- 1Academy of Agricultural Planning and Engineering, Key Laboratory of Energy Resource Utilization from Agriculture Residue, Ministry of Agriculture, Beijing, China
- 2Institute of Environment and Sustainable Development in Agriculture, Chinese Academy of Agricultural Sciences, Beijing, China
- 3Department of Agricultural and Biological Engineering, University of Illinois at Urbana–Champaign, Champaign, IL, United States
Microbial electrolysis cell (MEC) has emerged as the promising technology for COD removal as well as bioenergy recovery during the treatment of bio-refractory wastewaters. This study mainly focused on wood vinegar (the by-product from biomass pyrolysis) treatment via MEC technology with two typical biochars (coconut shell biochar and shrub biochar) as the carriers of microorganisms in anode chamber. Results indicated that MECs with coconut shell biochar had an obvious beneficial effect for treating wood vinegar, with COD removal reached up to 71.4%. GC-MS analysis showed that furfurals present in the wood vinegar were thoroughly degraded after MEC treatment. One interesting finding is that hydrocarbons accounted for a large portion of the compounds in the effluent, which may be the comprehensive result of complex organic reactions, including decarboxylation reactions, dehydration reaction, etc. The dominant microbial populations in MEC with biochar anode mainly included Geobacter, Macellibacteroides, Oscillibacter, Sedimentibacter, Comamonas, and Lachnoclostridium. This study demonstrated that pyrolysis biochar could be incorporated as a high-efficiency MEC anode material, and MECs with the inclusion of biochar could provide a feasible way for the treatment of recalcitrant wood vinegar.
Introduction
In recent years, pyrolysis has attracted a great amount of attention as a valuable waste treatment method (Gascó et al., 2018). Pyrolysis is a thermochemical process which is normally performed at high temperatures (300–700°C and above) with an absence of oxygen. The main products of pyrolysis are biochar and bio-oil (Chiaramonti et al., 2017). Meanwhile, a large amount of bio-products are produced after the pyrolysis process; the major by-product is wood vinegar. Wood vinegar is collected by separating the liquid product from the pyrolysis of wood biomass (Wu Q. et al., 2015; Sun et al., 2018). The yield of wood vinegar is over 20% among the pyrolysis products. This signifies that when 1000 kg of feedstock is treated, more than 200 kg of wood vinegar will be produced (Ratanapisit et al., 2009; Wu Q. et al., 2015). Wood vinegar with its high concentration of organic compounds will pollute the environment when released without any treatment; therefore, it is necessary to explore pathways to treat the excess wood vinegar.
There are more than 200 kinds of compounds in wood vinegar (Liu et al., 2018), and these compounds mainly consist of organic acids, alcohols, phenols, ketones and other organics. Generally, organic acids are easily biodegradable, while alcohols, phenols and ketones are bio-refractory, so wood vinegar can be classified as one kind of bio-refractory wastewater. Now, the application of wood vinegar is very limited, only focused on agriculture and industry field (Wang et al., 2010; Hagner et al., 2013; Wu Q. et al., 2015; Nunkaew et al., 2018), while little attention has been paid on how to treat this wastewater despite its considerable volume produced post-pyrolysis. Microbial electrolysis cell (MEC) is an emerging technique to degrade the bio-refractory wastewater via a combination of microorganisms and electrochemistry (Goglio et al., 2019). Recently, MEC showed a good performance for the treatment of recalcitrant wastewaters like molasses wastewater (Sevda et al., 2013), biodiesel wastewater (Feng et al., 2011), textile wastewater (Nor et al., 2015), seafood processing wastewater (Jayashree et al., 2016), etc. Moreover, when a complex biorefinery (pyrolysis) stream or de-oiled wastewaters were used as the substrates of MECs, the COD removal rate could reach up to 79% (Ren et al., 2013; Lewis and Borole, 2016). More detailed advancements on the breakdown of complex organic pollutants via MECs can be found in recent reviews (Lu and Ren, 2016; Hua et al., 2019).
In this study, we investigate the feasibility of wood vinegar treatment via MECs. Two typical biochars from pyrolysis of waste biomass were used as microbial carrier materials to improve the utilization and transfer of electrons due to its abundant micropore structures and oxygen-containing functional groups. Key parameters were investigated, including substrate types, wood vinegar concentrations, and applied voltages. The removal of chemical oxygen demand (COD) and the degradation of organic compounds were explored before and after MEC treatment. Additionally, the diversity of the microbial community in MEC anode biofilm and inoculum through Illumina Miseq sequencing was conducted. This study not only provides a theoretical basis for refractory wood vinegar treatment via MECs, but it also supplies a new utilization pathway for widespread application of pyrolysis biochar as an MEC electrode material.
Materials and Methods
MEC Reactor Design and Operation
Two-chamber fixed-bed MEC reactors were made of poly-methyl-methacrylate and were operated in batch mode. The detailed composition of MEC system is described in Figure 1. For each reactor, the working volume of both the anode and the cathode chamber was 600 mL. The biochar was used as the microbial carrier of MEC anode chamber with a packed density of 400 mL based on the anodic working volume. Each anode consisted of a piece of carbon fiber brush (d × h = 6 cm × 14 cm) embedded in biochar with a titanium wire (d = 0.8 mm) used as a current collector. Two typical biochars were chosen, including coconut shell biochar and shrub biochar. R1 and R2 were two parallel reactors with coconut shell biochar, while R3 and R4 were two parallel reactors with shrub biochar. The pretreatment processes of biochar included sifting (10 mesh), washing with tap water (at least three times), in order to remove the surface dust and carbon, and finally soaking overnight with alcohol to kill its existing microbes. The cathode was a piece of nickel foam (6 cm × 14 cm), which was reported to exhibit excellent electrochemical activities for MEC (Patil et al., 2011). A cation exchange membrane (CEM) (Beijing Fengxiang Science & Technology Company) was used to separate the two chambers, and a 10 Ω resistor was added between the anode and cathode for current measurement.
The MEC reactors started with 1.64 g/L CH3COONa in 50 mM PBS buffer with the inoculum (v:v = 1:1). The inoculum of MECs was the mixture of anaerobic sludge and MFC effluent, which were obtained from an anaerobic digester at Xiaohongmen Municipal Wastewater Treatment Plant (Beijing, China) and from a working MFC at the Environment-Enhancing Energy (E2-Energy) laboratory in the College of Water Resources and Civil Engineering at University of China Agricultural University, respectively. A 1.0 V external voltage was applied to the MEC. The catholyte (50 mM PBS) was mixed using a magnetic stirrer and exposed to nitrogen for 20 min to remove the dissolved oxygen before use (Yang et al., 2017). After 2 months of operation, when the current output became stable, the substrate was changed to a 1:1 mixture of 1.64 g/L CH3COONa and artificial wastewater (3 g glucose, 0.046 g KH2PO4, 0.054 g MgSO4⋅7H2O, 0.004 g CaCl2⋅2H2O, 0.2 g NH4Cl, 0.32 g yeast/L) without the addition of vitamins and trace elements. After that, actual wood vinegar, with different concentrations adjusted with deionized water, was used as the substrate of MECs. The effects of substrates types (sodium acetate, artificial wastewater, wood vinegar), organic loadings of wood vinegar (1.257–3.126 g COD/L), and applied voltages (0.6–1.2 V) on the MEC reactor performance were characterized. When changing the anolyte, the catholyte was simultaneously changed using refresh 50 mM PBS solution. All MEC reactors were operated at room temperature (25 ± 2°C) and a certain hydraulic retention time (48 h).
