- 1Department of Biotechnology and Bioengineering, CINVESTAV-IPN, Mexico City, Mexico
- 2Environmental Biotechnology and Renewable Energies Group, CINVESTAV-IPN, Mexico City, Mexico
- 3Unidad de Secuenciación e Identificación de Polimorfismos, Instituto Nacional de Medicina Genómica, Mexico City, Mexico
To date, there is an urgent need for implementing practical strategies to reduce CH4 emissions from ruminants. Lovastatin (Lv) is a specific inhibitor of methanogenic archaea. Due to the high cost of pure Lv, solid-state fermentation might be an economical bioprocess to produce Lv and facilitate its use in ruminant nutrition. The goal of this work was to assess the effects of supplementing fermented oat straw as a lovastatin carrier (FOS) to a high-grain ration on in vitro CH4 inhibition and rumen microbiota in beef cattle. The experimental design of in vitro rumen fermentation was completely randomized with four concentrations of Lv in the diet mixture. The supplementation with FOS to give Lv concentration of 100 and 150 mg L−1 in the ruminal fermentation medium significantly inhibited methanogenesis at similar levels. This suggested that less than 20% of FOS was required in the ration to achieve up to 38% of CH4 mitigation without affecting the chemical composition and nutritional value of the ration. Short-chain fatty acid (SCFA) production and profile showed that only the treatments with Lv at 100 and 150 mg L−1 decreased the concentration of total SCFAs; the molar ratio of propionate significantly increased with respect to that of the control. Treatment with Lv at 150 mg L−1 did not result in significant differences in the alpha and beta diversity indices compared to the control. However, significant changes in the relative abundance of some microorganisms were detected, such as an increase in Ruminococcus and a decrease in Prevotella. The predominant 99%+ MA in all controls, treatment, and inocula samples belonged to the Methanobrevibacter genus and very small (negligible) unclassified Methanobacterium genus (Euryarchaeota phylum). Interestingly, the reduction of relative abundance of MA was 39.17%, very close to the percent reduction of CH4 production, 38%. Our data showed that there was a parallel and similar percent decrease of both CH4 production and relative abundance of the predominant MA in our experiment, although the statistical significance was not complete. Finally, our results hold promise for significantly decreasing ruminal CH4 by 38%. Thus, our work is one step toward the sustainable management of the livestock sector.
Introduction
There is an urgent need for finding and implementing practical strategies to reduce greenhouse gases (GHGs) emissions from ruminants, especially its contribution to the atmospheric CH4 level (Havlík et al., 2014). Dangal et al. (2017) found that non-dairy cattle contributed to the major portion of CH4 emissions; they accounted for 70% of the total emissions from the livestock sector (2.72 gigatons of CO2 in the year 2014).
According to US-EPA (2014), the content of CH4 in the atmosphere has increased more than 2-fold compared to the concentration in the preindustrial age. In more recent years, concentrations of CH4 in the atmosphere have risen from 1’100 to 1’800 ppb; this represented ca. 64% in terms of concentration. The average relative rate of increase (relative to the initial concentration in 1950) was 1.01 (% yr−1) (Supplementary Appendix S1).
Mole to mole, CH4 is a 25-fold more powerful GHG than CO2. Thus, CH4 removal is more difficult and expensive if we consider an “end-of-pipe” classical approach of environmental engineering, that is, given the pollutant(s) for removing the pollutant by degradation/transformation/sequestration. Any treatment of the atmosphere to remove CH4 faces at least two stumbling blocks: 1) the massive movement of large flows of air, and 2) the abovementioned low concentration of CH4 in the air.
The alternative means of controlling CH4 contents in the atmosphere by mitigation/prevention measures and processes seem to be more feasible than “end-of-the-pipe” solutions and are described in several roadmaps of GHG abatement (Royal Society-Royal Academy of Engineering, 2018.) Significant savings in energy expenses can be made, as well as saving costs by avoided damages to the environment and health. Hopefully, “freezing” the contents of CH4 in the atmosphere or at least significantly decreasing its rate of accumulation would be achieved.
Our work deals with one of these approaches. The first step is the valorization of agricultural residues by solid-state fermentation (SSF) with fungi to produce lovastatin (Lv) (Ábrego-García et al., 2020; submitted to Scientific Reports). Lv is a well-known inhibitor of methanogenesis in pure cultures of methanogens (Miller and Wolin, 2001; DemonfortNkamga et al., 2017).
To some extent, livestock production can be envisioned as a sort of “animal biorefinery,” a specific type of biorefinery among a large variety of platforms and types (Castro, 2019) and implemented in a direct cascade mode (Poggi-Varaldo et al., 2014; Fava et al., 2015; Escamilla-Alvarado et al., 2017) whose first stage is the valorization of agricultural residues. Afterward, meat, milk, cheese, cream, butter, hides are obtained from animals, minimizing at the same time the emissions of CH4. The collection of ruminal fluids at the slaughterhouse would allow recovering value-added enzymes as well as inocula for biofuel production (Bansal and Goel, 2015; Goel et al., 2015; Weimer, 2015.) Furthermore, more bioenergy can be obtained downstream by hydrogenogenic or methanogenic fermentation of several wastes generated in the process (waste feedstock, animal feces, slaughterhouse wastes, etc.) (Escamilla-Alvarado et al., 2017). The final streams of solids and semisolids that are the remainder of the whole processing can be transformed into a soil amender.
CH4 mitigation strategies have focused mainly on animal breeding, increasing animal productivity, nutrition, and modulation of rumen fermentation (Beauchemin et al., 2020). Regarding the modulation of rumen fermentation, the inhibition of methanogenic archaea (MA) is an effective strategy to mitigate CH4 production from ruminants (Beauchemin et al., 2020). It is opportune to recall that there are three pathways of the archaeal membrane lipid biosynthesis (Jain et al., 2014): the 2-C-methyl-D-erythritol 4-phosphate 1-deoxy-d-xylulose 5-phosphate pathway and two mevalonate pathways. It has been reported that Lv is a specific inhibitor of 3-hydroxy-3-methyl-glutaryl-coenzyme A reductase, which limits the kinetics in the mevalonate pathway (Masters et al., 1995). Moreover, Miller and Wolin (2001) and DemonfortNkamga et al. (2017) have reported that Lv is a strong CH4 suppressor in pure cultures of Methanobrevibacter strains.
On the other hand, a major problem with pure Lv application in animal nutrition is its high cost up to 7.5 US $/g of industrial-grade Lv (Mulder et al., 2018; Sigma-Aldrich, 2020). In this regard, the solid-state fermentation (SSF) of agricultural residues can be an attractive alternative for Lv production because the equivalent price could be as low as 1/20 of that of commercial Lv Supplementary Appendix S2.
The fermented agricultural residues are used as a lovastatin carrier to provide moderate concentrations of lovastatin in cattle rations and to inhibit the ruminal methanogenesis in a significant proportion (Wang et al., 2016; Candyrine et al., 2018).
In general, substrates of SSF are the same as the typical components of animal feed. One of these is oat straw from oat, an agricultural crop widely distributed. In Mexico in the year 2016, 690′204 ha were dedicated to oat cultivation and 10.48 million metric tons of production was reported (SAGARPA, 2017). Approximately 5% of the total (ca. 0.5 million tons) was oat straw (Kim and Dale, 2004).
Oat straw is commonly used as feed for livestock and is characterized by its high content of cellulose, hemicellulose, and lignin (Zheng et al., 2020). Thus, processed agricultural residues such as oat straw and others could be incorporated into ruminant diets and could contribute 1) as a source of Lv and 2) to animal nutrition.
Despite this, very few studies have investigated the effects of fermented crops or agricultural residues (rice and rice straw) as an Lv-carrier supplement in in vitro rumen fermentation (Faseleh Jahromi et al., 2013a; Morgavi et al., 2013). The findings in those reports are subject to certain limitations. First, Lv yields by SSF were very low, 0.26 and 0.57 mg g−1 of dry matter (DM) fermented substrate for Faseleh Jahromi et al. (2013a) and Morgavi et al. (2013), respectively. Second, the in vitro ruminal fermentation substrate was carried out with 100% of fermented rice stubble and rice grain; they could not use a basic diet (total mixed ration) because the resulting final Lv concentration would have been very low and no inhibitory effects would have been detected. This, in turn, introduced a bias in the ruminal fermentation tests. These limitations are evidence of the difficulty of the inclusion of those fermented substrates in the rations without changing their ingredient proportions and chemical composition.
On the other hand, the results associated with pure Lv from in vitro experiments using RFI and forages or total mixed rations and low-dose Lv (<5 mg/L) (Busquet et al., 2005; OBrien et al., 2014) showed no effects of Lv on rumen CH4 production. By contrast, the experiment of Soliva et al. (2011) using the RUSITEC “rumen simulation technique” system, where RFI from a dairy cow, an experimental diet based on ryegrass hay, barley, soybeans, and a single dose of Lv (150 mg/L) were tested, showed that CH4 production was inhibited up to 40%.
So far, no reports exist on supplementing FOS as a lovastatin carrier to cattle ration and its impact on ruminal methanogenesis as well as on the rumen microbiota and fermentation end products under in vitro conditions.
The goal of this work was to assess the influence of supplementing FOS (as a lovastatin carrier) to a high-grain total mixed ration on in vitro methanization inhibition and rumen microbiota in beef cattle.
The contributions of our work revolve around 1) the determination of the effect of supplementing fermented oat straw as a lovastatin carrier (FOS) to a high-grain ration on in vitro; 2) microbial analysis by high-throughput sequencing technology and bioinformatics tools; 3) statistical analysis of key variables of microbial community characterization such as alpha and beta diversity as well as a Pearson correlation of the rumen microbiota and fermentation end products.
