- 1School of Materials and Energy, Guangdong University of Technology, Guangzhou, China
- 2School of Materials Science and Engineering, Nanyang Technological University, Singapore, Singapore
Rechargeable aqueous zinc ion batteries (ZIBs) have attracted increasingly solicitude in the application of large-scale electrochemical energy storage system (EES) as a result of their low-price, high security and environment-friendly. The synthesis of mass-produced electrode materials and the exploration of their potential electrochemical properties are essential steps to achieve superior large-scale EES. In this work, the large-scale preparation of vanadium oxyphosphate hydrate (VOPO4⋅2H2O) cathode material with impressively zinc storage ability is successfully demonstrated. Specially, it exhibits a high specific capacity of 165 mAh g–1 at 0.05 A g–1, and prominent rate property (90 and 75 mAh g–1 at 2 and 5 A g–1, respectively), as well as stable cyclability of 76% after 1000 cycles under a high current density of 5 A g–1 within the voltage window of 0.4–1.6 V (versus Zn2+/Zn). Moreover, the VOPO4⋅2H2O not only spreads superiority in electrochemical performance, but also shows the advantages of scalable production based on simple controllable adjustment in synthesis, which is expected to exhibit great development potential in the field of large-scale EES application.
Introduction
With the aggravation of the serious energy crisis, it is particularly important to maximize energy utilization through energy storage and conversion technologies (Palanisamy et al., 2016; Guo Q. et al., 2020; Yang et al., 2020; Zhang S. et al., 2020). Rechargeable batteries recently have received tremendous solicitude as a result of their broad prospect and great potential in energy storage application such as portable electronic device, electric vehicles, and large-scale grid (Xia et al., 2018; Ao et al., 2019; Xiong et al., 2020). Due to the extremely finite resources and steeply rising prices of lithium and its increasingly concerned safety issues, lithium-ion batteries (LIBs) barely meet the growing energy demand and seriously hinder their further growing for large-scale electrochemical energy storage (EES) in the modern society (Chen L. et al., 2019; Lin et al., 2019; Li C. et al., 2020; Wang et al., 2020).
While, aqueous rechargeable alkaline metal ion batteries based on environmentally friendly water electrolytes give prominence to the merits of low price, good safety and high ion conductivity (two orders of magnitude higher than that of traditional non-aqueous rechargeable batteries), which in turn become the most promising alternatives to traditional LIBs in large-scale EES (Chen D. et al., 2019; Li G. et al., 2020). Among these rising aqueous rechargeable batteries, aqueous zinc ion batteries (ZIBs) are characterized as the potential representative owing to the low redox potential (−0.76 V vs. SHE.), high theoretical specific capacities (819 mAh g–1 and 5845 mAh cm–3), rich reserves (as the fourth “common” metal in the crust of earth), low cost and outstanding water compatibility of the zinc mental (Xia et al., 2017; Song et al., 2018; Li et al., 2019). However, the current available cathode materials of ZIBs deliver unsatisfactory performance [e.g., the low discharge capacity of Prussian blue analogs (Ke et al., 2017), the poor cycling stability of MnO2 polymorphs (Sun et al., 2017), etc.], seriously restricting the development and commercialization of aqueous ZIBs. Recently, vanadium-based electrode materials show impressive electrochemical properties in alkaline metal ion batteries because of their multiple valence changes and high electrochemical activity in chemical energy storage (Deng et al., 2020; Xie et al., 2020; Zhang X. et al., 2020). Among various vanadium-based electrode materials (Yue et al., 2016; Guo X. et al., 2020; Liu et al., 2020), layered VOPO4⋅2H2O has been widely concerned. This electrode material provides adjustable layer spacing to accommodate the intercalated zinc ions, and with the help of water, the zinc ions are more easily intercalated into the VOPO4⋅2H2O material (Huang et al., 2014; Peng et al., 2017; Tang et al., 2020). Additionally, the VOPO4⋅2H2O material can also store electric charge during anionic redox reaction process (Yang et al., 2020). There are many recent reports about VOPO4⋅2H2O electrode material applied in aqueous ZIBs, but it is invariably found that the prepared VOPO4⋅2H2O material showed low output in production and unsatisfactory performance in zinc storage property, which is in a difficult position to meet the demand of large-scale EES application. For instance, Shi et al. studied the zinc storage performance of the VOPO4⋅2H2O cathode synthesized by a reflux method. And it is shown that the VOPO4⋅2H2O cathode only released 60 mAh g–1 at a high electric current density of 5 A g–1 and an unobtrusive cycle-life with only 500 cycles at 2 A g–1 (Shi et al., 2019). Consequently, it is essential to produce a high performance VOPO4⋅2H2O cathode material with large-scale production.
Herein, a facile route has been designed for the large-scale synthesis of VOPO4⋅2H2O sample by a simple solid-state method, which is in great favor of extending the application of aqueous ZIBs in large-scale storage devices. The VOPO4⋅2H2O electrode delivers an excellent zinc storage ability with high reversible discharge specific capacity (165 mAh g–1 at 0.05 A g–1) and good rate characteristics (90 and 75 mAh g–1 at 2 and 5 A g–1, respectively) in the voltage window of 0.4–1.6 V (versus Zn2+/Zn). Moreover, the VOPO4⋅2H2O electrode exhibits a superior long cycle performance with stable Coulombic efficiency evolution during the repetitive electrochemical process (i.e., 76% capacity retention up to the 1000th cycle at a high electric current density of 5 A g–1).
Materials and Methods
Synthesis of VOPO4⋅2H2O Sample
In a typical procedure, a suspension consisting of 1440 mL deionized water (DI H2O), 60 g vanadium pentoxide (V2O5) powders, and 360 mL concentrated phosphoric acid (85% H3PO4) was transferred to an agate tank and ball-milled at an appropriate speed of 500 rmp min–1 for 30 h. After the program completed, the yellow-greenish products were centrifuged and washed by water and acetone several times. Conclusively, the resulting product was dried in a vacuum (60°C for 8 h) to yield VOPO4⋅2H2O sample. Here, it is noteworthy that the total mass of the as-obtained VOPO4⋅2H2O power prepared by this simple method can reach to a mass production of ∼80 g, which is nearly 100 times of the yield via the traditional reflux method (Shi et al., 2019).