Analytical Methods
Wood Vinegar Analytical Methods
The wood vinegar generated from corn straw pyrolysis was collected from the Center of Energy and Environmental Protection, Chinese Academy of Agricultural Engineering Planning & Design. Pyrolysis of corn straw was performed in a biomass carbonization moving bed with internal heating set to a temperature of 600°C. ORP, TDS and pH were measured with an ORP meter (SX-630, Shanghai Sanxin Instruments), a TDS meter (SX-620, Shanghai Sanxin Instruments) and a pH meter (SX-610, Shanghai Sanxin Instruments), respectively. Total solids (TS) were measured following the standard method (Lu et al., 2017). COD was analyzed using the kits provided by HACH Company. TOC was measured using a TOC analyzer (TOC-4200, Shimadzu Corporation). The concentrations of K, Ca, Mn, and Fe in wood vinegar were determined by Inductive Coupled Plasma Emission Spectrometer (ICPE-9000, Shimadzu, Japan) analysis method. An elemental analyzer was used to measure C, H, N, and S mass percents (Series II CHNS/O Analyzer 2400, PerkinElmer). The O content was calculated according to the following formula:
The concentrations of volatile fatty acids (VFAs) were measured using a gas chromatograph (Agilent 7820A, America). All the samples were filtered with a filter membrane of 0.22 μm before testing. Organic compounds in the wood vinegar were identified by gas chromatography-mass spectrometry (GC-MS) (Model QP2010, Shimadzu Company, Japan). The samples were extracted with ether in a volume ratio of 1:3 prior to testing.
Electrochemical Analytical Methods
The power source (PS-305DM, Hong Kong Longwei Company, China) was used to supply the suitable and small voltages for the MECs. For each MEC test, the voltage (U) across a 10 Ω resistor was measured continuously by a data acquisition system (model 2700, Keithley). The voltage of the external resistance was monitored every 60 s in order to calculate the current of the electric circuit, in which the equation I = U/R was used. The internal resistance of MEC system was measured using the electrochemical impedance spectroscopy (EIS) method through an electrochemical workstation (CHI660E, Shanghai Chenghua Company, China). EIS was tested at the established frequency, amplitude, and quiet time (0.01–100000 Hz, 0.01 V, and 2 s).
Microbial Analytical Methods
The diversity of the microbial community was analyzed using Illumina MiSeq sequencing. The microbial samples included the inoculum and the anodic biofilms attached to biochar in the MEC at its steady state. The main procedure included the extraction of DNA and the amplification of the specified gene regions by PCR. Primer 515F (5′-GTGCCAGCMGCCGCGG-3′) and primer 907R (5′-CCGTCAATTCMTTTRAGTTT-3′) were used to amplify the V3-V4 region of the 16 S rRNA gene of the bacteria. The raw sequence data were deposited into the Sequence Read Archive (SRA1) database of National Center for Biotechnology Information (NCBI) data center. And the SRA accession number was SRP223408. The phylogenetic affiliation was analyzed by Silva 1282 against the RDP Classifier3 using a confidence threshold of 70%.
Results
Characteristics of the Wood Vinegar Generated From Biomass Pyrolysis Process
Table 1 shows the characteristics of the wood vinegar, which had a high concentration of organics (TCOD = 103.8 ± 0.95 g/L) and consisted of the following VFAs: acetic acid (26.52 g/L), propionic acid (6.30 g/L), isobutyric acid (3.54 g/L), and n-pentanoic acid (2.07 g/L), all which amounted to 38.43 g COD/L. In addition, the TC of wood vinegar was 133.35 g/L, and the TOC content was 131.21 g/L, accounting for 98.4% of the TC. Because the original wood vinegar had an acidic pH of 3.3, it was adjusted to pH = 7.0 with 1M NaOH before being used as the MEC substrate, which was conducted in order to satisfy the normal growth needs for electroactive microorganisms (Lu et al., 2015, 2016). The positive ORP value of wood vinegar (139.67 mV) showed its high oxidation capability. The majority of the substances in wood vinegar were organic acids and other organic compounds. These compounds were predominantly covalent (non-conductive), and only a few organic acids had a weak conductivity, so the conductivity of these compounds was assumed to be negligible. Therefore, only the presence of a few ions in wood vinegar led to the TDS value of 35.0 g/L. In wood vinegar, the concentrations of K, Ca, Mn, and Fe were 1.643, 0.276, 0.033, and 0.001 g/L, respectively. In addition, organic elemental analysis showed that wood vinegar consisted of 11.21% C, 10.92% H, 0.07% N, 76.75% O and 1.05% S (based on mass). The high oxygen content in wood vinegar was due to the presence of numerous organic acids and abundant oxygen-containing heterocyclic compounds. Therefore, this wastewater has a high degree of unsaturation and is relatively unstable, making it easy to precipitate during the storage process.
MEC Performance on COD/TOC Removal of Wood Vinegar
Intuitively, the MEC influent (wood vinegar) was dark brown, while the MEC effluent was light yellow (Figure 2), therefore it could be inferred that organic compounds in wood vinegar were well degraded via MECs. In fact, according to Table 2 and Figure 3, it was feasible to treat a wood vinegar solution via MECs. Even, the MEC performance with coconut shell biochar was better than that with shrub biochar in terms of the processing capacity of wood vinegar. For instance, at the same substrate (wood vinegar) concentration, a shrub biochar MEC was significantly less effective at enhancing the COD removal rate in comparison to a coconut shell biochar MEC (59.79% vs. 71.44%). The MEC with coconut shell biochar utilized as the anode had a better treatment effect on the low-concentration wood vinegar, and the removal rate of COD was 71.4%, the effluent COD was 0.359 g COD/L, which conformed to the third-level sewage discharge standard of China. However, if the technology was used into potential practical application, it appeared a high dilution of the original wood vinegar is needed? Based on the disadvantage or limitation, we consider a better way should be adopted, namely other low COD-concentration wastewaters like domestic wastewater, beer wastewater, etc. could be mixed with wood vinegar, which make high-efficiency treatment of different types of wastewaters come true.