Materials and Methods
Experimental Design
We performed two experimental designs based on in vitro ruminal fermentation tests. Regarding the debate between in vitro and in vivo tests, it is worth highlighting that there is a consensus of several experts (Yáñez-Ruiz et al., 2016; Danielsson et al., 2017) in that IVtFT is very useful for screening diets and preliminary evaluation of feed additives and inhibitory agents of CH4 production in ruminal fermentation. They also advise running IVvFT after the IVtFT to confirm the main results as well as to gain more information that can only be obtained in IVvFT, before making decisions or implementing strategies at the commercial level.
In the first experiment, we examined the effects of Lv concentration (through FOS supplementation) on the CH4 production in vitro rumen fermentation using a completely randomized design. The treatments were four concentrations of Lv in the medium: 0, 50, 100, and 150 mg L−1. The dose of 0 mg L−1 acted as a control. The doses were chosen to represent the interval of Lv concentrations reported for in vitro rumen fermentation experiments close to ours Supplementary Appendix S3. The response variables were total gas production (TGP), hydrogen, CH4, and short-chain fatty acids (SCFAs), among others.
In the second part of the experiment, the effect of Lv (supplemented by FOS) on microbial communities of batch ruminal fermentation was determined. To this goal, samples from the first experiment, that is, the control, treatment at 150 mg Lv L−1, and inoculum (ruminal fluid), were chosen for analysis and determination of alpha and beta diversity and the relative abundance of several phyla and families of key eubacteria and methanogenic archaea (MA), using high-throughput sequencing technology.
In both experimental designs, each treatment had three independent repetitions according to the replication practice in similar literature dealing with IVtFT (Morgavi et al., 2013; Danielsson et al., 2017b; O Brien 2014; Khonkhaeng and Cherdthong, 2020). Finally, the Spearman correlation coefficient between rumen microbiota and fermentation end products was calculated.
In vitro Rumen Fermentation Technique
The FOS supplement for rumen CH4 mitigation was obtained from our previous work (Ábrego-García et al., 2020, submitted to Scientific Reports.). The overall Lv concentration was 24 mg g−1 DM of oat straw. Five grams of oat straw were ground (5 mm), placed in 250 ml Erlenmeyer’s flask, and moisture content adjusted to 70% with the SSF medium (Jirasatid et al., 2013). The pH of the culture medium was set to 5.5. Then, the flasks were sterilized at 121°C/15 min and seeded with 2 ml of spore suspension A. terreus (CDBB H-194). Afterward, the temperature was reduced to 28°C/24 h and then to 26°C until the end of the incubation period (Xu et al., 2005).
FOS was dried in a convective, forced-air oven (55°C/248 h) and powdered. Dry-powdered substrates (1 g) were extracted with 40 ml of ethyl acetate in 250 ml Erlenmeyer’s flasks, and they were agitated for 2 h in a shaker at 200 rpm. The mixture was separated by filtration through a membrane filter (0.22 Durapore, Millipore, MA, United States). The solvent was evaporated in a rotary evaporator under vacuum at 60°C. The dry residual was re-dissolved in 5 ml of acetonitrile and further filtered through 0.22-μm Acrodisc syringe filters (Millipore, MA, United States of America) before HPLC analysis (Jaivel and Marimuthu, 2010). Lv was determined as reported by Yang and Hwang (2006) using HPLC (Varian Analytical Instruments, Model 9010, CA, United States). More detailed information on this procedure can be found in Supplementary Appendix S4.
The in vitro rumen fermentation was performed in 120-ml serum bottles. The main substrate of ruminal fermentation was a high-grain ration constituted by ground corn grain, soybean meal, urea, mineral mix, accompanied by FOS as the Lv carrier, and oat straw (to compensate FOS) (Table 1). Afterward, ruminal-fluid inoculum (RFI) and liquid medium were added to give the target Lv concentrations per liter of the mixture. Four treatments with four target concentrations of Lv in the suspension mixture (0, 50, 100, and 150 mg Lv L−1 of suspension mixture volume) were implemented, which corresponded to 0, 95, 189, and 284 g DM-FOS kg−1 DM of solids mixture (Table 1).
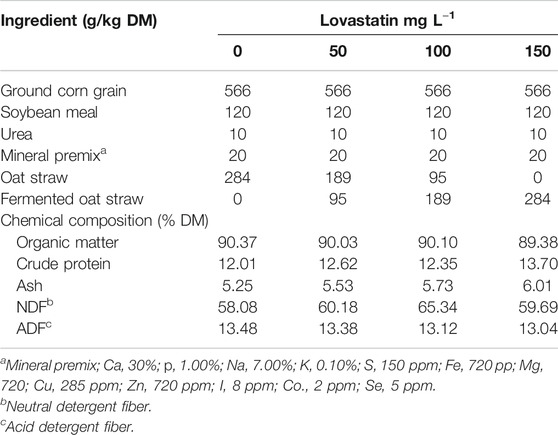
TABLE 1. Ingredients, chemical composition, and proportions of fermented oat straw as a lovastatin-carrier additive in the experimental diets.
The FOS was not sterilized before its use in this test. For more details on the units and how the solids mixtures were implemented for each target dose of Lv, please see Supplementary Appendix S5.
All ingredients of the cattle diets were ground and sieved through a 1-mm mesh screen, and 1 g of the ration was transferred into the serum bottles. The chemical composition of the ration with different proportions of FOS was very similar and contained an average (DM%) of 89.97 organic matter, 12.67 crude protein, and 5.63 ash; the predominant component of the diets was ground corn grain as an energy source and the second component in importance was soybean meal as a source of protein (Table 1). This ration is typical of beef cattle production in the finishing stage (Gaey, 1984).
Then, 30 ml of the buffer mineral medium (Menke and Steingass 1988) was transferred into the bottles and fitted with rubber stoppers and capped with aluminum caps. Finally, the bottles were purged with CO2 gas to ensure anaerobic conditions and conserved at –4°C.
The RFI was collected from three cannulated steers. The steer management procedures in our work were approved by the Animal Care Committee, Livestock Farming Program, Campus Montecillos, Colegio de Postgraduados (Texcoco, Edo. de Mexico). Technical specifications appropriate for the care and use of animals were according to the Official Mexican Standard NOM-062-ZOO-1999 (DOF Diario Oficial de la Federación, 2001).
The diet fed to steers contained forage and concentrate in the ratio of 60:40. The whole RFI was sampled manually 2 h before morning feeding, transferred to preheated 1.5-L thermos, and brought to the laboratory within 1 h post-collection. The RFI was filtered through four sheets of cheesecloth and mixed in equal portions into a flask in a water bath at 39°C under CO2. Immediately, 15 ml of FRI was transferred into prewarmed serum bottles, and they were placed in an orbital shaker incubator at 70 oscillations min−1 at 39°C for 48 h. We used three extra serum bottles (buffer mineral medium + RFI) as blanks for the correction of TGP.
Bromatological Analysis of the Diets
Experimental diets were analyzed as described by the AOAC (2002): organic matter, dry matter, ash, and crude protein contents. Also, neutral detergent fiber (NDF) and acid detergent fiber (ADF) were determined according to van Soest et al. (1991); NDF was performed with the addition of stable amylase and sodium sulfite.
Measurements of Total Gas Production, Hydrogen, CH4, and Short-Chain Fatty Acids
Total gas production was measured by acid brine displacement at 12, 24, 36, and 48 (Escamilla-Alvarado et al., 2014). Before the measurement of TGP, a sample was taken from each culture’s headspace and analyzed for CH4 and H2 determination by a gas chromatograph (Gow-Mac, Model 350, Pittsburgh, PA, United States of America) equipped with a TCD and a column packed with Molecular Sieve 5 A.
The temperatures of the detector, injector, and column at 100, 25, and 25°C, respectively; chromatographic Ar was the carrier. CH4, H2, and Ar standards were of chromatographic quality (>99.9%, INFRA S.A. de C.V., Mexico City, Mexico).
Pressure–volume–temperature characteristics of biogas samples were used to calculate biogas volume, according to the ideal (perfect) gas law (Castellan, 1983). Gas volume results were standardized (SmL) at standard pressure and temperature, 101.32 kPa and 298 K, respectively (Escamilla-Alvarado et al., 2014).
After 48 h of incubation, the fermentation was stopped by keeping serum bottles at –10°C for 10 min, and the pH was measured. For the determination of SCFA, the samples were analyzed as reported by Cottyn and Boucque (1968). Briefly, 250 μL of metaphosphoric acid (25%) was added to 750 μL of supernatant rumen fluid from the fermentation bottles. The samples were then centrifuged (15,000 g 10 min.−1; 4°C), and 700 μL of the clear supernatant was transferred to gas chromatography vials and injected into a PerkinElmer (Autosystem) gas chromatograph that was equipped with an FID and a capillary column (Model ZB-FFAP, Phenomenex, United States). The detector and injector temperatures was 250°C. N2 was the carrier at a flow rate of 20 ml min−1. Regarding the oven, its temperature program was as follows: 60°C for 2 min, an increase of temperature up to 140°C with a ramp of 5°C min−1, and then, a constant temperature of 140°C for 6 min (Muñoz-Páez et al., 2014). The external standard used was purchased from Sigma-Aldrich, Volatile Free Acid Mix CRM46975, Bellefonte, PA, United States.
Isolation of Total DNA From Rumen Fluid Samples
Total genomic DNA was isolated from the bottom (6 ml) and the fluid surface (6 ml) of the serum bottles. Afterward, they were centrifuged at 10,000 g/30 s. The pellets were recovered for the DNA extraction with a commercial kit (UltraClean Soil DNA Isolation Kit de MO BIO). DNA concentrations were determined using an A260/280 spectrophotometer (Epoch Microplate, BioTek Instruments, Vermont, United States), and the quality was assessed by electrophoresis in agarose gel. Samples of DNA were kept at –80°C until analyses.