Characterization
The polycrystall X-ray diffraction (XRD, Rigaku SmartLab) with Cu Kα X-ray source (λ = 0.154056 nm) was employed to accurately determine the crystallographic structure of the prepared sample. The fourier transform infrared spectroscope (FTIR, Nicolet 6700) was detected within a wavenumber interval of 4000–500 cm–1. The TG curve was obtained by a thermal gravimetric analysis (TGA/DSC3 +) instrument from 25 to 600°C in air atmosphere (heating rate: 10°C min–1). The X-ray photoelectron spectroscopy (XPS, Escalab 250Xi) was used to analysis phase composition and chemical state of element for the final product. The field-emission scanning electron microscopy (FESEM, Hitach SU-8220) and transmission electron microscopy (TEM, JEM-2100F) instrument were used to measure the morphology for the synthesized sample.
Electrochemical Measurement
The working electrode (i.e., cathode) contained 60 wt% active material VOPO4⋅2H2O, 30 wt% conductive carbon nanotubes and 10 wt% binder polyvinylidene fluoride in N-methyl pyrrolidone solvent. After thorough stirring, the homogenous slurry was coated on titanium foils and dried in a vacuum oven at 60°C for a whole night. The CR2032-type coin cells were used to assemble button cells, including the above working cathode, the metallic zinc anode and 3 M Zn (CF3SO3)2 electrolyte as well as glass-fiber separator in air atmosphere. Galvanostatic charge–discharge cycling and rate performance tests were investigated within the voltage test window of 0.4–1.6 V (vs Zn2+/Zn) by using NEWARE system. Electrochemical impedance spectroscopy (EIS) studies were executed with the button cell on an electrochemical workstation (Multi-Autolab M204) within the frequency domain of 105–10–2 Hz. Cyclic voltammetry (CV) were also tested by the Multi-Autolab M204 measurement at different potential scan rates scanning from 0.1 to 0.5 mV s–1. It is mentioned here that, before electrochemical testing, the VOPO4⋅2H2O cathode was initially activated by discharging to 0.4 V.
Results and Discussion
The crystallographic structure and phase purity of the resultant product is measured by XRD technique. As shown in Figure 1A, all observed reflections completely matched with the standard pattern of the tetragonal VOPO4⋅2H2O (space group: P4/n, JCPDS card: 84-0111). And no detectable impurity peaks can be found, indicating the high purity of VOPO4⋅2H2O crystal (Zhou et al., 2016). The FT-IR analysis is carried out to confirm the existence of various groups in the VOPO4⋅2H2O sample, and its spectrum is exhibited in Figure 1B. The absorption peaks located at about 684 cm–1 is attributed to the V-O extension vibration. The peaks at 950 and 1080 cm–1 can be related to the P-O stretching vibration in PO4 group (Bao et al., 2011). The absorption peaks of P–OH and O–H stretching vibration in H2O are observed at 3600 and 3400 cm–1, respectively. The peak at 1616 cm–1 corresponds to the H–O–H bending vibration of interlayer H2O (Zhou et al., 2014). These characteristic peaks are proved to be in agreement with the chemical composition of VOPO4⋅2H2O (Patel et al., 2003). Additionally, the water content of the final product is confirmed by the TG analysis in the air test environment within the operating temperature window of 25–600°C. As shown in the illustration of Figure 1B, the weight loss percentage of the final product between 25 and 150°C is calculated to be 18.5%, which conforming with the evaporation loss of two crystal water molecules in per mole of as-obtained sample (Zhou et al., 2018; Hyoung et al., 2019). The surface element analysis and chemical states for as-prepared composite were further identified via XPS spectra. As demonstrated in Figure 1C, P, O, and V elements were presented in VOPO4⋅2H2O survey spectrum, and the high-resolution XPS spectra of P2p and O1s are displayed in Supplementary Figure 1 (Supplementary Material), which are in good accordance with the reported result in the recent literature (Verma et al., 2019). According to the high-resolution V2p XPS spectrum in Figure 1D, it is clearly observed two sharp peaks located at the binding energy of 518.5 and 516.6 eV, according with V2p3/2 of V5+ and V4+, respectively. And the other peaks at 525.8 and 524.6 eV are associated with V2p1/2 of V5+ and V4+, respectively (Chen et al., 2018).
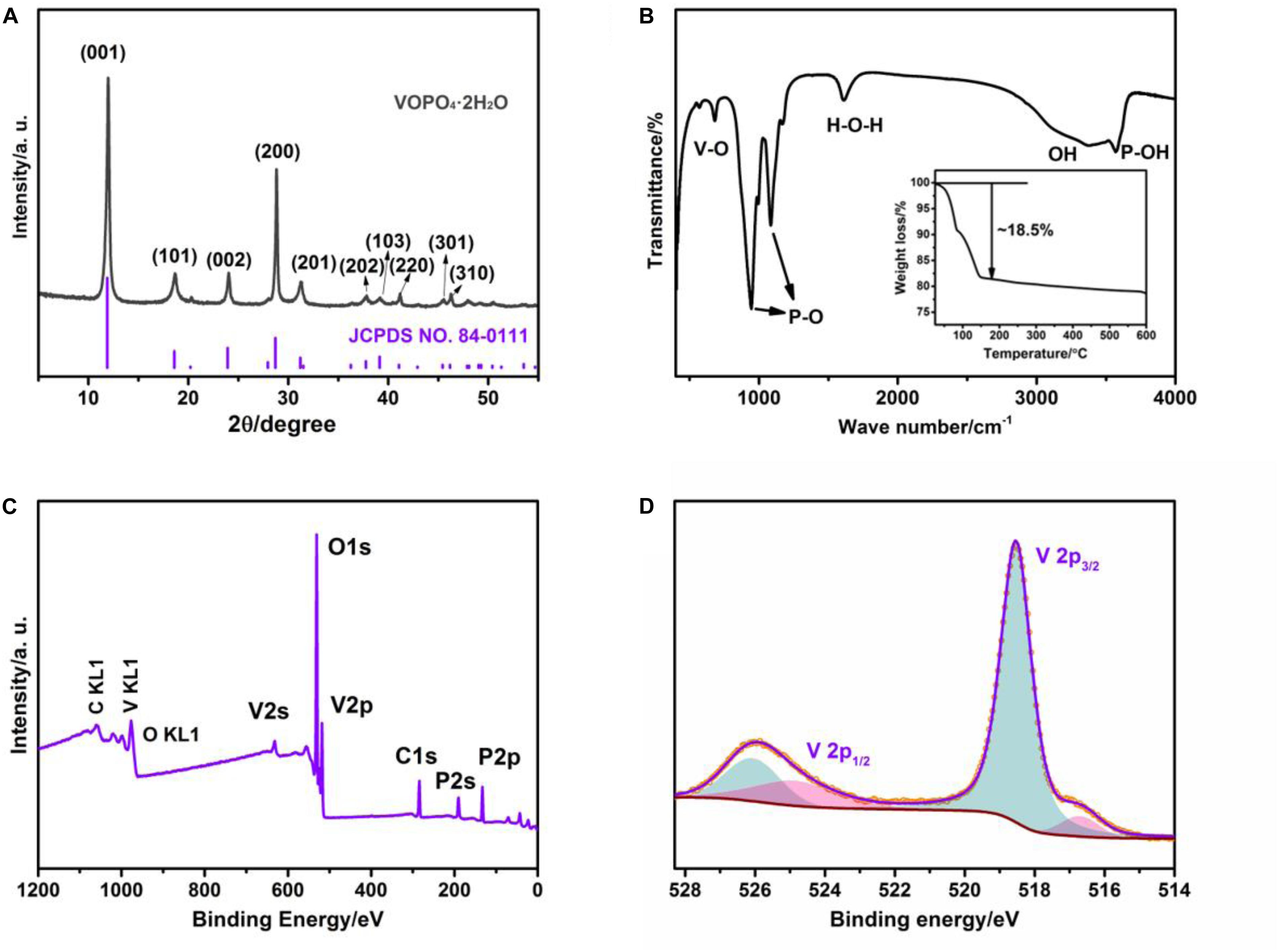
Figure 1. The chemical and physical characterization of VOPO4⋅2H2O sample. (A) XRD pattern; (B) FT-IR spectrum and TG curve (inset); (C) the survey XPS spectrum; (D) XPS high-resolution spectrum of V 2p.