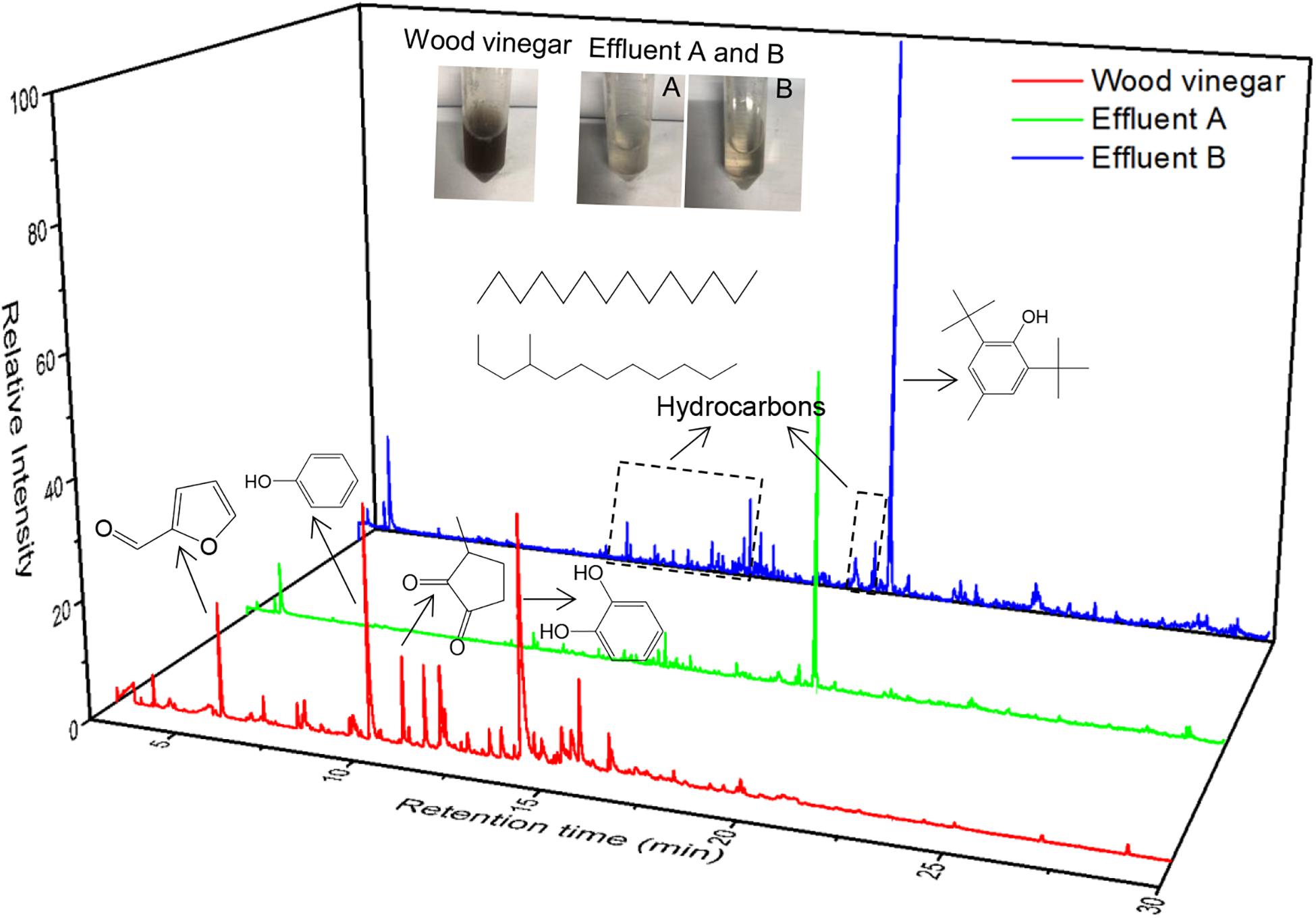
Figure 2. GC-MS analysis and physical pictures of the influent, effluent A, and effluent B. (Effluent A: under an influent concentration of 1.257 g COD/L; Effluent B: under an influent concentration of 2.316 g COD/L).
When coconut shell biochar was used as the anode, the COD removal rate ranged from 52.90 to 71.20% under different auxiliary voltages. When shrub biochar was used as the anode material, the COD removal rate ranged from 28.52 to 59.64% under different applied voltages. The COD removal rate in this study was positively correlated with applied voltages only within a certain range (0.6–1.0 V in this study). According to Figure 3, MECs showed different performances under different applied voltages, but higher applied voltages do not necessarily lead to a better MEC performance. When the applied voltage was 1.0 V, the highest COD removal rate was 71.20%. While the applied voltage was 1.2 V, the COD removal rate slightly decreased. Therefore, we believed that the optimal applied voltage in this system was 1.0 V.
The above results may be attributed to the characteristics of the biochar itself. The specific surface area of the coconut shell biochar was much larger than the shrub biochar (774.479 m2/g vs. 14.669 m2/g). As for the proportion of the different pore structures, the micropore structure of the coconut shell biochar accounted for 85.702%, which was higher than that of the shrub biochar (68.971%). More characteristics about the coconut shell biochar and the shrub biochar have been introduced in our previous study (Shen et al., 2020). A multi-microporous structure of biochar is more advantageous to microbial adhesion, and is therefore more conducive to the formation of a microbial biofilm.
However, compared with other substrates for MECs with coconut biochar, degradation performances of wood vinegar was inferior to the sodium acetate and artificial wastewater, and the degradation efficiency followed the order: sodium acetate (98.81%) > artificial wastewater (90.69%) > wood vinegar (71.44%). In addition, the absolute dominance of organic carbon in these substrates, so the TOC value and COD value showed a positive correlation, similarly the degradation rate of TOC was consistent with the degradation rate of COD, which was also verified by the data in Table 2. In the study, a good COD removal performance can be speculated to be due to the combined mechanism of microbial electrochemical degradation and adsorption of the initial anode biochar electrode.
MEC Performance on Organic Compounds Removal of Wood Vinegar
GC-MS analysis was performed to characterize the compounds present in the wood vinegar before and after MEC treatment (Figure 2). The detailed compounds detected via GC-MS are illustrated in Table 3. With respect to the compound carbon numbers, the short-chain compounds (<C6) and middle-chain compounds (C6–C12) in the influent occupied 67.68 and 32.32% of the total composition, respectively. However, the long-chain compounds (>C12) in the effluent (Effluent A) accounted for 88.06% and middle-chain compounds (C6, C7) constituted 11.94% of the total composition. Specifically, middle-chain compounds mainly included 48.0% Butylated Hydroxytoluene, 10.59% Hexadecane, 3.71% Dodecane, 4,6- dimethyl-, 2.56% Hexanedioic acid, bis(2-ethylhexyl) ester, 2.52% Phenol, 2,4-bis(1,1-dimethylethyl)-, 2.58% Decane, 3,7- dimethyl-, 2.09% Dodecane, etc. Thus, it can be concluded that short-chain compounds were completely degraded and middle-chain compounds were degraded efficiently. However, long-chain compounds were not effectively degraded, which led to a high-proportion of long-chain compounds in the effluent.
In addition, it was indicated that phenols (76.1%) were the dominant compounds present within the influent, and there was also small a small fraction of ketones, acids, esters (14.6%) and furfural derivatives (8.10%) present in the substrate. The existence of these compounds was corroborated by a previous study which emphasized the properties of wood vinegar, but there were some differences in the individual relative contents (Wu Q. et al., 2015). After MEC treatment, furfurals in the influent were thoroughly degraded. Ketones, acids, and esters were significantly degraded from 14.6% in the influent to 2.68% in the effluent. Phenols were also degraded to a certain extent from 76.1% in the influent to 50.1% in the effluent. In addition, the proportion of ethers increased a little bit compared with the influent. One interesting finding is that the hydrocarbons occupied 42.4 and 43.6% of the peak area in Effluent A and Effluent B, respectively. Hydrocarbons accounted for a large portion of the compounds in the effluent, which may be the result of complex organic reactions occurring during the transformation of organic compounds, including but not limited to the decarboxylation reaction, dehydration reaction, etc.