16S rDNA Library Preparation and Sequencing
The 16S rRNA gene sequences were amplified with the barcoded primers 341F and 806R (V4 region of bacteria and archaea) according to Zhu et al. (2018). Library preparation was carried out following 16S metagenomic sequencing library preparation using SuperFi 2x MM enzyme, and the samples were amplified with the following program: 98°C/30 s (1 cycle), 98°C/10 s, 50°C/30 s, 72°C/30 s (25 cycles), and 72°C/5 min. Amplicon sequencing was performed by Illumina MiSeq (2 × 250 pb) technology at the INMEGEN (Mexico City, Mexico). The read sequences were deposited in the Sequence Read Archive (SRA) database of the NCBI (BioProject: PRJNA690783).
Bioinformatic Analysis
The paired-end sequences were joined by q2-vsearch algorithm QIIME2, v. 2019.1 (Rognes et al., 2016). The adapters were removed with the Cutadapt tool (Martin, 2011). Quality control of the sequences was performed by the Deblur algorithm (Weiss et al., 2017).
Alfa and beta indices diversity as well as taxonomy-based analyses were calculated by QIIME two software, v. 2019.1 (Rideout et al., 2018). Sequences were organized into OTUs (operational taxonomic units) with 99% identity and classified into the taxonomical levels based on the Greengenes (v. 13.8) database (http://greengenes.lbl.gov).
Statistical Analysis
TGP, hydrogen, CH4, SCFA, relative abundance, and alpha diversity indices were subjected to the general linear effects model with PROC GLM of SAS Studio, v 3.8 (SAS Inst. Inc., Cary, NC, United States), and the LSD (Least Significant Difference) post hoc test was used to assess any significant means differences.
All graphics were performed using R studio (3.6.1). Alpha diversity boxplot was created with ggplot two package according to Hill et al. (2003). Beta diversity was generated by principal component analysis (PCA) of unweighted UniFrac and plotted with the Vegan and the ggplot2 packages (Lê et al., 2008). The Spearman correlation heat map between fermentation end products and rumen microbiota was calculated with the Hmisc package. Statistical significance was considered when the p-value < 0.05.
Results and Discussion
Effect of Lovastatin Concentration on Total Gas Production, CH4, Hydrogen, and Short-Chain Fatty Acids
The results obtained from in vitro rumen fermentation (TGP, CH4, hydrogen, and SCFA) are presented in Table 2. CH4 production at 48 h of incubation was significantly lower for all concentrations of Lv (p < 0.05); therefore, a similar pattern of TGP was observed.
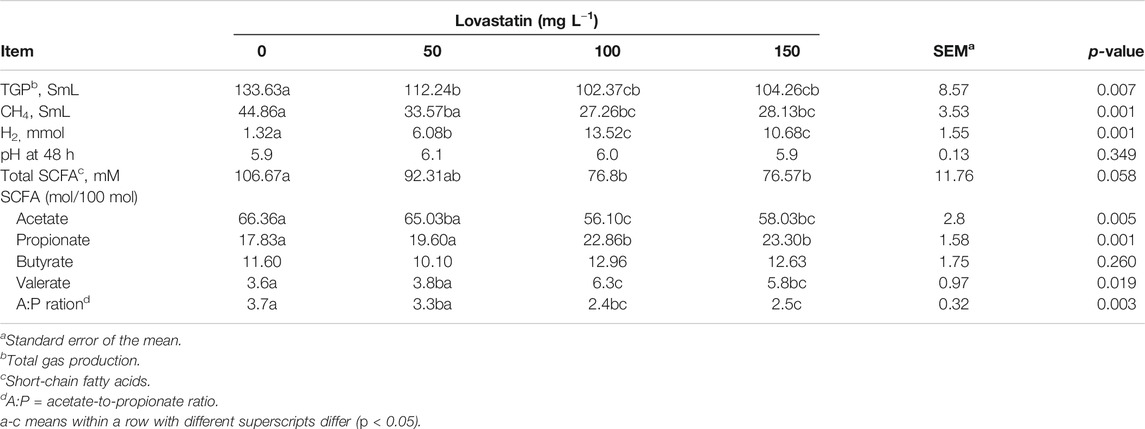
TABLE 2. Effects of fermented oat straw as a lovastatin-carrier additive on total gas production, methane, and short-chain fatty acids.
The CH4 mitigation was similar in the FOS treatments with concentrations of Lv at 100 and 150 mg L−1; both treatments showed inhibition levels of 38% compared to the control. As a reminder, all our treatments with Lv used FOS as Lv carrier.
This could be attributed to the inhibition of specific methanogens present in the rumen which are known to synthesize their cell membrane via mevalonate, for instance, M. ruminantium (Bharathi and Chellapandi, 2017). Moreover, it has been reported that Lv completely inhibited CH4 production of the strain (DemonfortNkamga et al., 2017).
Our finding agreed with the reports of Soliva et al. (2011) who observed that Lv dosage at 150 mg L−1 mitigated CH4 production by up to 42% in in vitro ruminal fermentation studies. According to Busquet et al. (2005) and O’Brien et al. (2014), low concentrations of commercial Lv (at five and 3.2 mg L−1) did not significantly inhibit ruminal methanogenesis. However, Faseleh Jahromi et al., 2013b demonstrated that processed rice straw as a lovastatin carrier (concentration of Lv on the rumen fluid-based medium 4.32 mg L−1) induced CH4 and TGP mitigation by 21 and 16% (p < 0.01), respectively, using the processed rice straw as the only feed ingredient of in vitro rumen fermentation. The use of high doses of Lv (100–150 mg L−1) in our work and in that of Soliva et al. (2011) could raise concerns on possible toxic effects of this substance on cattle. In principle, we did not evaluate the toxicity of Lv in cattle. Moreover, to the best of our knowledge, this type of study is nonexistent or very scarce in the open literature (Web of Science, 2021). Only one record was found in the period 2000–2021, and it dealt with arsenic toxicity in animals. However, more R&D on the toxicity of Lv and other inhibitors of cattle methanogenesis is clearly a must for the safe and sound application of the Lv approach for CH4 mitigation.
A close work by Ramírez-Restrepo et al. (2014) evaluated the effect of supplementing several doses of fermented red rice as Lv carrier on live animal weight and dry matter intake of cattle, as well as ruminal CH4 emission. The microbial agent was Monascus purpureus, an Lv-producing fungus. Some toxic episodes were observed in cattle at the highest dosages of fermented red rice in the feed, although the authors ascribed this effect to the fungus and some of its metabolites, not the Lv.
Recently, Leo et al. (2020) examined the effects of Lv on skeletal muscles of goats, intending to identify whether Lv supplementation of goat diets could negatively affect the health and welfare of these small ruminants. Four groups of five male goats each were fed a ration that contained 22.8% grain concentrate, 50% rice straw, and 27.2% of PKC (palm kernel cake; either fermented with A. terreus and non-fermented), to give 0, 2, 4, and 6 mg Lv/kg LW. Fermented PKC was the Lv-carrier component of the diet. They found degeneration in selected muscles of the goats that were fed the highest dose of Lv. The authors concluded that supplementing naturally produced Lv at a dose >4 mg/ kg Lv could negatively affect the welfare and health of the treated animals.
The limitation of the work by Leo et al. (2020) is that the adverse effects on the goat muscles cannot be ascribed to Lv per se since the authors did not include positive controls where the diets were enriched with pure (commercial grade) Lv. Due to the lack of positive controls, the negative effects determined in this work could have been caused either by Lv, or other compounds or microbes contained in the fermented residue carrying the Lv. In summary and unfortunately, there is not a conclusive case against Lv use in goat diets.
Instead of using Lv or other specific chemical inhibitors, a promising avenue for ruminal CH4 mitigation is the use of cultures (for instance, yeast cultures) as an inhibitor of CH4 production in ruminal fermentation. In the work of Ruiz et al. (2016), the authors studied the effects of the supplementation of Candida norvegensis (Cn) (live cells and likely metabolites present in the used medium) on the ruminal fermentation of oat straw (as the sole source of carbon, no actual cattle ration was used). Their results hold promise for developing an original approach to mitigation of ruminal CH4 generation. Neither Lv nor other conventional inhibitor was used for inhibition of the ruminal methanization. Batch tests of 36-h duration were run, with four replicates. Treatments were “Cn addition” and “no Cn addition” (or control), and 5 times of incubation were sampled. It was found that a significant CH4 production inhibition occurred only at 8-h incubation. Incubations at 4, 12, 24, and 36 h failed to demonstrate a significant CH4 generation inhibition. The latter was likely related to a high value of the standard error of the mean of the experiment.
On the other hand, the hydrogen gas production significantly increased with concentrations of Lv 100 and 150 mg L−1 (3 and 8 fold increase relative to control, respectively, Table 2). It is known that methanogenesis continually removes H2; the latter is used as an energy source. A possible explanation for this is that once the main sink for H2 in the rumen is inhibited (methanogens), the H2 generated in the fermentation starts to accumulate in the gas headspace. This has been reported for inhibited methanogenic consortia (Sparling et al., 1997; Martinez-Fernandez et al., 2016). Similarly, Guyader et al. (2016) reported that H2 accumulation occurred in around 15 and 25 h of incubation (7- fold with respect to control) for in vitro bovine rumen fermentation of a diet characterized by an f:c ratio of 50:50 spiked with nitrate as the methanogenesis inhibitor.
In our work, the contents of total SCFA at the end of the fermentation in units with Lv 100 and 150 mg L−1 was significantly reduced by 28% compared to that in the control (p < 0.05, Table 3). Interestingly, there was no significant difference between SFCA concentration in the units with 50 mg L−1 and that in the control units.
