These results further suggest that high oxygen solubility promotes the formation of CO4–.
Figure 4. Comparison of Raman spectra of three types of Li-Na MC (Ba-MC, Sr-MC, and MC) exposed to 45% CO2-10% O2-10% H2O-N2 atmosphere at 625°C.
Since both the H2O and alkaline earth metals increase the oxygen solubility of MCs, we also conducted Raman study of the Ba-MC sample under dry gas condition. Figure 5 shows that the Ba-MC sample does exhibit new surface species under CO2-free, H2O-free and wet gas conditions. Similar Raman spectra were obtained under the dry flue gas and wet 45% CO2 atmosphere, confirming that the oxygen solubility highly influences the formation of CO42– and HCO4–. Figure 5 also shows significant appearance of new shifts at 403 and 803 cm–1 under dry 10% O2 condition. DFT calculations suggest that it is related to BaCO3. This finding implies that Ba-MC is unstable under dry 10% O2-N2, precipitating out BaCO3 on the MC surface. However, in the presence of both H2O and CO2, BaCO3 re-dissolves back into MC, but with a pronounced shift at ∼600 cm–1 relating to species HCO4– and CO42–. In addition, the new shift at 360 cm–1 is attributed to CO42– according to the DFT calculation, further indicating that the Ba-added MC enhances oxygen solubility. For convenience, we show in Figure 6 a schematic of the BaCO3 precipitation and re-dissolution mechanisms in dry O2 and CO2-H2O-O2-containing gas mixtures, respectively.
Overall, based on the above Raman spectroscopic results, we propose the following mechanisms for CO2 and H2O transport in MCFCs with a high-basicity MC as the electrolyte, H2 as the anode gas and CO2/H2O/O2 as the cathode gas:
At Cathode:
At Anode:
Since HCO4– is virtually an intermediary species, the experimentally observed H2O and CO2 transport in MCFC are carried out by OH– and CO32–.
In summary, the surface chemistry of pristine and Ba- or Sr-modified Li-Na eutectic under seven gas mixtures has been studied by in situ Raman spectroscopy. Compared to the pristine MC under dry N2, all the CO2-containing dry gas conditions do not change Raman spectra of the MC. Under wet gas conditions, relatively weak new shifts appear in 300–700 cm–1 and their intensities increase with CO2 concentration. Assisted by DFT calculations, the new shifts are identified to be CO42–/HCO4– at low CO2 concentrations and C2O52– at high CO2 concentrations. With the addition of Ba to the pristine MC, the CO42–/HCO4– shifts become more pronounced due to enhanced oxygen solubility in MC. It was also discovered that BaCO3 can precipitate out under CO2-free dry gas environment. Based on the found surface intermediate species, a mechanism concerning H2O and CO2 co-transport is proposed.
The original contributions presented in the study are included in the article/supplementary material, further inquiries can be directed to the corresponding author/s.
PZ conducted the experiment. TW performed the DFT calculations. KH conceived the idea. All authors contributed to the article and approved the submitted version.
Financial supports from National Science Foundation (Award # 1924095) and ExxonMobil are greatly appreciated.
The authors declare that the research was conducted in the absence of any commercial or financial relationships that could be construed as a potential conflict of interest.
The authors would like to acknowledge constructive discussion with Timothy A. Barckholtz, Gabor Kiss, and David Perkins at ExxonMobil.
Bates, J. B., Brooker, M. H., Quist, A. S., and Boyd, G. E. (1972). Raman spectra of molten alkali metal carbonates. J. Phys. Chem. 76, 1565–1571. doi: 10.1021/j100655a013
Cassir, M., Moutiers, G., and Devynck, J. (1993). Stability and characterization of oxygen species in alkali molten carbonate: a thermodynamic and electrochemical approach. J. Electrochem. Soc. 140:3114. doi: 10.1149/1.2220995
Chen, L., Lin, C., Zuo, J., Song, L., and Huang, C. (2004). First spectroscopic observation of peroxocarbonate/peroxodicarbonate in molten carbonate. J. Phys. Chem. B 108, 7553–7556. doi: 10.1021/jp035749l
Chen, L.-J., Cheng, X., Lin, C.-J., and Huang, C.-M. (2002). In-situ Raman spectroscopic studies on the oxide species in molten Li/K2CO3. Electrochim. Acta 47, 1475–1480. doi: 10.1016/s0013-4686(01)00872-6
Claes, P., Thirion, B., and Glibert, J. (1996). Solubility of CO2 in the molten Na2CO3-K2CO3 (42 mol%) eutectic mixture at 800 oC. Electrochim. Acta 41, 141–146. doi: 10.1016/0013-4686(95)00278-m
Frisch, M. J. E. A., Trucks, G. W., Schlegel, H. B., Scuseria, G. E., Robb, M. A., Cheeseman, J. R., et al. (2009). Gaussian 09, Revision d. 01.Wallingford CT: Gaussian. Inc, 201.