Electrochemical Properties of MECs With Different Substrate Types Under Applied Voltages
The electrochemical microorganisms in the anode chamber of the MEC were gradually enriched after the startup of the reactor, and the current value in the MEC system demonstrated a rising trend. As shown in Figure 4, the maximum current reached ∼20 mA when the MEC entered the stable period (2 months after startup of the MEC reactor). R1 and R2 were two parallel reactors using coconut shell biochar as MEC anode. The operation of the two reactors was stable and their performance was similar. The total internal resistances of both MEC reactors were small, amounting to 66 Ω and 72 Ω for R1 and R2, respectively (Supplementary Figure S1).
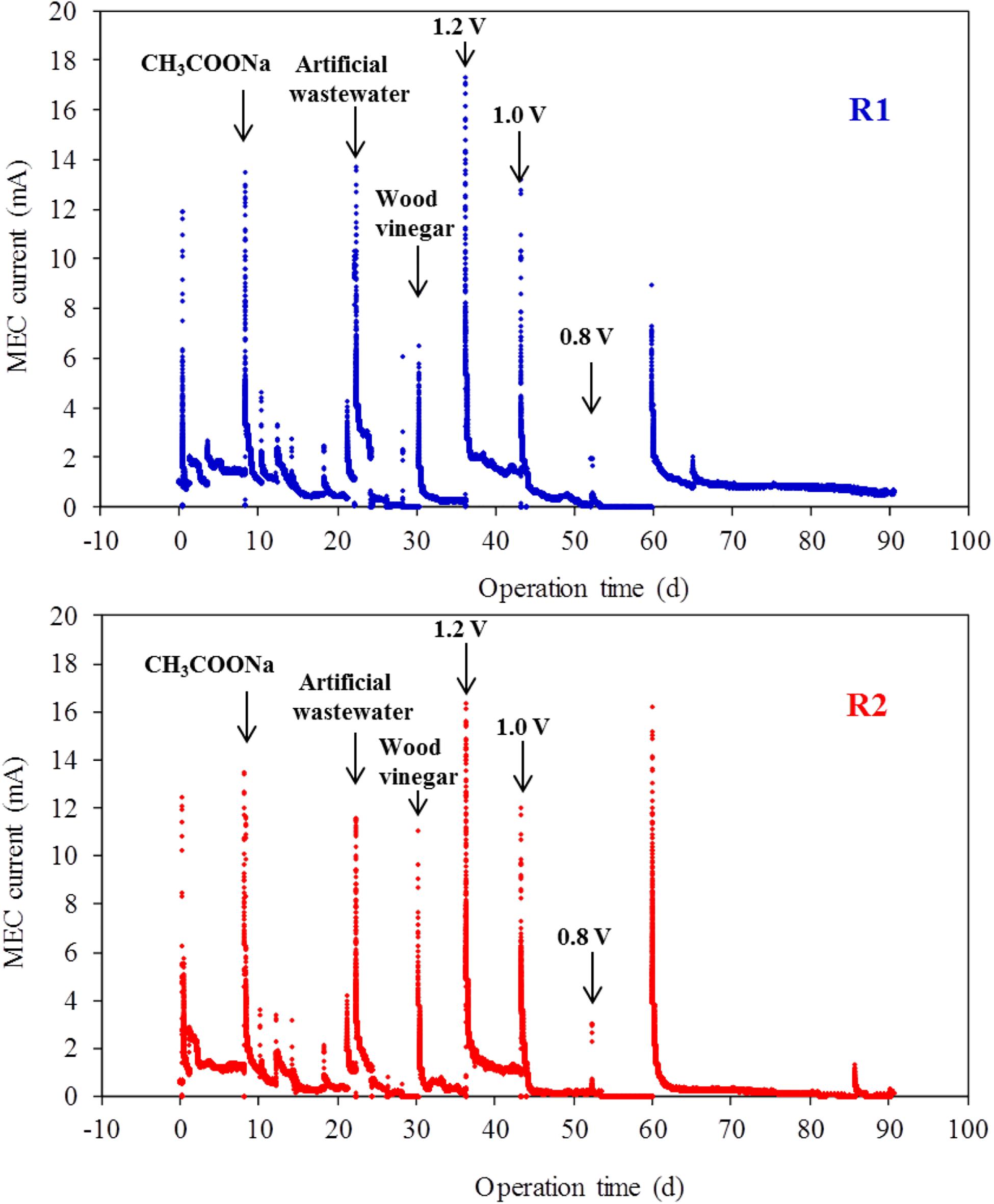
Figure 4. Current changes of MEC system with different substrates under applied voltages (R1 and R2 were two parallel reactors with coconut shell biochar incorporated as the anode).
At the beginning of batch reactions, upon the addition of substrates, the substrates were rapidly degraded by microorganisms and the system current reached a maximum value in a short time, which was consistent with a previous MEC study (Luo et al., 2019). This signified that MEC demonstrated good sensitivity to the addition of the substrate. Subsequently, with the continuous consumption of the substrates, the system current demonstrated a declining trend until the current reached close to 0 mA. The substrate and the applied voltage have great influence on the system current. At an applied voltage of 1.0 V, when the substrate was sodium acetate and artificial wastewater, the maximum current values of the system were 13.1 and 12.6 mA, respectively. However, when the substrate was wood vinegar, the maximum current value of the system was 8.65 mA (Figure 4). It can be seen that sodium acetate was the ideal substrate for the MEC using coconut shell biochar as the MEC anode. As the chemical complexity of the substrate composition increased, the degradation performance of organic compounds in the substrate became worse. In other words, some components of the complex substrate cannot be degraded and utilized by electrochemical flora. In addition, as the applied voltage decreased (ranging from 1.2 to 0.8 V), the maximum current value in the two parallel MEC systems demonstrated a decreasing trend (decreasing from 16.6 to 12.3 mA). In particular, there was a significant decrease in current when the applied voltage changed from 1.0 to 0.8 V. Therefore, the appropriate applied voltage in the system was 1.0 V.
Microbial Diversity Characteristics in MEC Anode and Inoculum
Here, Group A included A1 and A2, which represented MEC anode biofilm with coconut shell biochar filling. Group B included B1 and B2, which represented MEC anode biofilm with shrub biochar anode filling. Group C was the initial inoculum (anaerobic digestion sludge). Group D was a planktonic microorganism which existed on the surface of MEC anode. The gentle rarefaction curves demonstrated that the Illumina MiSeq sequencing data was reasonable (Supplementary Figure S2). Biological information including the statistical analysis of operational taxonomic units (OTUs) was carried out at a similarity level of 97%. The OTUs in the inoculum (913) were higher than the MEC anodic biofilm (613–695) (Table 4), revealing the lower species richness of bacteria in MEC, which was further supported by higher Shannon and Chao indexes. The phenomenon for this may be explained by the fact that under electrochemical acclimatization conditions, the superior electrochemical microorganisms were greatly enriched and other microbes acted on the principle of “survival of the fittest,” thus causing the microbial diversity to weaken.