The morphology and detailed structure of VOPO4⋅2H2O sample are investigated by FESEM and TEM analysis. The VOPO4⋅2H2O sample displays irregular bulk morphology with the size of 0.5- 2 μm from the SEM images (Figures 2a,b). The TEM images in Figures 2c,d indicate that this bulk material contains many nanosheets. Moreover, a lattice fringe with the d-spacing of ≈0.36 nm can be also investigated in the HRTEM image (Figure 2e), corresponding to the (0 0 2) crystal plane of the tetragonal VOPO4⋅2H2O.
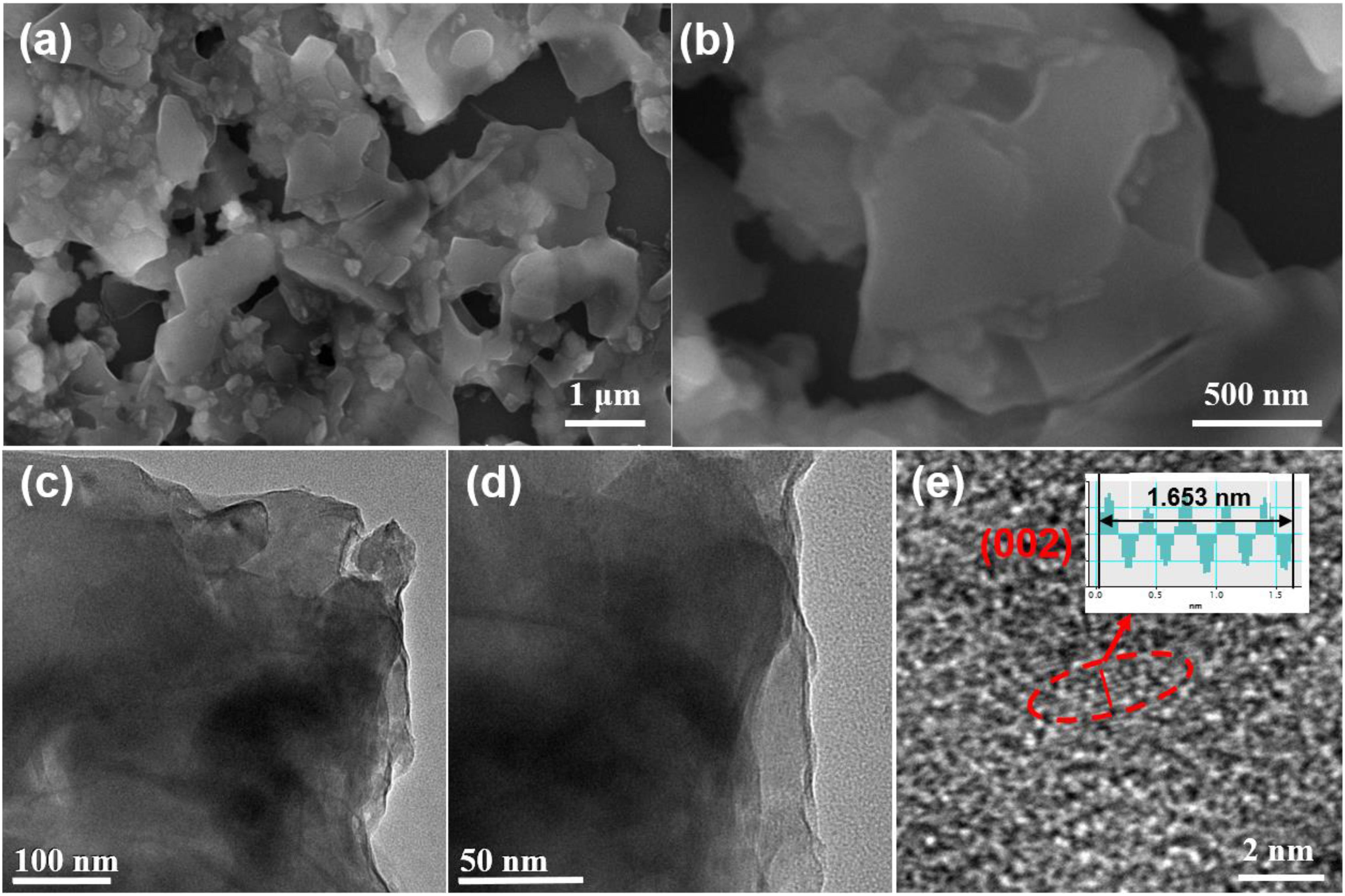
Figure 2. The microstructure and morphology of the VOPO4⋅2H2O sample. (a,b) SEM images under different scanning resolutions; (c,d) TEM images; (e) HRTEM image.