Itoh, T., Abe, K., Dokko, K., Mohamedi, M., Uchida, I., and Kasuya, A. (2004). In situ raman spectroelectrochemistry of oxygen species on gold electrodes in high temperature molten carbonate melts. J. Electrochem. Soc. 151:A2042.
Kawase, M. (2017). Durability and robustness of tubular molten carbonate fuel cells. J. Power Sources 371, 106–111. doi: 10.1016/j.jpowsour.2017.10.024
Mendoza, L., Baddour-Hadjean, R., Cassir, M., and Pereira-Ramos, J. P. (2004). Raman evidence of the formation of LT-LiCoO2 thin layers on NiO in molten carbonate at 650 C. Appl. Surf. Sci. 225, 356–361. doi: 10.1016/j.apsusc.2003.10.026
Morita, H., Komoda, M., Mugikura, Y., Izaki, Y., Watanabe, T., Masuda, Y., et al. (2002). Performance analysis of molten carbonate fuel cell using a Li/Na electrolyte. J. Power Sources 112, 509–518. doi: 10.1016/s0378-7753(02)00468-8
O’Boyle, N. M., Tenderholt, A. L., and Langner, K. M. (2008). cclib: a library for package-independent computational chemistry algorithms. J. Comput. Chem. 29, 839–845. doi: 10.1002/jcc.20823
Rosen, J., Geary, T., Hilmi, A., Blanco-Gutierrez, R., Yuh, C.-Y., Pereira, C. S., et al. (2020). Molten carbonate fuel cell performance for CO2 capture from natural gas combined cycle flue gas. J. Electrochem. Soc. 167:064505. doi: 10.1149/1945-7111/ab7a9f
Scaccia, S., and Frangini, S. (2009). Effect of Ba and Ca additions on the oxygen solubility properties of a (70/30) mol% Li2CO3/Na2CO3 carbonate melt. J. Mol. Liq. 146, 39–43. doi: 10.1016/j.molliq.2009.01.011
Tong, J., Lei, X., Fang, J., Han, M., and Huang, K. (2016). Remarkable O2 permeation through a mixed conducting carbon capture membrane functionalized by atomic layer deposition. J. Mater. Chem. A. 4, 1828–1837. doi: 10.1039/c5ta10105k
Watanabe, T., Izaki, Y., Mugikura, Y., Morita, H., Yoshikawa, M., Kawase, M., et al. (2006). Applicability of molten carbonate fuel cells to various fuels. J. Power Sources 160, 868–871.
Zhang, L., Huang, X., Qin, C., Brinkman, K., Gong, Y., Wang, S., et al. (2013). First spectroscopic identification of pyrocarbonate for high CO2 flux membranes containing highly interconnected three dimensional ionic channels. Phys. Chem. Chem. Phys. 15, 13147–13152. doi: 10.1039/c3cp52362d
Zhang, L., Tong, J., Gong, Y., Han, M., Wang, S., and Huang, K. (2014). Fast electrochemical CO2 transport through a dense metal-carbonate membrane: a new mechanistic insight. J. Membr. Sci. 468, 373–379. doi: 10.1016/j.memsci.2014.06.028
Keywords: molten carbonate, surface species, Raman spectroscopy, DFT, alkaline earth
Citation: Zhang P, Wu T and Huang K (2021) Identification of Active Surface Species in Molten Carbonates Using in situ Raman Spectroscopy. Front. Energy Res. 9:653527. doi: 10.3389/fenrg.2021.653527
Received: 14 January 2021; Accepted: 19 March 2021;
Published: 09 April 2021.
Edited by:
Hossein Ghezel-Ayagh, FuelCell Energy, United StatesReviewed by:
Chao-Yi Yuh, FuelCell Energy, United StatesCopyright © 2021 Zhang, Wu and Huang. This is an open-access article distributed under the terms of the Creative Commons Attribution License (CC BY). The use, distribution or reproduction in other forums is permitted, provided the original author(s) and the copyright owner(s) are credited and that the original publication in this journal is cited, in accordance with accepted academic practice. No use, distribution or reproduction is permitted which does not comply with these terms.
*Correspondence: Kevin Huang, aHVhbmc0NkBjZWMuc2MuZWR1
Disclaimer: All claims expressed in this article are solely those of the authors and do not necessarily represent those of their affiliated organizations, or those of the publisher, the editors and the reviewers. Any product that may be evaluated in this article or claim that may be made by its manufacturer is not guaranteed or endorsed by the publisher.
Research integrity at Frontiers
Learn more about the work of our research integrity team to safeguard the quality of each article we publish.