- 1Dipartimento di Ingegneria Chimica, dei Materiali e della Produzione Industriale, Università di Napoli Federico II, Naples, Italy
- 2Istituto di Ricerche sulla Combustione – CNR, Naples, Italy
This study investigates the catalytic properties of K+ and Cu2 + /Fe3 + co-doped ceria-zirconia (CeZr) toward water and carbon dioxide co-splitting. These materials can convert separate feeds of CO2 and H2O into CO and H2. In co-splitting tests, water reacts faster on the K-Cu-CeZr catalyst with negligible CO production. The reduction of the K-Fe-CeZr catalyst occurs over two broad temperature ranges: at low temperature, only H2 is produced; whereas CO is the most abundant product at high temperature. A kinetic model was developed to get insights into the reasons of the observed selectivity toward H2 at low temperature and CO at a higher temperature. The different reaction orders in the sites fraction were evaluated for CO2 and H2O reactions, highlighting that H2 production requires a larger number of adjacent reduced sites than CO production. Three regimes were identified through the model: Regime I- H2O driven regime @T ≤ 650°C; Regime II- mixed regime @ 560 < T < 700°C and Regime III: CO2 driven regime @ T > 700°C. These results indicate the appropriate conditions for tuning H2/CO selectivity, depending on the feed composition.
Introduction
The transformation of solar energy into synthetic fuels holds huge promise for sustainable approaches to harvesting renewable energy (Nguyen and Blum, 2015; Chuayboon and Abanades, 2020; Mao et al., 2020). Thermochemical splitting cycles have been proposed as a promising sustainable option, as this approach uses concentrated solar energy to convert H2O and CO2 into H2 and CO (Costa Oliveira et al., 2018; Bhosale et al., 2019; Takalkar et al., 2019). These building blocks can be further reacted into gaseous and liquid fuels.
Among thermochemical cycles, two-step high temperature processes are the most promising (Rao and Dey, 2015), because they are less complex and can achieve a higher and more efficient theoretical solar-to-fuel energy conversion (ηsolar–to–fuel) (Rao and Dey, 2015). The thermochemical splitting catalyst ceria is recognized as an important development (Bhosale et al., 2019) due to its thermal stability, high oxygen storage capacity (OSC), without any structural changes, and the fast kinetics of reduction and splitting reactions (Costa Oliveira et al., 2018; Bhosale et al., 2019; Chen et al., 2019; Takalkar et al., 2019). The thermochemical cycle can be schematically defined as follows:
Reaction 1 corresponds to high temperature self-reduction under concentrated solar power, whereas reactions 2 and 3 are the splitting reactions, which restore the oxidized state of the materials and producing fuels.
Despite the huge CeO2 potential in thermochemical cycles, high reduction temperature (step1), compromising long-term thermal stability over multiple cycles as well as small fuel production still limits its technological application. To date, a number of other studies have addressed these issues (Kaneko et al., 2011; Petkovich et al., 2011; Gokon et al., 2013, 2015a; Agrafiotis et al., 2015; Jarrett et al., 2016; Muhich et al., 2016; Al-Shankiti et al., 2017; Cho et al., 2017; Zhou et al., 2017; Luciani et al., 2019; Bhosale and AlMomani, 2020; Costa Oliveira et al., 2020; Haeussler et al., 2020). Modifying ceria fluorite structure with metal cations has proved to be a successful strategy for improving both oxygen ion mobility and thermal stability, ultimately enhancing the redox activity of the material (Kang et al., 2013; Cooper et al., 2015; Gokon et al., 2015a,b; Takacs et al., 2015; Bhosale et al., 2016; Lin et al., 2016; Zhao et al., 2016; Mostrou et al., 2017; Muhich and Steinfeld, 2017; Bhosale and Takalkar, 2018; Takalkar et al., 2018, 2020a,b; Naghavi et al., 2020).
It is widely accepted that ceria-zirconia solid solutions, in particular those with a Ce/Zr molar ratio ∼ 3, show improved splitting properties, especially in terms of self-reducibility (Abanades et al., 2010; Kaneko et al., 2011; Le Gal and Abanades, 2011; Abanades and Le Gal, 2012; Call et al., 2013; Le Gal et al., 2013; Jacot et al., 2017; Muhich and Steinfeld, 2017). Moreover, the performance of this class of materials can be further enhanced by doping with bi- or trivalent cations, such as Cu2 +, Mn3 +, Fe3 + (Gokon et al., 2015a,b; Lin et al., 2016).