In order to systematically investigate the zinc storage performance of VOPO4⋅2H2O cathode in large-scale EES applications, a series of electrochemical tests based on coin cells employing 3 M Zn(CF3SO3)2 aqueous electrolyte are carried out within the voltage window of 0.4–1.6 V (versus Zn2+/Zn). The typical galvanostatic charge-discharge profiles from the 1st to 50th cycles are revealed in Figure 3A. Two stable flat charge voltages at 0.7 and 1 V appeared under the driven forward by zinc ions over cycles (Shi et al., 2019). The associated differential capacity curves are also displayed in Supplementary Figure 2, Supporting information. And two apparent redox peaks of 0.58/0.76 and 0.92/1.05 V are associated with the two-step Zn2+ (de)intercalation processes. Moreover, the representative cyclic voltammetry test of the VOPO4⋅2H2O electrode at 0.1 mV s–1 scan rate in the voltage range of 0.4–1.6 V (vs Zn2+/Zn) is presented in Supplementary Figure 3, Supporting information. The two apparent redox peaks are clearly observed in the CV curves, which are almost identical to the result obtained from the associated differential capacity curves. Figure 3B exhibits the capacity and coulombic efficiency of VOPO4⋅2H2O cathode at 0.05 A g–1 in the voltage range of 0.4–1.6 V versus Zn2+/Zn. Obviously, the VOPO4⋅2H2O cathode exhibited a disappointing cycling stability at the low electric current density of 0.05 A g–1, this is mainly due to the presence or co-intercalation of water in the active material and the side reaction of the electrolyte caused during the longer Zn2+ insertion process at the low current density, the zinc storage capacity of VOPO4⋅2H2O electrode is on the decline in the previous activation stages, leading to the discharge specific capacity of the VOPO4⋅2H2O cathode decayed from 165 to 108 mAh g–1 over 50 cycles.
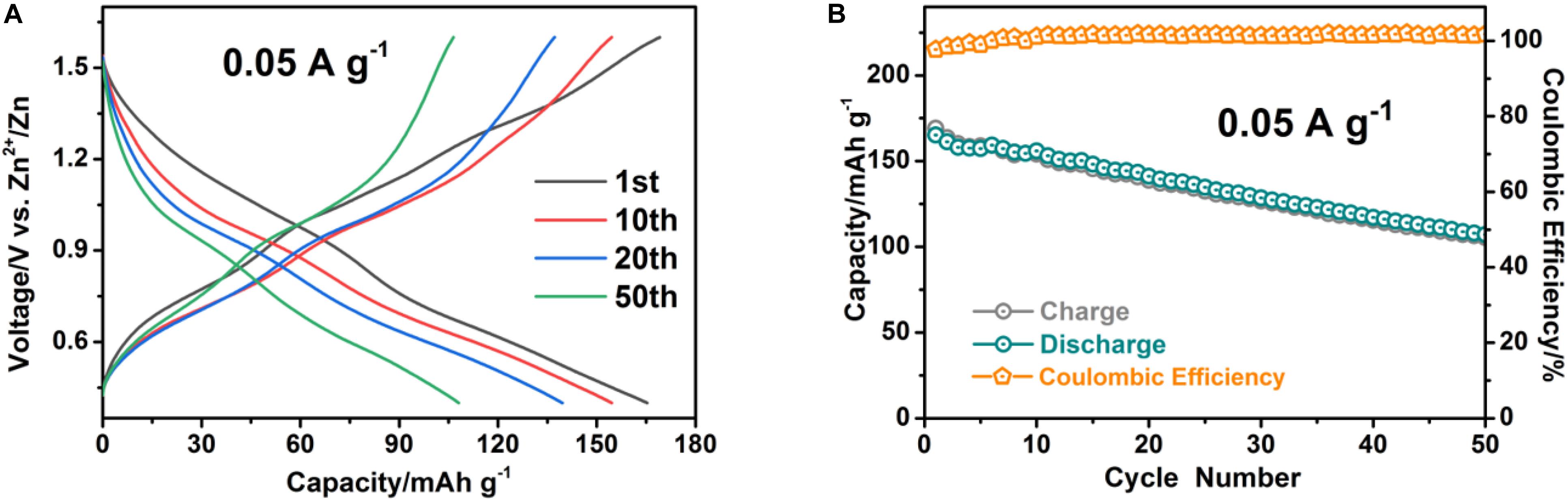
Figure 3. The electrochemical performance of the VOPO4⋅2H2O sample at low current density of 0.05 A g−1. (A) Reversible charge-discharge profiles; (B) capacity and coulombic efficiency evolution.
Additionally, the rate performance and its associated voltage profiles of the VOPO4⋅2H2O electrode under various current densities are exhibited in Figures 4A,B. The VOPO4⋅2H2O electrode displays high reversible specific capacities of 165, 114, 108, 103, 97, and 90 mAh g–1 (according to the discharge specific capacities at 1st, 6th, 11th, 16th, 21th, and 26th cycles) at 0.05, 0.1, 0.2, 0.5, 1, and 2 A g–1, respectively. Even at a high electric current density of 5 A g–1, the VOPO4⋅2H2O cathode can still achieve an attractive reversible capacity of 75 mAh g–1. And a reversible specific capacity of 125 mAh g–1 remains when the electric current density is changed back to 0.05 A g–1, manifesting the reversible redox kinetic of VOPO4⋅2H2O electrode. Furthermore, the cycling behavior of the VOPO4⋅2H2O electrode has been examined at the high electric current density of 5 A g–1 displayed in Figure 4C and Supplementary Figure 4, Supporting information. The VOPO4⋅2H2O electrode not only holds the general shape of the galvanostatic charge-discharge profiles during the long term cycle (1000 times) at 5 A g–1, but also retains a stable reversible specific capacity of 57 mAh g–1 with 76% of capacity retention after 1000 cycles, which is exceptional to that of recently covered VOPO4⋅2H2O based aqueous ZIBs (Shi et al., 2019).