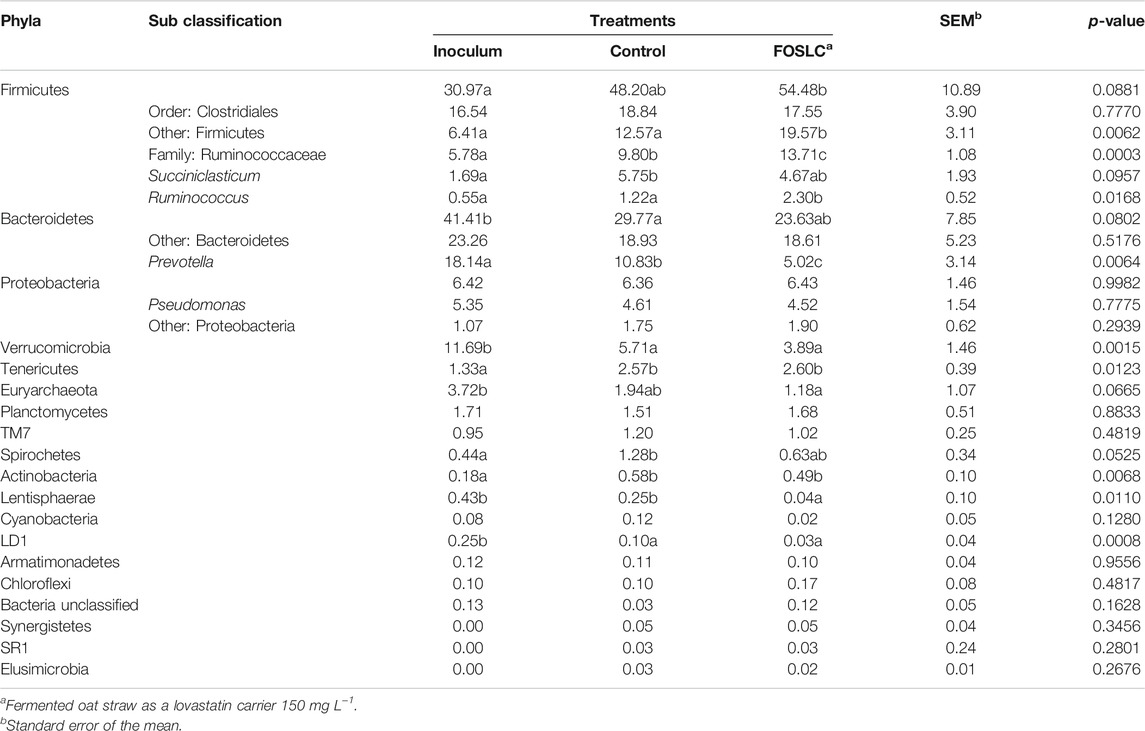
TABLE 3. Relative abundance of bacteria and archaea of rumen fermentation in the following treatments: inoculum, control, and fermented oat straw as lovastatin carrier 150 mg L−1.
The molar proportion of acetate was reduced by 14% when comparing the treatments with Lv 100 and 150 mg L−1 (p < 0.05) to the control. Yet, the molar percentages of valerate and propionate increased (p < 0.01). The Lv concentration did not affect the molar percentage of butyrate (p > 0.26). The ratio of acetic to propionic acids decreased only in the treatments with concentrations of Lv 100 and 150 mg L−1 (p < 0.05) (Table 2).
The methanogenesis abatement would imply the existence of alternative routes for the electron flow, some of them thriving on the accumulated H2 (Weimer, 1998). For instance, propionate production can be one of these pathways since electron flow would shift to propionate. Likely, this could have occurred in the treatments with 100 and 150 Lv mg L−1, where the final propionate molar proportion increased up to 28%.
Our results agreed fairly well with those of Ungerfeld (2015) and Capelari and Powers (2017), who reported a significant increase in the molar proportion of propionate in vitro batch cultures of rumen fluid with either forage or grain diets as a substrate when CH4 production was inhibited.
Effect of Lovastatin Concentration on Alpha and Beta Diversity
Rarefaction curves (Supplementary Appendix S6) suggested that our samples were close to asymptotic patterns. This, in turn, indicated that the depth of sequencing covered most of the existing microorganisms (Brewer and Williamson, 1994).
As shown in Figure 1, the alpha diversity; Shannon and Simpson indices; the richness index, Chao1; the evenness index, Pielou, and Faith; and as well as the number of observed OTUs showed that there were no significant differences between the Lv-treated, control with no Lv, and inoculum.
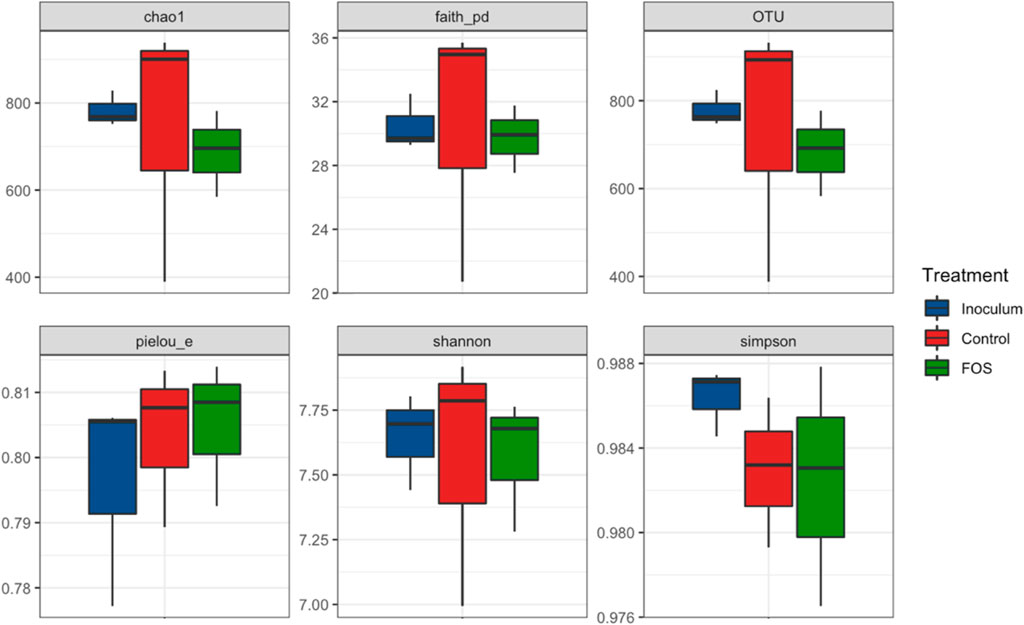
FIGURE 1. Alpha diversity measures of rumen microbiota in the following treatments: inoculum, control, and FOS (Lv concentration 150 mg L−1).
The mean OTU richness of the treatments was 783, 687, and 743 for the rumen inoculum, the control, and the treatment with Lv 150 mg L−1, respectively. Diversity indices of Chao1 ranged from 687 to 779. Its trend, and the pattern of the diversity indices Faith and Shannon, paralleled the trend shown for the OTU values (Figure 1).
The diversity index Shannon–Weaver based on log 10 was on the order of 7.6; the highest value corresponded to the control, which denotes high diversity among the microorganisms that make up this community. We obtained values for the Simpson index in a narrow and high range (0.983–0.987), also designating high diversity for the treatments. Pielou’s index fell in a very narrow interval of 0.805–0.808. Since a value of 1 would mean a perfect evenness, those values indicated a fair degree of equidistribution of microbial groups in our samples (Magurran, 2004).
Our results were consistent with those of Zhou et al. (2020) who evaluated the effect of oregano essential oil concentration (130 mg L−1) on in vitro fermentation and rumen microbiota. In their work, when CH4 production was inhibited (p < 0.05), no significant differences in several diversity indices were found between the control and essential oil–treated units (p > 0.05). For instance, Shannon index values were 8.01 and 8.12, Simpson of 0.99 and 0.99, Chao1 of 1979.1 and 1928.0, and OTU numbers of 1297 and 1323, for control and treatment, respectively.
The analysis of beta diversity using PCA (weighted UniFrac distances) revealed that the mean distance between inoculum, control diet, and the treatment with Lv 150 mg/L was not separated into clusters, indicating that Lv treatment had no impact on the beta diversity (Figure 2A). Also, the Venn diagram (Figure 2B) suggested that the treatment groups shared most of the microorganisms which confirmed that the concentration of Lv 150 mg L−1 did not affect the beta diversity of the rumen microbiota. These results agree with the findings of other studies, where SSF of palm kernel cake (as a source of Lv) was used as a supplement to mitigate rumen CH4 emissions in goats (Candyrine et al., 2018). It was found that the fermented palm kernel cake did not affect the alpha and beta diversity of the rumen microbiota.