To better characterize the common and unique OTU numbers and compare the overlap of these microorganisms, a Venn diagram was constructed (Supplementary Figure S3). Out of 613 microbial OTUs found in A1, 501 (81.7%) were shared with the A2 in Group A. This signified the existence of parallelism between two MEC reactors with coconut shell biochar utilized as the anode from a microbial diversity perspective. Similarly, in Group B with shrub biochar used the MEC anode, 85.2% of B1 was shared with B2. Conversely, common OTU numbers were lower between the inoculum and MEC anode biofilm. The reason for this similarity is the same as the aforementioned rationale.
The NMDS analysis at the OTU level indicated that A1 and A2 (Group A) were in one region, so the difference of the inter-group was not obvious. Similarly, B1 and B2 (Group B) were also in one region, so the difference of the inter-group was also not obvious (Figure 5). Further, there was no significant interior-group difference between Group A and Group B. Thus, it could be concluded that biochar type had no significant impact on the microbial community of MEC anode. On the contrary, Group C (the inoculum) was in another region compared with Group A and Group B, so there was a big difference among them. This was caused by the enrichment of the electrochemical microbes, which was in concert with previous explanations. This conclusion was also confirmed by a Cluster histogram, in which the left side is the gradation cluster analysis, the right side was a histogram of the community structure, the abscissa was the relative abundance of the species, and the ordinate was the sample name (Supplementary Figure S4).
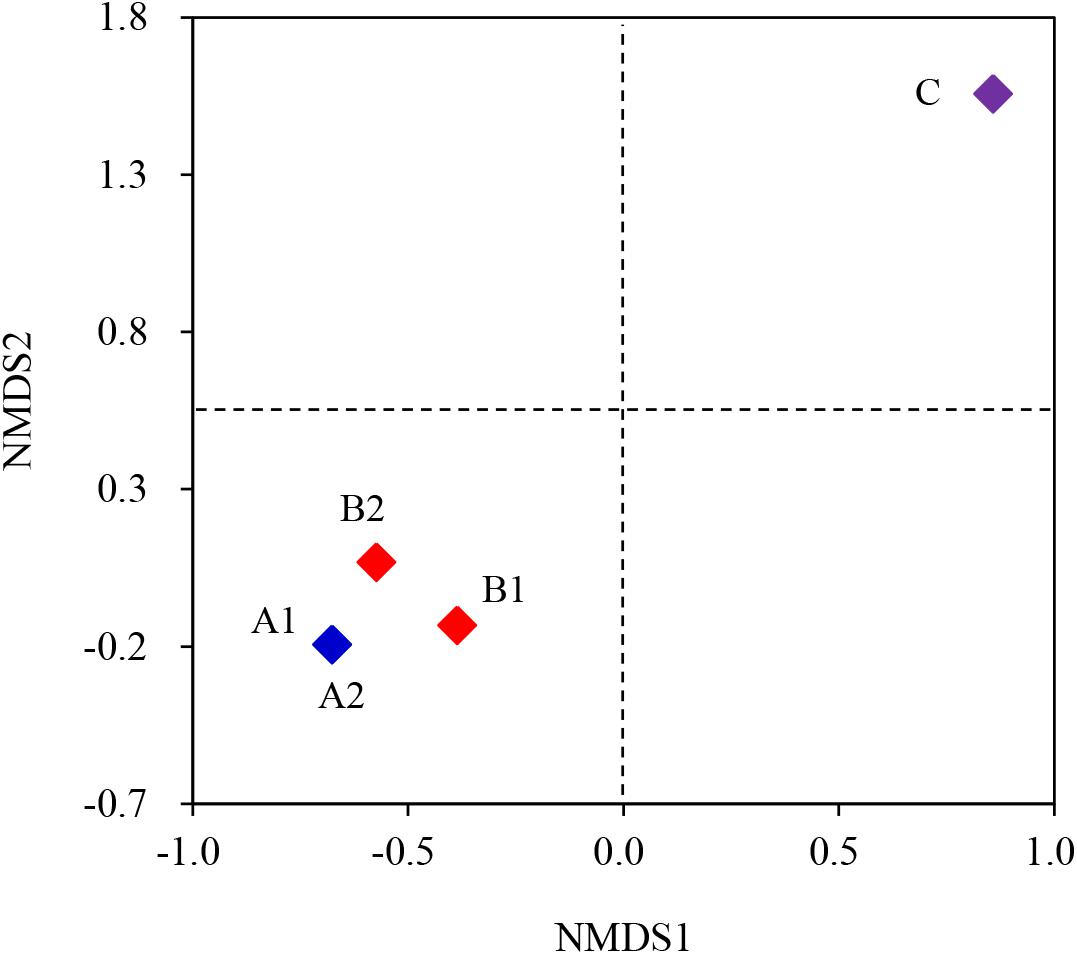
Figure 5. NMDS analysis at the OTU level of the inoculum and the MEC anodic biofilm. A1 and A2 represented MEC with coconut shell biochar; B1 and B2 represented MEC with shrub biochar; C was the initial inoculum.
Taxonomic analysis showed the phylogenetic differences between the inoculum and biofilm in MEC. A significantly different microbial diversity was observed based on genus-level classification between the groups. In this study, the microorganisms of MEC anode with coconut shell biochar (A1) mainly included Geobacter (10.78%), Macellibacteroides (9.47%), Oscillibacter (8.12%), Sedimentibacter (6.48%), Comamonas (4.35%), Clostridium (3.42%), and Lachnoclostridium (3.34%) (Figure 6). In addition, the proportion of unidentified and uncultured microbes decreased significantly from 58.89% (in inoculum) to 23.62% (in MEC anode). However, considerable unclassified microorganisms in the microbial community were not known, among which some new exoelectrogens may be present which have the ability to produce electrical current in MECs. For the shrub biochar anode MEC, the dominant microorganisms were similar to the MEC with coconut shell biochar.