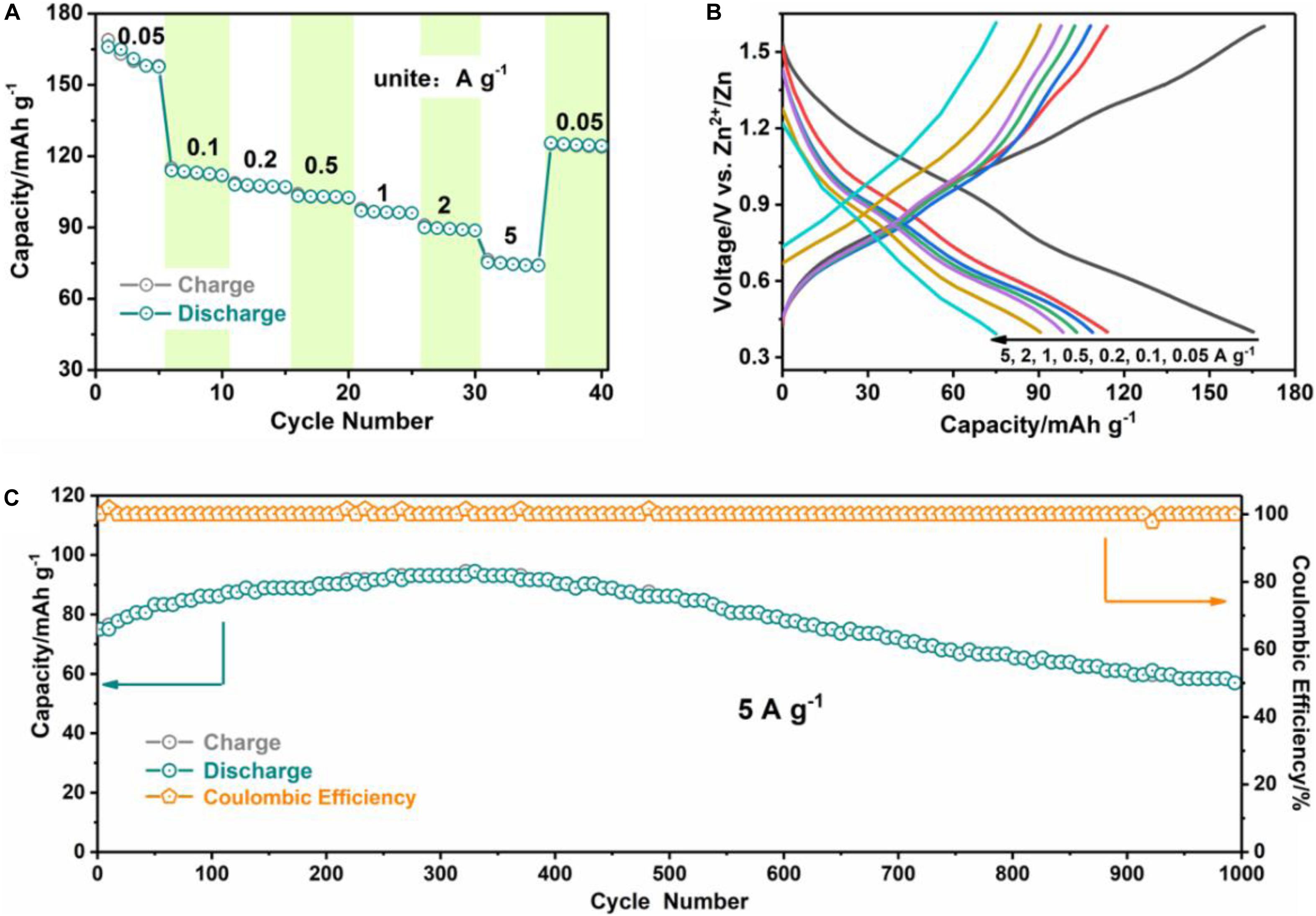
Figure 4. The electrochemical characteristics of VOPO4⋅2H2O sample. (A) Rate capabilities at different current densities from 0.05 to 5 A g–1; (B) typical galvanostatic charge-discharge curves; (C) the long-term cycling performance at high current density of 5 A g–1 (the cell is initially activated for five cycles at 0.05 A g–1).
Furthermore, EIS analysis is also measured to explore the electrochemical kinetics of VOPO4⋅2H2O cathode for zinc storage behavior. As shown in Figure 5A, the Nyquist plot of the VOPO4⋅2H2O electrode is evaluated at the 3rd fully charged state. It includes the semicircle part of high-middle frequency range and the slant part of low frequency, which represent charge transfer impedance (Rct) between the VOPO4⋅2H2O electrode and electrolyte and Warburg resistance (W) corresponded to the zinc ion diffusion in active material, respectively. Equivalent circuit model is established by EIS analysis and shown in the inset of Figure 5A. It has been calculated that the Rct-value of VOPO4⋅2H2O electrode is simulated to be around 184 Ω, smaller than that of recent reported VOPO4⋅2H2O electrodes in aqueous ZIBs (Shi et al., 2019). It is remarkable that the smaller Rct-value indicates the more relax desolvation at the interphase and faster zinc ions diffusion. Here, the apparent diffusion coefficient of Zn2+ (DZn^2+) for the VOPO4⋅2H2O electrode is explored and calculated by applying the Equation: (Yang et al., 2019). In the formula, R, T, and F stand for the universal gas constant, absolute temperature and the Faraday constant, corresponding to the related values of 8.314 J mol–1 K–1, 298.15 K, and 96,485 C mol–1, respectively. S, n, and C are delegated the active surface area of the cathode, the number of electrons migration and the concentration of zinc ions, respectively. The Warburg coefficient σ is related to the gradient of the fitting linear of Z′-ω–1/2 based on the standard equation: Z′ = R + σω−1/2 (Chen S. et al., 2019). Where ω astricts the low frequency area, R is a frequency independent kinetic parameter, whose value is approximately equal to that of Rct in the Nyquist plot. Figure 5B displays the linear fitting of Z′-ω–1/2 and shows a faster diffusion process (3.2 × 10–14 cm2 s–1) of zinc ions during the repeated charge/discharge process. Overall, such small charge transfer impedance and large apparent diffusion coefficient are favorable to promote the (de)intercalation of zinc ions, exhibiting outstanding large current performance and excellent rate capability. The cyclic voltammetry (CV) technique was performed to further understand the zinc storage performance of the as-obtained VOPO4⋅2H2O cathode. The CV profiles at different potential scanning rates ranging from 0.1 to 0.5 mV s–1 are revealed in Figure 5C, two well-defined sharp redox reaction peaks are observed, corresponding to the two-step Zn2+ (de)intercalation behavior, which