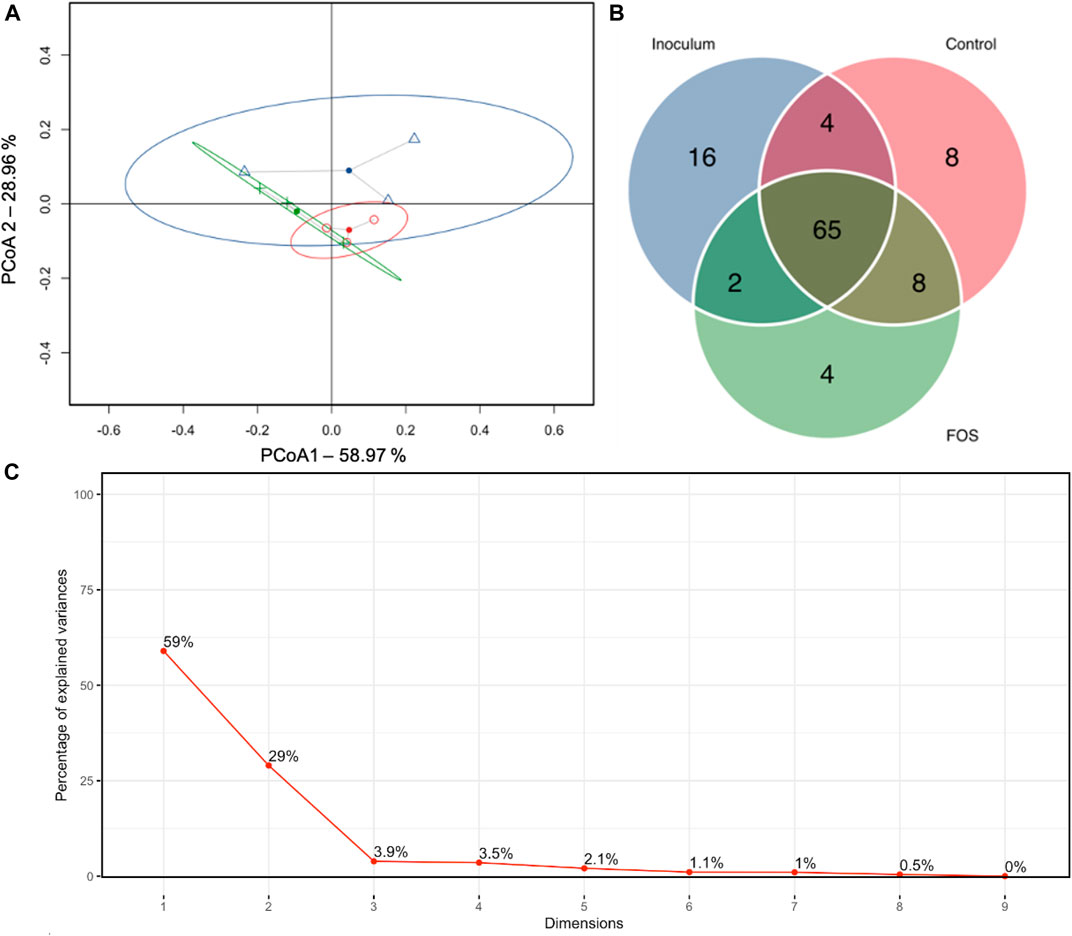
FIGURE 2. (A) Principal coordinate analysis derived from weighted UniFrac distance among samples of treatments (inoculum, blue; control, red; and concentration of Lv 150 mg/L, green). (B) Venn diagram analysis among three treatments. (C) The percentage of variation explained by each PCoA axis.
Effect of Lovastatin on the Relative Abundance of the Rumen Microbiota
The relative abundances at the phylum level of rumen microbiota among the treatment groups are shown in Figure 3. Nineteen bacterial phyla were assigned with the predominance of Bacteroidetes and Firmicutes, accounting for 70% of the total sequences. These results are consistent with those of Wu et al. (2012) and Myer et al. (2016) who found that these two phyla were predominant in bovine rumen communities.
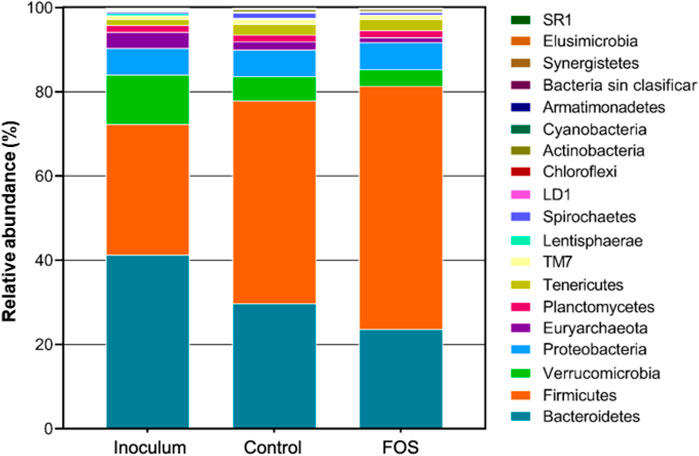
FIGURE 3. Microbiota profiles at the phylum level representing bacterial and archaea of the following treatments: inoculum, control, and supplemented with fermented oat straw (Lv concentration of 150 mg L−1).
Subsequently, the samples were dominated by phyla: Verrucomicrobia (∼7%), Proteobacteria (∼6%), Euryarchaeota (∼2%), Tenericutes (∼2.1%), Planctomycetes (∼1.6%), and the remainder was represented by <3% of the total sequences (Figure 3).
At the phylum level, a significant change was observed in the relative abundance: Lentisphaerae was reduced at a concentration of Lv 150 mg L−1, suggesting a possible subacute ruminal acidosis (Mao et al., 2013). However, concentration of Lv had no effect on the medium pH (p > 0.05; Table 3).
The predominant 99%+ MA in all controls, treatment, and inoculum belonged to the Methanobrevibacter genus and very small (negligible) unclassified Methanobacterium genus. Both genera are included in the Euryarchaeota phylum. No genera from the phylum Crenarchaeota were found in our samples (Brochier-Armanet et al., 2008).
Moreover, the percent reduction of relative abundances of MA between control and “treatment 150 mgLv/L” ηc-t was given by the following equation:
with p < 0.066, close to p = 0.05; unfortunately the SEM was high, 1.07% (new Table 3).
Interestingly, the reduction of the relative abundance of MA was very close to the percent reduction of CH4 production, 38% (Table 2). This is a striking parallel trend between methanogenesis inhibition and the relative abundance of MA, irrespective of the sharp p-values.
In summary, our data showed that there was a parallel and similar percent decrease of both CH4 production and relative abundance of the predominant MA in our experiment. The statistical significance was not complete since the decrease in CH4 production was significant (p < 0.05), whereas the decrease in the relative abundance of MA was p = 0.066.
The observed trend was consistent with Gottlieb (2916) and Miller and Wolin (2001) who reported negative effects of Lv on the growth and activity of MA pure cultures.
As we mentioned in the Introduction section, Lv as an HMGR inhibitor disrupts the cell membrane by interfering with the biosynthesis of isoprenoids. This, in turn, disturbs the membrane-bound respiratory chain of methanogenesis and mevalonate pathway (Gottlieb et al., 2016). Previous experimental works have shown that the growth and activity of several MA were significantly reduced by Lv; the Lv’s effect on cellulolytic bacteria was negligible (Wolin and Miller, 2001).
Interestingly, Sharma et al. (2011) modeled the behavior of the enzyme F420–dependent NADP oxidoreductase. It is known to catalyze the electron transfer during methanogenesis in Methanobrevibacter smithii. Consequently, it can be a potential target for interrupting CH4 production. They conducted a modeling study. In the last stage of their research (the inhibitor prediction study), it was predicted that mevastatin and Lv produced more affinity for the model structure of NADP oxidoreductase as compared to F420. Therefore those compounds might act as a potential inhibitor of F420-dependent NADP oxidoreductase protein.
One consequence of Sharma’s hypothesis could be that this mechanism of decrease of CH4 production would not be necessarily related to a decrease in the MA growth. However, this interpretation should be considered with caution because it is a result of a model prediction, not an experimental fact.
In this context, Wang et al. (2016) evaluated the effect of red yeast rice inclusion in the ration (as a source of Lv, 4 mg kg−1 LW) of goats and reported that the abundance of Methanobrevibacter significantly decreased.
Regarding other microbial groups, at the genus level, the following changes were observed: Prevotella was significantly reduced (p < 0.05) by a concentration of Lv 150 mg L−1 compared with the control. Prevotella is directly associated with cattle fed high-grain diets (Wu et al., 2012; Tao et al., 2017). The observed decrease in Prevotella could be interpreted as the result of hemicellulose degradation during the early stages of in vitro fermentation (Emerson and Weimer, 2017).
On the other hand, it has been reported that Ruminococcus is one of the predominant cellulolytic genera in the rumen (Puniya et al., 2015). The family Ruminococcaceae, as well as the Ruminococcus genus abundance, increased by 2.38- and 4.18- fold (p < 0.05) in units with Lv 150 mg L−1 compared with the control.
The advantages of the FOS Lv carrier derives from its production with the fungus A. terreus, which is known to produce fibrinolytic enzymes, and it also exhibits minimal potential hazard to humans, the environment, and minimal biosafety protection (Level 1) (Prévot et al., 2013 and CDCP (2020). Despite the latter, studies on the potential toxicity of FOS and other fermented residues that act as an Lv carrier (toxicity either due to surviving A. terreus or fermentation metabolites that are not usually monitored) is an important area of research that should be reinforced to confirm the R&D of the SSF approach to Lv production and feeding.
Figure 4 displays Spearman’s correlation between the rumen microbiota and fermentation end products. Only significant correlations are discussed here.
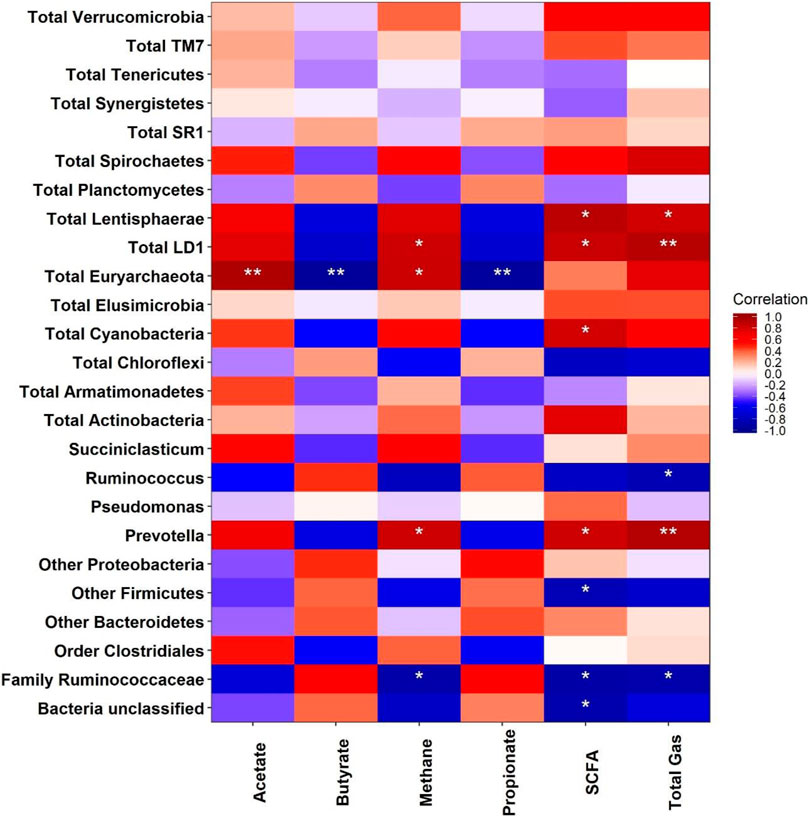
FIGURE 4. Correlation between rumen fermentation parameters and the microbiota identified from phylum to genus.