The improved splitting activity of ceria-zirconia solid solutions has been related to the formation of a Zr2ON2 like-phase at the nanoscale level during thermal treatment in N2 atmosphere (Pappacena et al., 2016, 2017), which is associated with a decrease of surface Ce/Zr ratio (Luciani et al., 2019). Good splitting performance has been related also to the formation of both bulk and surface oxygen vacancies, enhancing surface reactivity (oxygen evolution during self-reduction and splitting reactivity during oxidation) and oxygen diffusivity from the bulk to the surface and vice-versa (Luciani et al., 2019).
While a huge amount of literature has been devoted to the thermochemical splitting of bare H2O or CO2, little is known about their simultaneous splitting (Furler et al., 2012; Lorentzou et al., 2014, 2017; Falter and Pitz-Paal, 2018; de la Calle and Bayon, 2019; Tou et al., 2019; Hao et al., 2020). It is worth noting that co-splitting has been generally defined as concurrent or combined splitting, but it should be better defined as competitive splitting; as a matter of fact, CO2 and H2O compete for the same reduced sites, whereas the overall fuel production depends on the reduction extent of the material.
Recently, we proved that potassium cation doping improved Ce0.75Zr0.25O2 splitting performance toward CO2 or H2O (Landi, 2019). Alkali metal ions doping as well as transition metals cations co-doping, such as Cu(II) and Fe(III), provided the formation of both bulk and surface oxygen vacancies, significantly enhancing the splitting performance. Interestingly, K-addition boosted the oxidation kinetics, in agreement with the results obtained in the three step cycles (Charvin et al., 2009). Recently, Takalkar et al. showed a positive effect of Li+ cation on the CO production during CO2 splitting cycles (Takalkar et al., 2020b).
In this work, K-M-doped ceria-zirconia solid solutions (M = Cu2 + and Fe3 +), containing 5% mol/mol of both K and M cations, were tested as catalysts for carbon dioxide and water co-splitting. Furthermore, a kinetic model was developed and used to get insights into the active sites of the catalysts and their activity toward H2O and CO2 splitting, to simulate the splitting performance as well as the composition of produced gases at different oxidation temperatures.
Materials and Methods
Materials Preparation
Reagents were purchased from Sigma-Aldrich and used as received. The material synthesis was carried out by employing the following reagents: cerium (III) nitrate hexahydrate, Ce(NO3)3⋅6H2O; zirconium (IV) oxynitrate hydrate, ZrO(NO3)2⋅xH2O; iron (III) nitrate non ahydrate, Fe(NO3)3⋅9H2O; potassium nitrate, KNO3; copper (II) nitrate tetrahydrate, Cu(NO3)2⋅4H2O; and manganese (II) nitrate tetrahydrate, Mn(NO3)2⋅4H2O. The Ce/Zr molar ratio was kept constant at 3 (Ce0.75Zr0.25O2 as general formula) since it ensured the highest reducible performance among CeO2-ZrO2 solutions (Le Gal and Abanades, 2012).
Bare ceria-zirconia, as well as doped and co-doped materials, were prepared according to the co-precipitation method (Luciani et al., 2018;, Landi, 2019). Stoichiometric amounts of precursor salts were dissolved in 75 ml of bi-distilled water and stirred for 3 h. Then, solutions were heated in an MW oven (CEM SAM-155) until a homogeneous gel was obtained. The calcination in air at 1,100°C for 4 h was then carried out to decompose nitrates and to obtain the oxides. Table 1 summarizes the composition of produced materials as well as the label used.

Table 1. Materials tested for the thermochemical splitting process, including nominal compositions (molar ratios), general formulas, and maximum oxygen evolutions (mmol/g).
H2O/CO2 Splitting and Co-splitting Tests
The experimental equipment and experimental details described above were used to assess the splitting ability of the samples under investigation (Luciani et al., 2018, 2019; Portarapillo et al., 2019). Temperature programmed reduction (TPR) and oxidation (TPO) were carried out on powdered samples (500 mg; 170–300 μm), placed in a tubular quartz reactor, and inserted into an electric tubular furnace (Lenton). The catalyst temperature was measured by a K-type thermocouple placed inside a tube co-axial with the reactor.
As previously reported (Luciani et al., 2018, 2019; Portarapillo et al., 2019), the samples were pre-treated in a hydrogen flow (TPR; 10 l(SLT)/h; 5 vol.% H2/N2) up to approximately 1,000°C, heating rate 10°C/min (Al-Shankiti et al., 2013; Pappacena et al., 2016, 2017) and cooled down to 60°C under a nitrogen flow. TPO was then carried out by flowing (i) a 3 vol.% H2O/N2 mixture (10 l(SLT)/h), (ii) a 6 vol.% CO2/N2 mixture (10 l(SLT)/h), (iii) a (6 vol.% H2O + 6 vol.% CO2)/N2 mixture (10 l(SLT)/h); the temperature raised up to about 1,000°C (heating rate: 10°C/min; time at the maximum temperature: 20 min). We chose to use a diluted mixture according to the method outlined by Petkovich et al. (2011), to avoid a fast reaction, especially on H2-reduced samples. The consistency of this approach has been demonstrated (Luciani et al., 2018, 2019; Portarapillo et al., 2019). The reduction and oxidation profiles were deconvoluted using OriginPro 8.5 software.