also are well consisted with the voltage platforms appeared in galvanostatic charge-discharge curves. What’s more, the sharp defined redox peaks could still be kept at the high scanning rate of 0.5 mV s–1, although the redox peaks increased in width and height with the increment of the scan rate, suggesting the good reaction kinetics and excellent rate performance of VOPO4⋅2H2O cathode. Furthermore, the zinc ion diffusion coefficient can be also obtained base on the data from the CV profiles and applying of the Randles-Sevcik equation (Wei et al., 2017):
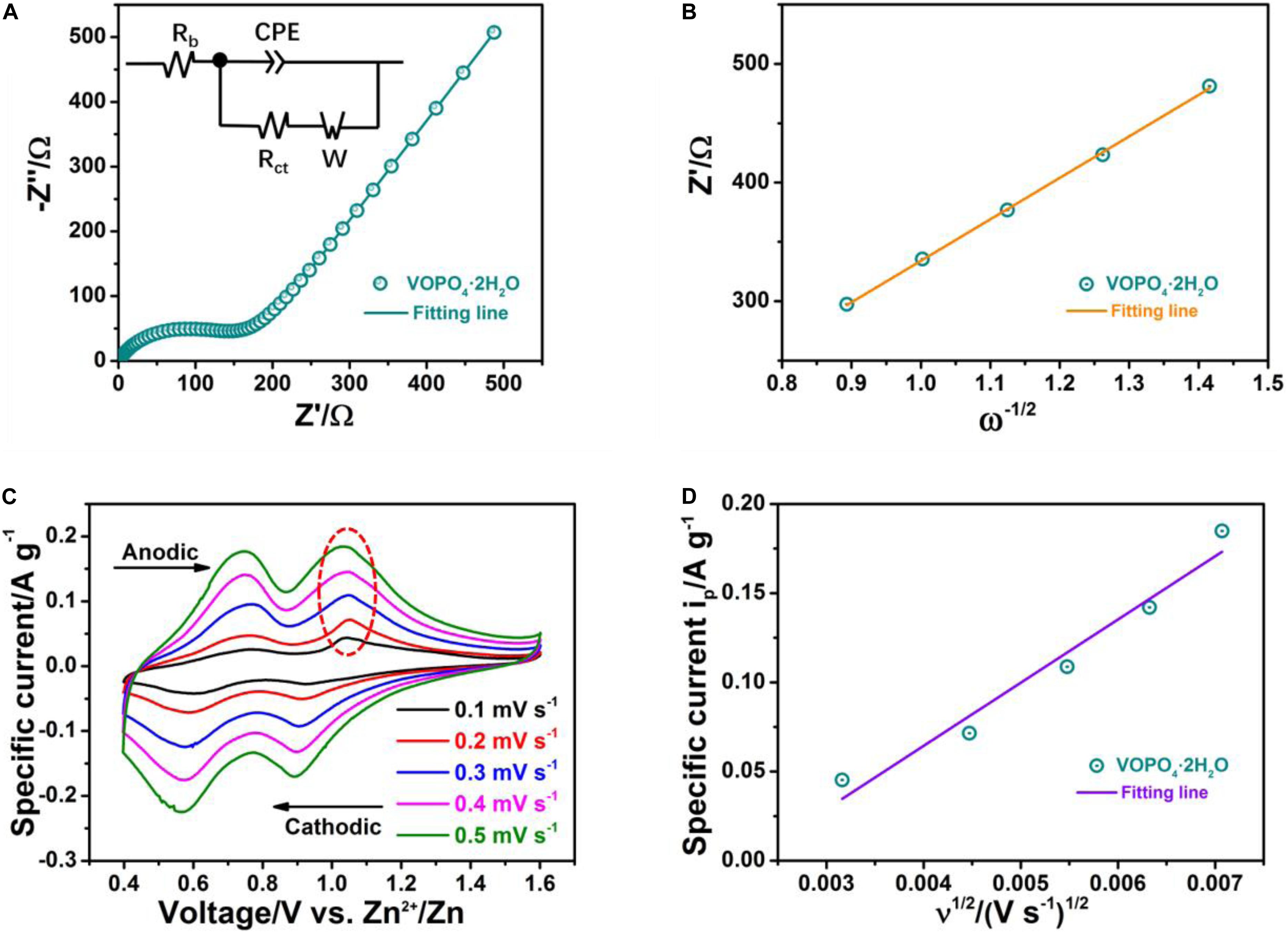
Figure 5. The kinetics behavior of the VOPO4⋅2H2O sample. (A) Nyquist plot; (B) fitting line of Z′−ω–1/2; (C) CV curves at various scan rates; (D) the relationship of the specific current of the oxidation peak marked by a circle in panel (C) and the square root of scan rate.
wherein ip is the specific peak current, n stands for the number of electrons migration, S is the actual contact area between the cathode active material and electrolyte, C is referred to the concentration of Zn2+, and υ is scanning rate. is estimated from the slope of fitting linear of the specific current ip along with the square root of the scan rate υ1/2. Based on the slope value of ip-υ1/2 in Figure 5D, the zinc ion diffusion coefficient () of VOPO4⋅2H2O cathode is calculated to be ∼2.0 × 10–13 cm2 s–1, according well with the value of Zn2+ coefficient obtained from EIS measurement. And the fast charge transfer kinetics of Zn2+ (de)intercalation for the VOPO4⋅2H2O cathode is further highlighted via the applying of CV technique.
Conclusion
In this study, we have developed a facial solid state reaction to prepare the VOPO4⋅2H2O sample with industrial mass production grade. When the VOPO4⋅2H2O is applied as cathode material of rechargeable aqueous ZIBs, it displays high reversible specific capacity (165 mAh g–1 at 0.05 A g–1 within the voltage window of 0.4–1.6 V (vs. Zn2+/Zn)) and superior rate capability (90 and 75 mAh g–1 at 2 and 5 A g–1, respectively), as well as outstanding stable cyclicity (76% of capacity retention after 1000 cycles at 5 A g–1). In addition to the excellent zinc storage performance, the VOPO4⋅2H2O cathode can easily achieve mass production based on simple effective regulation and control in synthesis, showing great development potential in the field of large-scale EES.
Data Availability Statement
The raw data supporting the conclusions of this article will be made available by the authors, without undue reservation.
Author Contributions
XR directed the project. XZ, DY, and WL performed the experiment, analyzed data, and wrote the manuscript. All authors contributed to the discussion.
Funding
The authors gratefully acknowledge the National Natural Science Foundation of China (Grant Nos. 51972067 and 51802044) and Guangdong Natural Science Funds for Distinguished Young Scholar (Grant No. 2019B151502039).