Euryarchaeota was negatively correlated (p < 0.01) with the production of propionate and butyrate, whereas it was positively correlated with the production of CH4 and acetate. The correlation between Euryarchaeota abundance and CH4 was expected since decreases of abundance and CH4 of the control vs treatment 150 mgLv/L were 38 and 39%, respectively. A strong correlation between the Euryarchaeota abundance and CH4 was also reported by Danielsson et al. (2017) in experiments with a variety of cows fed the same diet. Most ruminal methanogenic archaea that metabolize CO2 and H2 (CO2-reducing methanogenesis) correspond to the Euryarchaeota phylum (Borrel et al., 2016). For example, Henderson et al. (2015) in their extensive work reported that M. gottschalkii and M. ruminantium were the most predominant methanogenic archaea in rumen fluid samples of ruminants (cattle, sheep, goats, and cervids) and camelids from different geographical regions. Also, the data reported about negative Spearman's correlations between Euryarchaeota butyrate and Euryarchaeota propionate as discussed above appear to substantiate that propionate was considered as an alternate sink for H2 in the rumen.
Prevotella genus was positively correlated with the production of TGP (p < 0.01), SCFA, and CH4 (p < 0.05). As already stated, Prevotella contributes to ruminal hemicellulose degradation and some fermentation end products are acetate, CO2, and H2 (Emerson and Weimer, 2017). Our results were congruent with the findings of Shen et al. (2017), who observed a significant positive correlation between Prevotella and the production of CH4, acetate, and butyrate when in vitro CH4 production was inhibited by monensin and nisin treatments (p < 0.05).
Despite the low relative abundance of Lentisphaerae, a positive correlation (p < 0.05) with TGP and SCFA was found. This result was likely due to the contribution of glycoside hydrolase enzymes of Lentisphaerae to the hydrolysis of the dietary cell wall polysaccharides (Kaoutari et al., 2013).
Contrary to expectations, the family Ruminococcaceae was negatively correlated (p < 0.05) with TGP, CH4, and SCFAs. It is known that family Ruminococcaceae includes predominant cellulolytic bacterial species (R.?avefaciens and R. albus) and they can produce some SCFAs and substrates that are consumed by methanogenesis (Zhou et al., 2015); thus, some parallel development of Ruminococcaceae and variables associated with robust methanogenesis would have been expected. Despite this, our findings appear to be supported by Mi et al. (2018) who showed that family Ruminococcaceae was negatively correlated with the production of CH4 from in vivo experiments.
Conclusion
This study provided evidence that the supplementation of FOS (to give concentrations of Lv 100 and 150 mg L−1) in the high-grain diet has potential for rumen CH4 mitigation without significantly changing the chemical composition of the diet. Less than 20% of FOS supplement was required in the diet to achieve up to 38% of CH4 mitigation.
SCFA production and profile showed that only the treatments with Lv at 100 and 150 mg L−1 decreased the concentration of total SCFA; the molar ratio of propionate significantly increased with respect to that of the control.
The ruminal fermentation at Lv 150 mg L−1 did not show significant differences in the alpha and beta diversity indices compared to the control. However, significant changes in the relative abundance of some microorganisms were found, such as an increase in Ruminococcus, and a decrease in Prevotella.
The predominant 99%+ MA in all controls, treatment, and inocula samples belonged to the Methanobrevibacter genus and very small (negligible) unclassified Methanobacterium genus (Euryarchaeota phylum). Interestingly, the reduction of relative abundance of MA was 39.17%, very close to the percent reduction of CH4 production, 38%. Our data showed that there was a parallel and similar percent decrease of both CH4 production and relative abundance of the predominant MA in our experiment, although the statistical significance was not complete.
Data Availability Statement
The original contributions presented in the study are publicly available. These data can be found at the NCBI repository, Sequence Read Archive (SRA) database, with accession number PRJNA690783
Author Contributions
All authors listed have made a substantial and intellectual contribution to the work.
Funding
This research was funded by Ciencia Básica, SEP-CONACYT project (grant number A1-S-26901). Partial funding from a personal grant of Dr Héctor M. Poggi-Varaldo to the Environmental Biotechnology and Renewable Energies through CINVESTAV is appreciated.
Conflict of Interest
The authors declare that the research was conducted in the absence of any commercial or financial relationships that could be construed as a potential conflict of interest.
Acknowledgments
The authors wish to thank the guest editor of the Topic Issue of Frontiers in Energy Research and the anonymous reviewers, for the insightful suggestions and advice that helped improve the manuscript. CONACYT granted a graduate fellowship to one of the authors (Ph. D. candidate Amaury Ábrego-García). We also thank Professor Omar Hernández-Mendo (COLPOS, Programa de Ganadería) for giving us access to the rumen-cannulated steers and IQI. Gustavo G. Medina Mendoza for his skilful technical chromatographic assistance. Partial support by a personal grant of HéMP-V to the Environmental Biotechnology and Renewable Energies through CINVESTAV is acknowledged.
Supplementary Material
The Supplementary Material for this article can be found online at: https://www.frontiersin.org/articles/10.3389/fenrg.2021.630701/full#supplementary-material.
Notation
ADF Acid detergent fiber
Cn Candida norvegensis
CP Crude protein
DM Dry matter
DMI Dry matter intake
FOS Fermented oat straw, lovastatin carrier
GHG Greenhouse gases
IVtFT In vitro fermentation test
IVvFT In vivo fermentation test
LSD Least significant difference
Lv Lovastatin
MA Methanogenic archaea
NDF Neutral detergent fiber
OM Organic matter
OTUs Operational taxonomic units
PCA Principal Component Analysis
PKC Palm kernel cake
RFI Ruminal-fluid inoculum
SCFA Short-chain fatty accidents
SEM Standard error of the mean
SFF Solid-state fermentation
SM Supplementary Material
TGP Total gas production
Greek characters
ηc-t percent decrease of relative abundance of MA between the control (0 mgLv/L) and the “treatment” with 150 mgLv/L
ηin-t decrease of relative abundance of MA between the inoculum and the “treatment” with 150 mgLv/L.
References
ÁbregoGarcía, A., Poggi-Varaldo, H. M., Mendoza-Vargas, A., Robles-González, V. S., Ríos-Leal, E., Ponce-Noyola, M. T., et al. (2020). Valorization and characterization of two crop residues by solid-state fermentation for lovastatin production. Scientific Reports.
Aoac, (2002). Association of official analytical chemists official methods of analysis. 17th ed. Gaithersburg, MA: AOAC International.
Bansal, S., and Goel, G. (2015). “Chapter 19. Commercial application of rumen microbial enzymes,” in Rumen microbiology: from evolution to revolution. Editors A. K. Puniya, R. Singh, and D. N. Kamrapp (New Delhi, India: Springer), 281–292.
Beauchemin, K. A., Ungerfeld, E. M., Eckard, R. J., and Wang, M. (2020). Review: fifty years of research on rumen methanogenesis: lessons learned and future challenges for mitigation. Animal 14 (S1), S2–S16. doi:10.1017/S1751731119003100
Bharathi, M., and Chellapandi, P. (2017). Intergenomic evolution and metabolic cross-talk between rumen and thermophilic autotrophic methanogenic archaea. Mol. Phylogenet. Evol. 107, 293–304. doi:10.1016/j.ympev.2016.11.008
Borrel, G., Adam, P. S., and Gribaldo, S. (2016). Methanogenesis and the wood-ljungdahl pathway: an ancient, versatile, and fragile association. Genome Biol. Evol. 8 (6), 1706–1711. doi:10.1093/gbe/evw114
Brewer, A., and Williamson, M. (1994). A new relationship for rarefaction. Biodivers Conserv 3 (4), 373–379. doi:10.1007/bf00056509
Brochier-Armanet, C., Boussau, B., Gribaldo, S., and Forterre, P. (2008). Mesophilic crenarchaeota: proposal for a third archaeal phylum, the Thaumarchaeota. Nat. Rev. Microbiol. 6, 245–252. doi:10.1038/nrmicro1852
Busquet, M., Calsamiglia, S., Ferret, A., Cardozo, P. W., and Kamel, C. (2005). Effects of cinnamaldehyde and garlic oil on rumen microbial fermentation in a dual flow continuous culture. J. Dairy Sci. 88 (7), 2508–2516. doi:10.3168/jds.S0022-0302(05)72928-3
Candyrine, S. C. L., Mahadzir, M. F., Garba, S., Jahromi, M. F., Ebrahimi, M., Goh, Y. M., et al. (2018). Effects of naturally-produced lovastatin on feed digestibility, rumen fermentation, microbiota and methane emissions in goats over a 12-week treatment period. PLoS 13 (7), 1–19. doi:10.1371/journal.pone.0199840
Capelari, M., and Powers, W. (2017). The effect of nitrate and monensin on in vitro ruminal fermentation. J. Anim. Sci. 95 (11), 5112–5123. doi:10.2527/jas2017.1657
Castro, E. (2020). Biorefineries: a step forward to a circular bioeconomy. Biofuels, Bioprod. Bioref. 14, 5–6. doi:10.1002/bbb.2082
Cottyn, B. G., and Boucque, C. V. (1968). Rapid method for the gas-chromatographic determination of volatile fatty acids in rumen fluid. J. Agric. Food Chem. 16 (1), 105–107. doi:10.1021/jf60155a002
Dangal, S. R. S., Tian, H., Zhang, B., Pan, S., Lu, C., and Yang, J. (2017). Methane emission from global livestock sector during 1890-2014: magnitude, trends and spatiotemporal patterns. Glob. Chang Biol. 23 (10), 4147–4161. doi:10.1111/gcb.13709
Danielsson, R., Dicksved, J., Sun, L., Gonda, H., Müller, B., Schnürer, A., et al. (2017). Methane production in dairy cows correlates with rumen methanogenic and bacterial community structure. Front. Microbiol. 8, 226–315. doi:10.3389/fmicb.2017.00226
Danielsson, R., Ramin, M., Bertilsson, J., Lund, P., and Huhtanen, P. (2017b). Evaluation of a gas in vitro system for predicting methane production in vivo. J. Dairy Sci. 100, 8881–8894. doi:10.3168/jds.2017-12675
DOF Diario Oficial de la Federación (2001). Norma Oficial Mexicana NOM-062-ZOO-1999, especificaciones técnicas para la producción, cuidado y uso de los animales de laboratorio. Mexican Official Regulation NOM-062-ZOO-1999 Technical specifications fo the production, handling, and use of laboratory animals. Available at: http://dof.gob.mx/ (Accessed July 11, 2018).