Oxygen, hydrogen, and carbon monoxide amounts (mol/g), nO2, nH2, and nCO respectively, were calculated as follows:
where mcat (g) is the sample mass, P (atm) and T (K) are standard pressure and temperature, R (atm⋅l⋅mol−1⋅K–1) is the ideal gas constant, Fred and Fox (l(STP)/s) are the flow rates of permanent gases during H2 treatment and splitting test, respectively, nH2_cons,i and nH2_prod,i are consumed and produced hydrogen moles during the H2 reduction treatment and splitting test, respectively. The areaH2_cons,i, and areaH2_prod,i (%⋅s) are the calculated areas of the reduction and oxidation H2 profiles, respectively. nCO_prod,i is the amount of carbon monoxide produced during the splitting test, and areaCO_prod,i (%⋅s) is the calculated area of the oxidation CO profile. The nO2 does not represent a real molecular oxygen evolution but oxygen subtracted from the materials.
The reduction degree (xred,i) after the current reduction or oxidation step (i) was calculated as follows:
and as in the following for oxidation steps:
where nO2,max is the maximum O2 amount (mol/g) that could be evolved, if reducible cations were reduced to their lowest oxidation state (Ce4+ to Ce3 +, Fe3 + to Fe2 +, Cu2 + to Cu0; corresponding values are reported in Table 1). xred,i–1 is the reduction degree of the previous (oxidation or reduction) step. Therefore, xred,i does not simply quantify the current fraction of Ce3 + ions on the overall content of Ce atoms in the system.
Oxidation yield (αi) is calculated through produced CO (mol/g) during the current re-oxidation step and the corresponding O2 evolved amount (mol/g) during the previous reduction step:
Reduction yield (βi) is calculated through the overall O2 amount (mol/g) produced during the current reduction step and CO evolved amount (mol/g) during the previous oxidation step:
Kinetic Model
A kinetic model was developed to get insights into the role of the superficial reaction on the selectivity in co-feed splitting.
The model is based on the Polanyi-Wigner equation under the Redhead approximation, which states that catalytic sites do not interact. The reactor is modeled as a continuous stirred tank reactor (CSTR).
The reaction mechanism is a redox pathway involving active sites σ: σred as the reduced form and σox as the oxidized form.
According to these hypotheses, the reaction steps are:
Sites fractions are defined by the following equations:
The reaction rates of steps (10–11) are:
where θred is the reduced sites fraction, ni are the reaction orders, Yi are the reactants molar fractions, and ki are the kinetic constants which are evaluated as follows:
where Ei are the activation energies, and are the frequency factors.
The unsteady balance equations on reduced sites fraction for CO2 splitting, H2O splitting, and co-splitting are respectively:
The unsteady balance equations on gaseous molar fractions are:
where τ is the residence time equal to the experimental one.
The initial conditions are:
Differential equations were numerically solved using the Eulero explicit method.
To analyze the fitting quality, the differences between the experimental data and the model were evaluated through the root mean square error (SRMSE), normalized for the maximum value of the curve of the flow test for each step:
Model and experimental curves were considered in good agreements for SRMSE values ≤0.045.
Results
Experimental Results
Previous research has reported on H2/H2O cycling, outlining that it can be used to define the splitting properties of materials (Landi, 2019). Results of thermogravimetric (TG) measurements (reduction under inert Ar atmosphere; oxidation under 40 vol.% CO2/Ar) and H2O splitting tests on H2-reduced samples were successfully compared (Al-Shankiti et al., 2013; Pappacena et al., 2016, 2017; Luciani et al., 2018).
Results by Landi (2019) showed that K-addition to bare ceria-zirconia and materials doped with transition metals (K-Fe-CeZr and K-Cu-CeZr) significantly enhance the evolved oxygen amount and lower the reduction onset temperature. These reduction profiles obtained for the co-splitting reaction are consistent with those reported by other studies (Landi, 2019) and will, therefore, not be further discussed.
Figure 1 (bottom) shows H2 profiles measured in the H2O splitting tests. The profiles refer to the stable performance obtained after the multiple reduction/oxidation cycle (Landi, 2019). One wide peak was detected over the K-Cu-CeZr sample, while two peaks were found over K-Fe-CeZr catalysts.