Conflict of Interest
The authors declare that the research was conducted in the absence of any commercial or financial relationships that could be construed as a potential conflict of interest.
Supplementary Material
The Supplementary Material for this article can be found online at: https://www.frontiersin.org/articles/10.3389/fenrg.2020.00211/full#supplementary-material
References
Ao, H., Zhao, Y., Zhou, J., Cai, W., Zhang, X., Zhu, Y., et al. (2019). rechargeable aqueous hybrid ion batteries: developments and prospects. J. Mater. Chem. A 7, 18708–18734. doi: 10.1039/C9TA06433H
Bao, C., Guo, Y., Song, L., Lu, H., Yuan, B., and Hu, Y. (2011). Facile Synthesis of Poly(vinyl alcohol)/alpha-Titanium phosphate nanocomposite with markedly enhanced properties. Ind. Eng. Chem. Res. 50, 11109–11116. doi: 10.1021/ie200700t
Chen, D., Rui, X., Zhang, Q., Geng, H., Gan, L., Zhang, W., et al. (2019). Persistent Zinc-ion storage in mass-produced V2O5 architecture. Nano Energy 60, 171–178. doi: 10.1016/j.nanoen.2019.03.034
Chen, L., Chen, L., Zhai, W., Li, D., Lin, Y., Guo, S., et al. (2019). Tunable synthesis of LixMnO2 nanowires for aqueous li-ion hybrid supercapacitor with high rate capability and ultra-long cycle life. J. Power Sources 413, 302–309. doi: 10.1016/j.jpowsour.2018.12.026
Chen, S., Zhang, Y., Geng, H., Yang, Y., Rui, X., and Li, C. (2019). Zinc ions pillared vanadate cathodes by chemical pre-intercalation towards long cycling life and low-temperature zinc ion batteries. J. Power Sources 441, 227192. doi: 10.1016/j.jpowsour.2019.227192
Chen, N., Zhou, J., Zhu, G., Kang, Q., Ji, H., Zhang, Y., et al. (2018). A High-performance asymmetric supercapacitor based on vanadyl phosphate/carbon nanocomposites and polypyrrole-derived carbon nanowires. Nanoscale 10, 3709–3719. doi: 10.1039/C7NR08909K
Deng, C., Xie, X., Han, J., Tang, Y., Gao, J., Liu, C., et al. (2020). A sieve-functional and uniform-porous kaolin layer toward stable zinc metal anode. Adv. Funct. Mater. 30:2000599. doi: 10.1002/adfm.202000599
Guo, Q., Li, S., Liu, X., Lu, H., Chang, X., Zhang, H., et al. (2020). Ultrastable sodium-sulfur batteries without polysulfides formation using slit ultramicropore carbon carrier. Adv. Sci. 7:1903246. doi: 10.1002/advs.201903246
Guo, X., Zhou, J., Bai, C., Li, X., Fang, G., and Liang, S. (2020). Zn/MnO2 battery chemistry with dissolution-deposition mechanism. Mater. Today Energy 16:100396. doi: 10.1016/j.mtener.2020.100396
Huang, H.-L., Lii, K.-H., and Wang, S.-L. (2014). Really understanding layered vanadyl phosphate hydrates. J. Chin. Chem. Soc. 61, 199–206. doi: 10.1002/jccs.201300520
Hyoung, J., Heo, J. W., Chae, M. S., and Hong, S.-T. (2019). Electrochemical exchange reaction mechanism and the role of additive water to stabilize the structure of VOPO4 center dot 2H2O as a cathode material for potassium-ion batteries. Chemsuschem 12, 1069–1075. doi: 10.1002/cssc.201802527
Ke, L., Song, B., Zhang, Y., Ma, H., and Zhang, J. (2017). Encapsulation of zinc hexacyanoferrate nanocubes with manganese oxide nanosheets for high-performance rechargeable zinc-ion batteries. J. Mater. Chem. A 5, 23628–23633. doi: 10.1039/C7TA07834J
Li, C., Wang, B., Chen, D., Gan, L., Feng, Y., Zhang, Y., et al. (2020). Topotactic transformation synthesis of 2D Ultrathin GeS2 nanosheets toward high-rate and high-energy-density sodium-Ion Half/Full Batteries. ACS Nano 14, 531–540. doi: 10.1021/acsnano.9b06855
Li, G., Chen, W., Zhang, H., Gong, Y., Shi, F., Wang, J., et al. (2020). Membrane-Free Zn/MnO2 flow battery for large-scale energy storage. Adv. Energy Mater. 10:1902085. doi: 10.1002/aenm.201902085
Li, X., Tang, Y., Lv, H., Wang, W., Mo, F., Liang, G., et al. (2019). Recent advances in flexible aqueous zinc-based rechargeable batteries. Nanoscale 11, 17992–18008. doi: 10.1039/C9NR06721C
Lin, B., Zhu, X., Fang, L., Liu, X., Li, S., Zhai, T., et al. (2019). Birnessite Nanosheet Arrays with High K Content as a High-Capacity and Ultrastable Cathode for K-Ion Batteries. Adv. Mater. 31:e1900060. doi: 10.1002/adma.201900060
Liu, S., Zhu, H., Zhang, B., Li, G., Zhu, H., Ren, Y., et al. (2020). Tuning the kinetics of zinc-ion insertion/extraction in V2O5 by in situ polyaniline intercalation enables improved aqueous zinc-ion storage performance. Adv. Mater. 32:2001113. doi: 10.1002/adma.202001113
Palanisamy, K., Um, J. H., Jeong, M., and Yoon, W.-S. (2016). Porous V2O5/RGO/CNT hierarchical architecture as a cathode material: emphasis on the contribution of surface lithium storage. Sci. Rep. U. K. 6:31275. doi: 10.1038/srep31275
Patel, V., Chudasam, A., Nivsarkar, M., Vasu, K., and Shishoo, C. (2003). Synthesis and Characterization of an VOPO4–polyaniline lamellar hybrid compound. Solid State Sci. 5, 611–613. doi: 10.1016/S1293-2558(03)00046-3
Peng, L., Zhu, Y., Peng, X., Fang, Z., Chu, W., Wang, Y., et al. (2017). effective interlayer engineering of two-dimensional VOPO4 nanosheets via controlled organic intercalation for improving alkali ion storage. Nano Lett. 17, 6273–6279. doi: 10.1021/acs.nanolett.7b02958