El Kaoutari, A., Armougom, F., Gordon, J. I., Raoult, D., and Henrissat, B. (2013). The abundance and variety of carbohydrate-active enzymes in the human gut microbiota. Nat. Rev. Microbiol. 11 (7), 497–504. doi:10.1038/nrmicro3050
Emerson, E. L., and Weimer, P. J. (2017). Fermentation of model hemicelluloses by Prevotella strains and Butyrivibrio fibrisolvens in pure culture and in ruminal enrichment cultures. Appl. Microbiol. Biotechnol. 101 (10), 4269–4278. doi:10.1007/s00253-017-8150-7
Escamilla-Alvarado, C., Poggi-Varaldo, H. M., and Ponce-Noyola, M. T. (2017). Bioenergy and bioproducts from municipal organic waste as alternative to landfilling: a comparative life cycle assessment with prospective application to Mexico. Environ. Sci. Pollut. Res. 24 (33), 25602–25617. doi:10.1007/s11356-016-6939-z
Escamilla-Alvarado, C., Ponce-Noyola, M. T., Poggi-Varaldo, H. M., Ríos-Leal, E., García-Mena, J., and Rinderknecht-Seijas, N. (2014). Energy analysis of in-series biohydrogen and methane production from organic wastes. Int. J. Hydrogen Energ. 39 (29), 16587–16594. doi:10.1016/j.ijhydene.2014.06.077
Faseleh Jahromi, M., Liang, J. B., Ho, Y. W., Mohamad, R., Goh, Y. M., Shokryazdan, P., et al. (2013a). Lovastatin in Aspergillus terreus: fermented rice straw extracts interferes with methane production and gene expression in Methanobrevibacter smithii. Biomed. Res. Int. 2013, 604721. doi:10.1155/2013/604721
Faseleh Jahromi, M., Liang, J. B., Mohamad, R., Goh, Y. M., Shokryazdan, P., and Ho, Y. W. (2013b). Lovastatin-enriched rice straw enhances biomass quality and suppresses ruminal methanogenesis. Biomed. Res. Int. 2013, 397934. doi:10.1155/2013/397934
Fava, F., Totaro, G., Diels, L., Reis, M., Duarte, J., Carioca, O. B., et al. (2015). Biowaste biorefinery in Europe: opportunities and research & development needs. N. Biotechnol. 32 (1), 100–108. doi:10.1016/j.nbt.2013.11.003
Goel, G., Dagar, S. S., Raghav, M., and Bansal, S. (2015). “Chapter 17. Rumen: an underutilised niche for industrially important enzymes,” in Rumen microbiology: from evolution to revolution. Editors A. K. Puniya, R. Singh, and D. N. Kamrapp (New Delhi, India: Springer), 247–264.
Guyader, J., Tavendale, M., Martin, C., and Muetzel, S. (2016). Dose-response effect of nitrate on hydrogen distribution between rumen fermentation end products: an in vitro approach. Anim. Prod. Sci. 56 (3), 224–230. doi:10.1071/an15526
Havlík, P., Valin, H., Herrero, M., Obersteiner, M., Schmid, E., Rufino, M. C., et al. (2014). Climate change mitigation through livestock system transitions. Proc. Natl. Acad. Sci. USA 111 (10), 3709–3714. doi:10.1073/pnas.1308044111
Henderson, G., Cox, F., Ganesh, S., Jonker, A., Young, W., Janssen, P. H., et al. (2015). Rumen microbial community composition varies with diet and host, but a core microbiome is found across a wide geographical range. Sci. Rep. 5, 14567. doi:10.1038/srep14567
Hill, T. C., Walsh, K. A., Harris, J. A., and Moffett, B. F. (2003). Using ecological diversity measures with bacterial communities. FEMS Microbiol. Ecol. 43 (1), 1–11. doi:10.1111/j.1574-6941.2003.tb01040.x
Jain, S., Caforio, A., and Driessen, A. J. (2014). Biosynthesis of archaeal membrane ether lipids. Front. Microbiol. 5, 641. doi:10.3389/fmicb.2014.00641
Jirasatid, S., Nopharatana, M., Kitsubun, P., Vichitsoonthonkul, T., and Tongta, A. (2013). Statistical optimization for Monacolin K and yellow pigment production and citrinin reduction by Monascus purpureus in solid-state fermentation. J. Microbiol. Biotechnol. 23 (3), 364–374. doi:10.4014/jmb.1206.06068
Khonkhaeng, B., and Cherdthong, A. (2020). Improving nutritive value of purple field corn residue and rice straw by culturing with white-rot fungi. JoF 6 (2), 69. doi:10.3390/jof6020069
Kim, S., and Dale, B. E. (2004). Global potential bioethanol production from wasted crops and crop residues. Biomass and Bioenergy 26 (4), 361–375. doi:10.1016/j.biombioe.2003.08.002
Lê, S., Josse, J., and Husson, F. (2008). FactoMineR: an R package for multivariate analysis. J. Stat. Softw. 25 (1), 1–18. doi:10.18637/jss.v025.i01
Leo, T. K., Garba, S., Abubakar, D., Sazili, A. Q., Candyrine, S. C. L., Jahromi, M. F., et al. (2020). Naturally produced lovastatin modifies the histology and proteome profile of goat skeletal muscle. Animals. 10, 72. doi:10.3390/ani10010072
Mao, S. Y., Zhang, R. Y., Wang, D. S., and Zhu, W. Y. (2013). Impact of subacute ruminal acidosis (SARA) adaptation on rumen microbiota in dairy cattle using pyrosequencing. Anaerobe 24, 12–19. doi:10.1016/j.anaerobe.2013.08.003
Martin, M. (2011). Cutadapt removes adapter sequences from high-throughput sequencing reads. EMBnet j. 17 (1), 10–12. doi:10.14806/ej.17.1.200
Martinez-Fernandez, G., Denman, S. E., Yang, C., Cheung, J., Mitsumori, M., and McSweeney, C. S. (2016). Methane inhibition alters the microbial community, hydrogen flow, and fermentation response in the rumen of cattle. Front. Microbiol. 7, 1122–1214. doi:10.3389/fmicb.2016.01122
Masters, B. A., Palmoski, M. J., Flint, O. P., Gregg, R. E., Wang-Iverson, D., and Durham, S. K. (1995). In vitro myotoxicity of the 3-hydroxy-3-methylglutaryl coenzyme a reductase inhibitors, pravastatin, lovastatin, and simvastatin, using neonatal rat skeletal myocytes. Toxicol. Appl. Pharmacol. 131, 163–174. doi:10.1006/taap.1995.1058
Menke, K. H., and Steingass, H. (1988). Estimation of energetic feed value obtained from chemical analysis and in vitro gas production using rumen fluid. Anim. Res. Dev. 28, 7–55.
Mi, L., Yang, B., Hu, X., Luo, Y., Liu, J., Yu, Z., et al. (2018). Comparative analysis of the microbiota between sheep rumen and rabbit cecum provides new insight into their differential methane production. Front. Microbiol. 9, 575–614. doi:10.3389/fmicb.2018.00575
Miller, T. L., and Wolin, M. J. (2001). Inhibition of growth of methane-producing bacteria of the ruminant forestomach by hydroxymethylglutaryl-SCoA reductase inhibitors. J. Dairy Sci. 84 (6), 1445–1448. doi:10.3168/jds.s0022-0302(01)70177-4
Morgavi, D. P., Martin, C., and Boudra, H. (2013). Fungal secondary metabolites from Monascus spp. reduce rumen methane production in vitro and in vivo. J. Anim. Sci. 91 (2), 848–860. doi:10.2527/jas.2012-5665
Muñoz-Páez, K., Poggi-Varaldo, H., García-Mena, J., Ponce-Noyola, M., Ramos-Valdivia, A., Barrera-Cortés, J., et al. (2014). Cheese whey as substrate of batch hydrogen production: effect of temperature and addition of buffer. Waste Manag. Res. 32 (5), 434–440. doi:10.1177/0734242x14527333
Myer, P. R., Kim, M., Freetly, H. C., and Smith, T. P. (2016). Evaluation of 16S rRNA amplicon sequencing using two next-generation sequencing technologies for phylogenetic analysis of the rumen bacterial community in steers. J. Microbiol. Methods 127, 132–140. doi:10.1016/j.mimet.2016.06.004
Nkamga, V. D., Henrissat, B., and Drancourt, M. (2017). Archaea: essential inhabitants of the human digestive microbiota. Hum. Microbiome J. 3, 1–8. doi:10.1016/j.humic.2016.11.005
O’Brien, M., Navarro-Villa, A., Purcell, P. J., Boland, T. M., and O’Kiely, P. (2014). Reducing in vitro rumen methanogenesis for two contrasting diets using a series of inclusion rates of different additives. Anim. Prod. Sci. 54 (2), 141–157. doi:10.1071/AN1220410.1071/anv54n6_fo
Poggi-Varaldo, H. M., Munoz-Paez, K. M., Escamilla-Alvarado, C., Robledo-Narváez, P. N., Ponce-Noyola, M. T., Calva-Calva, G., et al. (2014). Biohydrogen, biomethane and bioelectricity as crucial components of biorefinery of organic wastes: a review. Waste Manag. Res. 32 (5), 353–365. doi:10.1177/0734242X14529178
Prévot, V., Lopez, M., Copinet, E., and Duchiron, F. (2013). Comparative performance of commercial and laboratory enzymatic complexes from submerged or solid-state fermentation in lignocellulosic biomass hydrolysis. Bioresour. Technol. 129, 690–693. doi:10.1016/j.biortech.2012.11.135
Puniya, A. K., Singh, R., and Kamra, D. N. (2015). Rumen microbiology: from evolution to revolution. New Delhi, India: Springer.