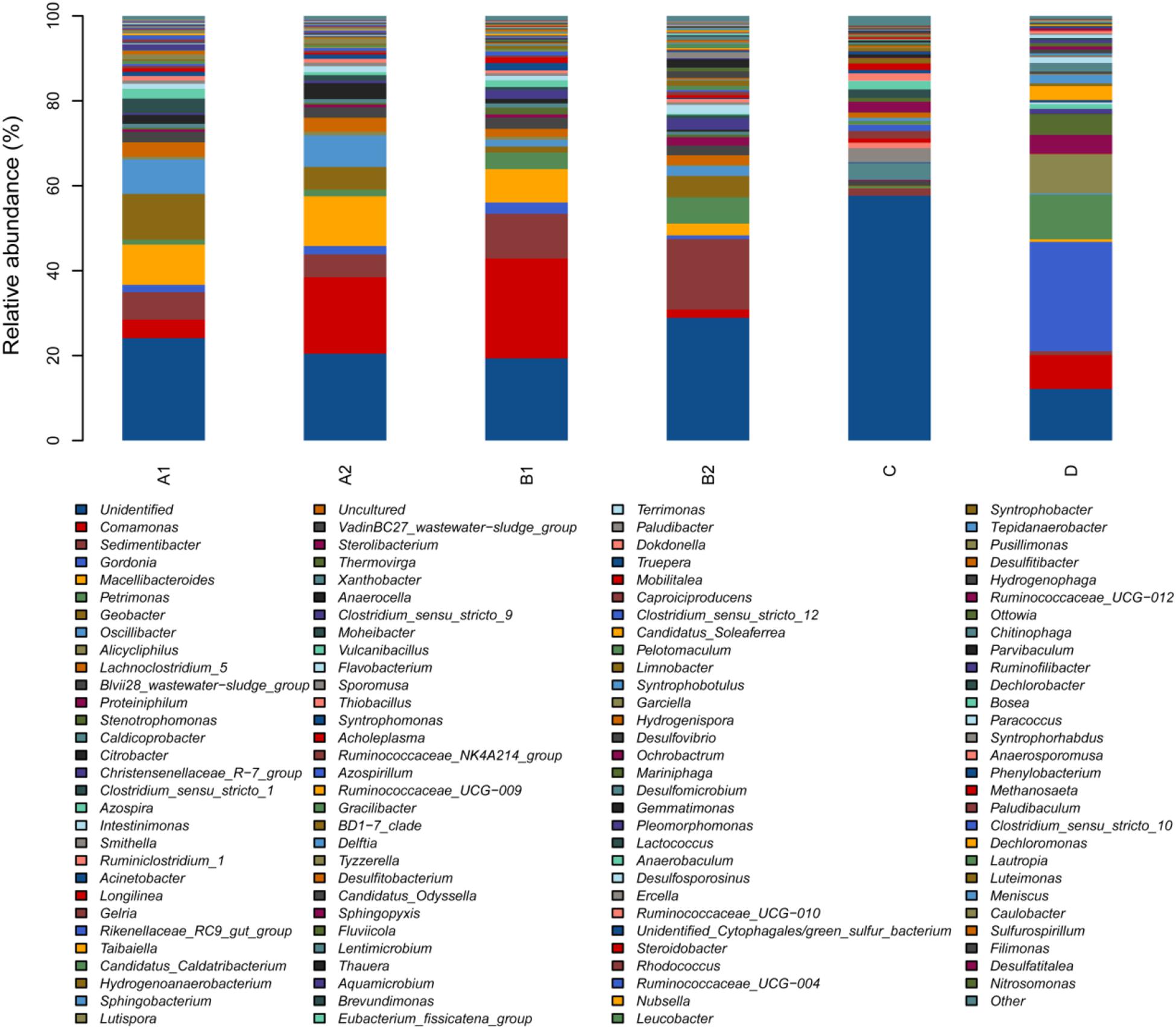
Figure 6. Taxonomic classification of microbial communities in both the inoculum and anodic biofilm in MEC at the genus level. All components less than 1% were classified as “others.” (A1) and (A2) represented MEC with coconut shell biochar; (B1) and (B2) represented MEC with shrub biochar; (C) represented the initial inoculum; (D) represented a planktonic microorganism which existed on the surface of MEC anode.
Specifically, the content of Geobacter in the inoculum was 0.21%, and it dramatically increased to 10.78% in MEC anode biofilm. This phenomenon was reasonable because the Geobacter genus is well known for its excellent electrochemical properties which often exist in MEC fed with acetate and other complex organics (Call et al., 2009; Zakaria et al., 2019). A previous study found that the proportions of Geobacter, Macellibacteroides, and Oscillibacter in MECs was 3.55, 1.52, and 1.80%, respectively, which were much lower than this study (10.78, 9.47, and 8.12%). This could be attributed to different niches in the diverse environment of a particular system (Lu et al., 2012; Wang et al., 2017). Except for Geobacter, Macellibacteroides, Oscillibacter, Sedimentibacter, and Comamonas were greatly enriched after MEC acclimatization. As previously reported, it has been confirmed that Comamonas can produce current in microbial electrochemical systems with acetate (Xing et al., 2010). In contrast to the above analysis, the contents of Smithella decreased significantly from 3.19% (in inoculum) to 0.71% (in MEC anode). Specifically, Sterolibacterium was not detected in MEC compared with having a content of 2.44% in the inoculum. The diversity of the microbial community in MEC anodic biofilm was associated with MEC substrate type (electron donor), i.e., sodium acetate, artificial wastewater, wood vinegar, etc.
Discussion
Microbial electrochemical reactors can effectively degrade refractory compounds, such as phenols or mixed hydrocarbons. Similar to previous studies (Luo et al., 2009; Wu S. et al., 2015; Wu et al., 2016), in this study the biochar selected as the anode material can also facilitate adsorption and promote the degradation of substances. For MEC treatment of wood vinegar, the COD removal of 71.4% in this study was higher than the reported value in a previous study (48%), in which MECs were fed with a pyrolysis stream derived from switchgrass (Lewis et al., 2015). This difference may be caused by multiple effects like substrate types, electrode materials, etc. However, as can be seen from Table 2, MEC degradation efficiency decreases with an increase of the wood vinegar concentration. When using high-concentration wood vinegar as MEC substrate, the removal rate of COD was less than 50%, and the effluent COD was more than 1 g COD/L. Thus, further work is needed to improve the endurance of exoelectrogens in MEC anode for wood vinegar through optimizing MEC configuration, etc.
Microbial growth requires appropriate applied voltages, and too high or too low voltages will affect its performance. In particular, a high applied voltage can not only increase the operating cost, but it can also affect the cell structure of microorganisms and even lead to the death of exoelectrogens. In addition, the optimal applied voltage for MECs is beneficial to the degradation of organic matter in the substrate, which depends on many factors, such as MEC configuration, substrate characteristics, key factors, microbial community diversity, etc. (Liu et al., 2010).
Generally, the internal resistance in the two-chamber MECs was higher than that of the single-chamber MEC because most of the protons were transferred through the exchange membrane in the middle of the two chambers (Lu and Ren, 2016; Hua et al., 2019). In addition, a higher internal resistance might be due to the poor electrical connection between the anode filling materials and the current collector (He et al., 2015). However, the internal resistance of the two-chamber MECs was much lower than that of typical single-chamber MECs with carbon cloth electrodes (∼200 Ω) (Miller et al., 2019). This may be due to the contribution of biochar addition in MEC anode, which greatly accelerated the transfer and utilization of electrons and organic matters, so the resistance of the MEC system was subsequently reduced.
Except for Lachnoclostridium, main microbes of MEC anode (Geobacter, Macellibacteroides, Oscillibacter, Sedimentibacter, Comamonas, and Clostridium) in this study are known to possess an exoelectrogenic activity, as demonstrated in previous studies (Call et al., 2009; Xing et al., 2010; Lu et al., 2012; Hari et al., 2016; Wang et al., 2017; Fujinawa et al., 2019; Zakaria et al., 2019). Substrate properties of MECs have important effect on microbial community structure. For instance, when propionate was used as MEC substrate, the content of Sedimentibacter was 4% (6.48% in the study) (Hari et al., 2016), and when waste activated sludge was used as MEC substrate, the content of Comamonas was 3% (4.35% in the study) (Lu et al., 2012). When wood vinegar was used as the MEC substrate, Lachnoclostridium (3.34%) was enriched, which has not been previously discovered in other MEC studies. Thus, it can be proposed that Lachnoclostridium might contributed to the degradation of wood vinegar, while its pathways and mechanism of extracellular electron transfer need to be further investigated.