Shi, H., Song, Y., Qin, Z., Li, C., and Sun, X. (2019). Inhibiting VOPO4⋅2H2O decomposition and dissolution in rechargeable aqueous zinc batteries to promote voltage and capacity stabilities. Angew. Chem. 58, 16057–16061. doi: 10.1002/anie.201908853
Song, M., Tan, H., Chao, D., and Fan, H. J. (2018). Recent advances in Zn-Ion batteries. Adv. Funct. Mater. 28:1802564. doi: 10.1002/adfm.201802564
Sun, W., Wang, F., Hou, S., Yang, C., Fan, X., Ma, Z., et al. (2017). Zn/MnO2 battery chemistry With H+ and Zn2 + coinsertion. J. Am. Chem. Soc. 139, 9775–9778. doi: 10.1021/jacs.7b04471
Tang, Y., Liu, C., Zhu, H., Xie, X., Gao, J., Deng, C., et al. (2020). Ion-confinement effect enabled by gel electrolyte for highly reversible dendrite-free zinc metal anode. Energy Storage Mater. 27, 109–116. doi: 10.1016/j.ensm.2020.01.023
Verma, V., Kumar, S., Manalastas, W. Jr., Zhao, J., Chua, R., Meng, S., et al. (2019). Layered VOPO4 as a cathode material for rechargeable zinc-ion battery: effect of polypyrrole intercalation in the host and water concentration in the electrolyte. ACS Appl. Energy Mater. 2, 8667–8674. doi: 10.1021/acsaem.9b01632
Wang, B., Ang, E. H., Yang, Y., Zhang, Y., Geng, H., Ye, M., et al. (2020). Interlayer engineering of molybdenum trioxide toward high-capacity and stable sodium ion Half/Full batteries. Adv. Funct. Mater. 30:2001708. doi: 10.1002/adfm.202001708
Wei, T., Yang, G., and Wang, C. (2017). Bottom–up assembly of strongly–coupled Na3V2(PO4)3/C into hierarchically porous hollow nanospheres for high–rate and –stable Na–ion Storage. Nano Energy 39, 363–370. doi: 10.1016/j.nanoen.2017.07.019
Xia, C., Guo, J., Lei, Y., Liang, H., and Alshareef, H. N. (2017). Rechargeable aqueous zinc-ion battery based on porous framework zinc pyrovanadate intercalation cathode. Adv. Mater. 30:1705580. doi: 10.1002/adma.201705580
Xia, H., Zhu, X., Liu, J., Liu, Q., Lan, S., Zhang, Q., et al. (2018). A monoclinic polymorph of sodium birnessite for ultrafast and ultrastable sodium ion storage. Nat. Commun. 9:5100. doi: 10.1038/s41467-018-07595-y
Xie, X., Liang, S., Gao, J., Guo, S., Guo, J., Wang, C., et al. (2020). Manipulating the ion-transfer kinetics and interface stability for high-performance zinc metal anodes. Energy. Environ. Sci. 13, 503–510. doi: 10.1039/c9ee03545a
Xiong, P., Zhang, F., Zhang, X., Wang, S., Liu, H., Sun, B., et al. (2020). Strain engineering of two-dimensional multilayered heterostructures for beyond-lithium-based rechargeable batteries. Nat. Comm. 11, 3297–3297. doi: 10.1038/s41467-020-17014-w
Yang, L., Liao, H., Tian, Y., Hong, W., and Ji, X. (2019). Rod-Like Sb2MoO6: structure evolution and sodium storage for sodium-ion batteries. Small Methods 3:1800533. doi: 10.1002/smtd.201800533
Yang, Y., Zhu, H., Xiao, J., Geng, H., Zhang, Y., Zhao, J., et al. (2020). Achieving ultrahigh-rate and high-safety Li+ storage based on interconnected tunnel structure in micro-size niobium tungsten oxides. Adv. Mater. 32:1905295. doi: 10.1002/adma.201905295
Yue, Z., Peng, L., Chen, D., and Yu, G. (2016). Intercalation pseudocapacitance in ultrathin VOPO4 nanosheets: toward high-rate alkali-ion-based electrochemical energy storage. Nano Lett. 16, 742–747. doi: 10.1021/acs.nanolett.5b04610
Zhang, S., Yang, D., Tan, H., Feng, Y., Rui, X., and Yu, Y. (2020). Advances in K-Q (Q = S, Se and SexSy) batteries, mater. Today
Zhang, X., Chen, H., Liu, W., Xiao, N., Zhang, Q., Rui, X., et al. (2020). A long-cycling aqueous zinc-ion pouch cell: NASICON-Type material and surface modification. Chem. Asian J. 15, 1430–1435. doi: 10.1002/asia.202000162
Zhou, K., Zhang, Q., Wang, B., Liu, J., Wen, P., Gui, Z., et al. (2014). The integrated utilization of typical clays in removal of organic dyes and polymer nanocomposites. J. Clean. Prod. 81, 281–289. doi: 10.1016/j.jclepro.2014.06.038
Zhou, K., Zhou, G., and Yuan, H. (2016). Ultrathin 2D VOPO4 nanosheets: a novel reinforcing agent in polymeric composites. RSC Adv. 6, 100344–100351. doi: 10.1039/C6RA24111E
Keywords: zinc ion battery, cathode material, VOPO4⋅2H2O, large-scale synthesis, long cycling performance
Citation: Zhang X, Yang D, Liu W and Rui X (2020) VOPO4⋅2H2O: Large-Scale Synthesis and Zinc-Ion Storage Application. Front. Energy Res. 8:211. doi: 10.3389/fenrg.2020.00211
Received: 28 June 2020; Accepted: 05 August 2020;
Published: 04 September 2020.
Edited by:
Cheng Zhong, Tianjin University, ChinaReviewed by:
Lina Chen, Harbin Institute of Technology, Shenzhen, ChinaHui Xia, Nanjing University of Science and Technology, China
Copyright © 2020 Zhang, Yang, Liu and Rui. This is an open-access article distributed under the terms of the Creative Commons Attribution License (CC BY). The use, distribution or reproduction in other forums is permitted, provided the original author(s) and the copyright owner(s) are credited and that the original publication in this journal is cited, in accordance with accepted academic practice. No use, distribution or reproduction is permitted which does not comply with these terms.
*Correspondence: Xianhong Rui, eGhydWlAZ2R1dC5lZHUuY24=