Ramírez-Restrepo, C. A., O'Neill, C. J., López-Villalobos, N., Padmanabha, J., and Mcsweeney, C. (2014). Tropical cattle methane emissions: The role of natural statins supplementation. Anim. Prod. Sci. 54, 1294–1299.doi:10.1071/AN14246
Rideout, J. R., Dillon, M. R., Bokulich, N. A., Abnet, C., Ghalith, G. A. Al., Alexander, H., et al. (2018). Qiime 2 : reproducible. interactive. scalable. and extensible microbiome data science. PeerJ PrePrints 37, 852–857. doi:10.7287/peerj.preprints.27295
Rognes, T., Flouri, T., Nichols, B., Quince, C., and Mahé, F. (2016). VSEARCH: a versatile open source tool for metagenomics. PeerJ 4, e2584, doi:10.7717/peerj.2584
Royal Society-Royal Academy of Engineering (2018). Greenhouse gas removal. London, UK: Royal Society-Royal Academy of Engineering, 164. Available at: royalsociety.org/greenhouse-gas-removal raeng.org.uk/greenhousegasremoval.
Ruiz, O., Castillo, Y., Arzola, C., Burrola, E., Salinas, J., Corral, A., et al. (2016). Effects of Candida norvegensis live cells on in vitro oat straw rumen fermentation. Asian-australas. J. Anim. Sci. 29 (2), 211–218. doi:10.5713/ajas.15.0166
SAGARPA (2017). Secretaria de Agricultura, Ganadería, Desarrollo Rural, Pesca y Alimentación. Planeación Agrícola Nacional 2017–2030. Avena forrajera en México. Forage oat in México. Available at: https://www.gob.mx/agricultura (Accessed January 25, 2020).
Sharma, A., Chaudhary, P. P., Sirohi, S. K., and Saxena, J. (2011). Structure modeling and inhibitor prediction of NADP oxidoreductase enzyme from Methanobrevibacter smithii. Bioinformation 6 (1), 15–19. doi:10.6026/97320630006015
Shen, J., Liu, Z., Yu, Z., and Zhu, W. (2017). Monensin and nisin affect rumen fermentation and microbiota differently in vitro. Front. Microbiol. 8, 1–13. doi:10.3389/fmicb.2017.01111
Soliva, C. R., Amelchanka, S. L., Duval, S. M., and Kreuzer, M. (2011). Ruminal methane inhibition potential of various pure compounds in comparison with garlic oil as determined with a rumen simulation technique (Rusitec). Br. J. Nutr. 106, 114–122. doi:10.1017/S0007114510005684
Sparling, R., Risbey, D., and Poggi-Varaldo, H. M. (1997). Hydrogen production from inhibited anaerobic composters. Int. J. Hydrogen Energ. 22, 563–566. doi:10.1016/s0360-3199(96)00137-1
Tao, S., Tian, P., Luo, Y., Tian, J., Hua, C., Geng, Y., et al. (2017). Microbiome-metabolome responses to a high-grain diet associated with the hind-gut health of goats. Front. Microbiol. 8, 1764–1771. doi:10.3389/fmicb.2017.01764
Ungerfeld, E. M. (2015). Shifts in metabolic hydrogen sinks in the methanogenesis-inhibited ruminal fermentation: a meta-analysis. Front. Microbiol. 6, 1–17. doi:10.3389/fmicb.2015.00538
US-EPA (2014). Climate change indicators in the United States 2014. 3 edn.Available at: www.epa.gov/climatechange/indicators. Tables 1 and 2 (Accessed November 15, 2020).
Van Soest, P. J., Robertson, J. B., and Lewis, B. A. (1991). Methods for dietary fiber, neutral detergent fiber, and nonstarch polysaccharides in relation to animal nutrition. J. Dairy Sci. 74, 3583–3597. doi:10.3168/jds.S0022-0302(91)78551-2
Wang, L. Z., Zhou, M. L., Wang, J. W., Wu, D., and Yan, T. (2016). The effect of dietary replacement of ordinary rice with red yeast rice on nutrient utilization, enteric methane emission and rumen archaeal diversity in goats. PLoS One 11 (7), e0160198. doi:10.1371/journal.pone.0160198
Weimer, P. J. (1998). Manipulating ruminal fermentation: a microbial ecological perspective. J. Anim. Sci. 76 (12), 3114–3122. doi:10.2527/1998.76123114x
Weimer, P. M. (2015). “Chapter 18. Ruminal fermentations to produce liquid and gaseous fuels,” in Rumen microbiology: from evolution to revolution. Editors A. K. Puniya, R. Singh, and D. N. Kamrapp (New Delhi, India: Springer), 265–280.
Weiss, S., Xu, Z. Z., Peddada, S., Amir, A., Bittinger, K., Gonzalez, A., et al. (2017). Normalization and microbial differential abundance strategies depend upon data characteristics. Microbiome 5 (1), 27–18. doi:10.1186/s40168-017-0237-y
Wu, S., Baldwin, R. L., Li, W., Li, C., Connor, E. E., and Li, R. W. (2012). The bacterial community composition of the bovine rumen detected using pyrosequencing of 16S rRNA genes. Metagenomics 1, 1–11. doi:10.4303/mg/235571
Xu, B. J., Wang, Q. J., Jia, X. Q., and Sung, C. K. (2005). Enhanced lovastatin production by solid state fermentation of Monascus ruber. Biotechnol. Bioproc. Eng. 10, 78–84. doi:10.1007/bf02931187
Yáñez-Ruiz, D. R., Bannink, A., Dijkstra, J., Kebreab, E., Morgavi, D. P., O’Kiely, P., et al. (2016). Design, implementation and interpretation of in vitro batch culture experiments to assess enteric methane mitigation in ruminants-a review. Anim. Feed Sci. Technology 216, 1–18. doi:10.1016/j.anifeedsci.2016.03.016
Yang, D. J., and Hwang, L. S. (2006). Study on the conversion of three natural statins from lactone forms to their corresponding hydroxy acid forms and their determination in Pu-Erh tea. J. Chromatogr. A. 1119 (12), 277–284. doi:10.1016/j.chroma.2005.12.031
Zheng, M., Zuo, S., Niu, D., Jiang, D., Tao, Y., and Xu, C. (2020). Effect of four species of white rot fungi on the chemical composition and in vitro rumen degradability of naked oat straw. Waste and Biomass Valorization 12, 435–443. doi:10.1007/s12649-020-00991-w
Zhou, M., Chen, Y., and Guan, L. L. (2015). “Rumen bacteria,” in Rumen microbiology: from evolution to revolution. Editors A. K. Puniya, R. Singh, and D. N. Kamra, 79–98. doi:10.1007/978-81-322-2401-3_6
Zhou, R., Wu, J., Lang, X., Liu, L., Casper, D. P., Wang, C., et al. (2020). Effects of oregano essential oil on in vitro ruminal fermentation, methane production, and ruminal microbial community. J. Dairy Sci. 103 (3), 2303–2314. doi:10.3168/jds.2019-16611
Keywords: lovastatin, fermented oat straw, rumen, methanogenesis, microbiota
Citation: Ábrego-Gacía A, Poggi-Varaldo HM, Mendoza-Vargas A, Mercado-Valle FG, Ríos-Leal E, Ponce-Noyola T and Calva-Calva G (2021) Effects of Fermented Oat Straw as a Lovastatin Carrier on in vitro Methane Production and Rumen Microbiota. Front. Energy Res. 9:630701. doi: 10.3389/fenrg.2021.630701
Received: 18 November 2020; Accepted: 15 February 2021;
Published: 21 April 2021.
Edited by:
Eulogio Castro, University of Jaén, SpainReviewed by:
Mahdi Ebrahimi, Shahid Beheshti University, IranObulisamy Parthiba Karthikeyan, University of Houston, United States
Saleem Javed, Aligarh Muslim University, India
Copyright © 2021 Ábrego-Gacía, Poggi-Varaldo, Mendoza-Vargas, Mercado-Valle, Ríos-Leal, Ponce-Noyola and Calva-Calva. This is an open-access article distributed under the terms of the Creative Commons Attribution License (CC BY). The use, distribution or reproduction in other forums is permitted, provided the original author(s) and the copyright owner(s) are credited and that the original publication in this journal is cited, in accordance with accepted academic practice. No use, distribution or reproduction is permitted which does not comply with these terms.
*Correspondence: Héctor M. Poggi-Varaldo, cjRjZXBlQHlhaG9vLmNvbQ==