Conclusion
It was feasible to treat recalcitrant wood vinegar from biomass pyrolysis via MEC technology with a high-efficiency. When wood vinegar was used as the substrate and the anode chamber of MECs were filled with coconut shell biochar, high COD removal was obtained (71.4%), and furfurals were thoroughly degraded. The dominant microbial populations in MEC anode with biochar were mainly Geobacter, Macellibacteroides, etc. Specifically, Lachnoclostridium may be correlated with the degradation of wood vinegar. Further work is needed to improve the endurance of exoelectrogens for treating wood vinegar.
Data Availability Statement
The datasets presented in this study can be found in online repositories. The names of the repository/repositories and accession number(s) can be found in the article/ Supplementary Material.
Author Contributions
RS: conceptualization, investigation, visualization, and original draft preparation. LZ: supervision, conceptualization, reviewing and editing, and funding acquisition. ZY: resources and reviewing and editing. JF: methodology and reviewing and editing. YJ: validation, investigation, and data curation. JW: reviewing and editing. All authors contributed to the article and approved the submitted version.
Funding
The work was supported by the China Postdoctoral Science Foundation (2018M641295), China Agriculture Research System (CARS-02), and Open Subject Based on Key Laboratory of the Ministry of Agriculture and Rural Affairs (KLERUAR2018-01).
Conflict of Interest
The authors declare that the research was conducted in the absence of any commercial or financial relationships that could be construed as a potential conflict of interest.
Supplementary Material
The Supplementary Material for this article can be found online at: https://www.frontiersin.org/articles/10.3389/fenrg.2020.00216/full#supplementary-material
Footnotes
References
Call, D. F., Wagner, R. C., and Logan, B. E. (2009). Hydrogen production by geobacter species and a mixed consortium in a microbial electrolysis cell. Appl. Environ. Microb. 75, 7579–7587. doi: 10.1128/aem.01760-09
Chiaramonti, D., Prussi, M., Buffi, M., Rizzo, A. M., and Pari, L. (2017). Review and experimental study on pyrolysis and hydrothermal liquefaction of microalgae for biofuel production. Appl. Energ. 185, 963–972. doi: 10.1016/j.apenergy.2015.12.001
Feng, Y., Yang, Q., Wang, X., Liu, Y., Lee, H., and Ren, N. (2011). Treatment of biodiesel production wastes with simultaneous electricity generation using a single-chamber microbial fuel cell. Bioresour. Technol. 102, 411–415. doi: 10.1016/j.biortech.2010.05.059
Fujinawa, K., Nagoya, M., Kouzuma, A., and Watanabe, K. (2019). Conductive carbon nanoparticles inhibit methanogens and stabilize hydrogen production in microbial electrolysis cells. Appl. Microbiol. Bio. 103, 6385–6392. doi: 10.1007/s00253-019-09946-1
Gascó, G., Paz-Ferreiro, J., Álvarez, M. L., Saa, A., and Méndez, A. (2018). Biochars and hydrochars prepared by pyrolysis and hydrothermal carbonisation of pig manure. Waste Manage. 79, 395–403. doi: 10.1016/j.wasman.2018.08.015
Goglio, A., Tucci, M., Rizzi, B., Colombo, A., Cristiani, P., and Schievano, A. (2019). Microbial recycling cells (MRCs): a new platform of microbial electrochemical technologies based on biocompatible materials, aimed at cycling carbon and nutrients in agro-food systems. Sci. Total Environ. 649, 1349–1361. doi: 10.1016/j.scitotenv.2018.08.324
Hagner, M., Penttinen, O., Tiilikkala, K., and Setälä, H. (2013). The effects of biochar, wood vinegar and plants on glyphosate leaching and degradation. Eur. J. Soil Biol. 58, 1–7. doi: 10.1016/j.ejsobi.2013.05.002
Hari, A. R., Katuri, K. P., Gorron, E., Logan, B. E., and Saikaly, P. E. (2016). Multiple paths of electron flow to current in microbial electrolysis cells fed with low and high concentrations of propionate. Appl. Microbiol. Biot. 100, 5999–6011. doi: 10.1007/s00253-016-7402-2
He, Y., Liu, Z., Xing, X., Li, B., Zhang, Y., Shen, R., et al. (2015). Carbon nanotubes simultaneously as the anode and microbial carrier for up-flow fixed-bed microbial fuel cell. Biochem. Eng. J. 94, 39–44. doi: 10.1016/j.bej.2014.11.006
Hua, T., Li, S., Li, F., Zhou, Q., and Ondon, B. S. (2019). Microbial electrolysis cell as an emerging versatile technology: a review on its potential application, advance and challenge. J. Chem. Technol. Biotechnol. 94, 1697–1711. doi: 10.1002/jctb.5898
Jayashree, C., Tamilarasan, K., Rajkumar, M., Arulazhagan, P., Yogalakshmi, K. N., Srikanth, M., et al. (2016). Treatment of seafood processing wastewater using upflow microbial fuel cell for power generation and identification of bacterial community in anodic biofilm. J. Environ. Manage. 180, 351–358. doi: 10.1016/j.jenvman.2016.05.050
Lewis, A. J., and Borole, A. P. (2016). Understanding the impact of flow rate and recycle on the conversion of a complex biorefinery stream using a flow-through microbial electrolysis cell. Biochem. Eng. J. 116, 95–104. doi: 10.1016/j.bej.2016.06.008
Lewis, A. J., Ren, S., Ye, X., Kim, P., Labbe, N., and Borole, A. P. (2015). Hydrogen production from switchgrass via an integrated pyrolysis–microbial electrolysis process. Bioresour. Technol. 195, 231–241. doi: 10.1016/j.biortech.2015.06.085
Liu, H., Hu, H., Chignell, J., and Fan, Y. (2010). Microbial electrolysis: novel technology for hydrogen production from biomass. Biofuels 1, 129–142. doi: 10.4155/bfs.09.9
Liu, L., Guo, X., Wang, S., Li, L., Zeng, Y., and Liu, G. (2018). Effects of wood vinegar on properties and mechanism of heavy metal competitive adsorption on secondary fermentation based composts. Ecotox. Environ. Safe. 150, 270–279. doi: 10.1016/j.ecoenv.2017.12.037
Lu, J., Liu, Z., Zhang, Y., Li, B., Lu, Q., Ma, Y., et al.. (2017). Improved production and quality of biocrude oil from low-lipid high-ash macroalgae Enteromorpha prolifera via addition of crude glycerol. J. Clean. Prod. 142, 749–757. doi: 10.1016/j.jclepro.2016.08.048
Lu, L., Hou, D., Fang, Y., Huang, Y., and Ren, Z. J. (2016). Nickel based catalysts for highly efficient H2 evolution from wastewater in microbial electrolysis cells. Electrochim. Acta 206, 381–387. doi: 10.1016/j.electacta.2016.04.167
Lu, L., and Ren, Z. J. (2016). Microbial electrolysis cells for waste biorefinery: a state of the art review. Bioresource Technol. 215, 254–264. doi: 10.1016/j.biortech.2016.03.034
Lu, L., Xing, D., and Ren, N. (2012). Pyrosequencing reveals highly diverse microbial communities in microbial electrolysis cells involved in enhanced H2 production from waste activated sludge. Water Res. 46, 2425–2434. doi: 10.1016/j.watres.2012.02.005
Lu, L., Xing, D., and Ren, Z. J. (2015). Microbial community structure accompanied with electricity production in a constructed wetland plant microbial fuel cell. Bioresour. Technol. 195, 115–121. doi: 10.1016/j.biortech.2015.05.098
Luo, H., Hu, J., Qu, L., Liu, G., Zhang, R., Lu, Y., et al. (2019). Efficient reduction of nitrobenzene by sulfate-reducer enriched biocathode in microbial electrolysis cell. Sci. Total Environ. 674, 336–343. doi: 10.1016/j.scitotenv.2019.04.206
Luo, H., Liu, G., Zhang, R., and Jin, S. (2009). Phenol degradation in microbial fuel cells. Chem. Eng. J. 147, 259–264. doi: 10.1016/j.cej.2008.07.011
Miller, A., Singh, L., Wang, L., and Liu, H. (2019). Linking internal resistance with design and operation decisions in microbial electrolysis cells. Environ. Int. 126, 611–618. doi: 10.1016/j.envint.2019.02.056
Nor, M. H. M., Mubarak, M. F. M., Elmi, H. S. A., Ibrahim, N., Wahab, M. F. A., and Ibrahim, Z. (2015). Bioelectricity generation in microbial fuel cell using natural microflora and isolated pure culture bacteria from anaerobic palm oil mill effluent sludge. Bioresource Technol. 190, 458–465. doi: 10.1016/j.biortech.2015.02.103
Nunkaew, T., Kantachote, D., Chaiprapat, S., Nitoda, T., and Kanzaki, H. (2018). Use of wood vinegar to enhance 5-aminolevulinic acid production by selected Rhodopseudomonas palustris in rubber sheet wastewater for agricultural use. Saudi J. Biol. Sci. 25, 642–650. doi: 10.1016/j.sjbs.2016.01.028
Patil, S. A., Harnisch, F., Koch, C., Hübschmann, T., Fetzer, I., Carmona-Martínez, A. A., et al. (2011). Electroactive mixed culture derived biofilms in microbial bioelectrochemical systems: the role of pH on biofilm formation, performance and composition. Bioresource Technol. 102, 9683–9690. doi: 10.1016/j.biortech.2011.07.087
Ratanapisit, J., Apiraksakul, S., Rerngnarong, A., Chungsiriporn, J., and Bunyakarn, C. (2009). Preliminary evaluation of production and characterization of wood vinegar from rubberwood. Songklanakarin J. Sci. Technol. 31, 343–349.
Ren, L., Siegert, M., Ivanov, I., Pisciotta, J. M., and Logan, B. E. (2013). Treatability studies on different refinery wastewater samples using high-throughput microbial electrolysis cells (MECs). Bioresource Technol. 136, 322–328. doi: 10.1016/j.biortech.2013.03.060
Sevda, S., Dominguez-Benetton, X., Vanbroekhoven, K., De Wever, H., Sreekrishnan, T. R., and Pant, D. (2013). High strength wastewater treatment accompanied by power generation using air cathode microbial fuel cell. Appl. Energ. 105, 194–206. doi: 10.1016/j.apenergy.2012.12.037
Shen, R., Jing, Y., Feng, J., Luo, J., Yu, J., and Zhao, L. (2020). Performance of enhanced anaerobic digestion with different pyrolysis biochars and microbial communities. Bioresource Technol. 296:122354. doi: 10.1016/j.biortech.2019.122354
Sun, H., Feng, Y., Ji, Y., Shi, W., Yang, L., and Xing, B. (2018). N2O and CH4 emissions from N-fertilized rice paddy soil can be mitigated by wood vinegar application at an appropriate rate. Atmos. Environ. 185, 153–158. doi: 10.1016/j.atmosenv.2018.05.015
Wang, D., Han, Y., Han, H., Li, K., and Xu, C. (2017). Enhanced treatment of Fischer-Tropsch wastewater using up-flow anaerobic sludge blanket system coupled with micro-electrolysis cell: a pilot scale study. Bioresource Technol. 238, 333–342. doi: 10.1016/j.biortech.2017.04.056
Wang, Z., Lin, W., Song, W., and Yao, J. (2010). Preliminary investigation on concentrating of acetol from wood vinegar. Energ. Convers. Manage. 51, 346–349. doi: 10.1016/j.enconman.2009.09.031
Wu, Q., Zhang, S., Hou, B., Zheng, H., Deng, W., Liu, D., et al. (2015). Study on the preparation of wood vinegar from biomass residues by carbonization process. Bioresource Technol. 179, 98–103. doi: 10.1016/j.biortech.2014.12.026
Wu, S., Li, H., Zhou, X., Liang, P., Zhang, X., Jiang, Y., et al. (2016). A novel pilot-scale stacked microbial fuel cell for efficient electricity generation and wastewater treatment. Water Res. 98, 396–403. doi: 10.1016/j.watres.2016.04.043
Wu, S., Liang, P., Zhang, C., Li, H., Zuo, K., and Huang, X. (2015). Enhanced performance of microbial fuel cell at low substrate concentrations by adsorptive anode. Electrochim. Acta 161, 245–251. doi: 10.1016/j.electacta.2015.02.028
Xing, D., Cheng, S., Logan, B. E., and Regan, J. M. (2010). Isolation of the exoelectrogenic denitrifying bacterium Comamonas denitrificans based on dilution to extinction. Appl. Microbiol. Biot. 85, 1575–1587. doi: 10.1007/s00253-009-2240-0
Yang, N., Ren, Y., Li, X., and Wang, X. (2017). Effect of short-term alkaline intervention on the performance of buffer-free single-chamber microbial fuel cell. Bioelectrochemistry 115, 41–46. doi: 10.1016/j.bioelechem.2017.02.002
Keywords: microbial electrolysis cell, wood vinegar, biochar anode, recalcitrant wastewater treatment, microbial diversity
Citation: Shen R, Zhao L, Yao Z, Feng J, Jing Y and Watson J (2020) Efficient Treatment of Wood Vinegar via Microbial Electrolysis Cell With the Anode of Different Pyrolysis Biochars. Front. Energy Res. 8:216. doi: 10.3389/fenrg.2020.00216
Received: 04 July 2020; Accepted: 10 August 2020;
Published: 08 September 2020.
Edited by:
Yong Jiang, Fujian Agriculture and Forestry University, ChinaReviewed by:
Gefu Zhu, Institute of Urban Environment (CAS), ChinaZhu Xiangdong, Fudan University, China
Huijie Hou, Huazhong University of Science and Technology, China
Copyright © 2020 Shen, Zhao, Yao, Feng, Jing and Watson. This is an open-access article distributed under the terms of the Creative Commons Attribution License (CC BY). The use, distribution or reproduction in other forums is permitted, provided the original author(s) and the copyright owner(s) are credited and that the original publication in this journal is cited, in accordance with accepted academic practice. No use, distribution or reproduction is permitted which does not comply with these terms.
*Correspondence: Lixin Zhao, emhhb2xpeGluY2FhZUAxNjMuY29t