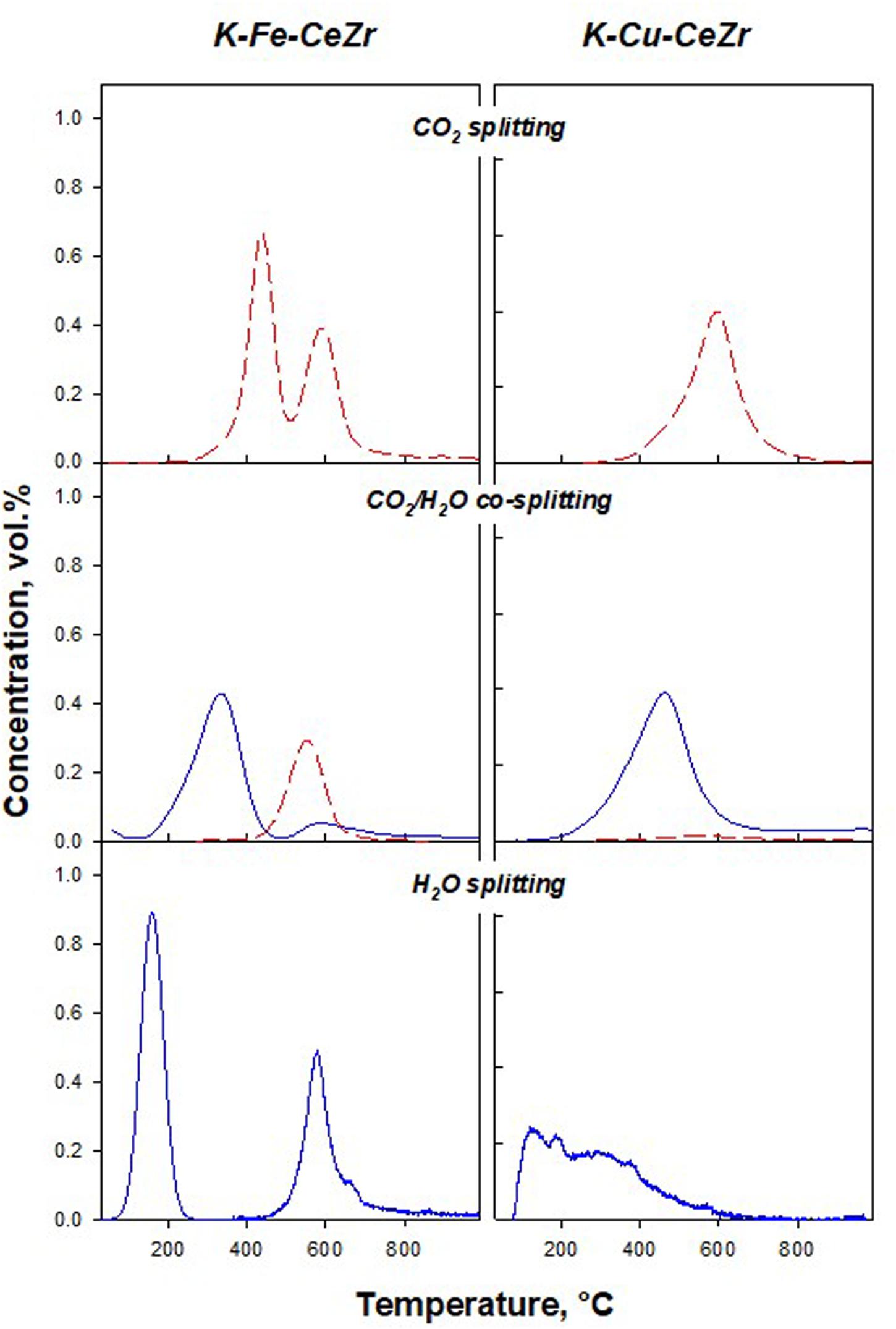
Figure 1. H2 (blue solid lines) and CO (red dashed lines) concentration profiles during splitting tests under different oxidizing streams on K-Fe-CeZr (left) and K-Cu-CeZr (right).
Potassium doped samples show faster oxidation kinetics than K-free materials (Landi, 2019). In particular, H2 production occurs at very low temperature (peak temperature at 160°C for K-Fe-CeZr and 200°C for K-Cu-CeZr sample, respectively). Furthermore, the K-Fe-CeZr sample shows a second oxidation phenomenon at a higher temperature (at about 590°C).
Table 2 reports the amount of produced O2 and H2, reduction degrees after each step (xred), oxidation yields (α), and reduction yields (β) as obtained during H2O splitting tests. Co-doped samples show higher oxygen and hydrogen production than the bare CeZr catalyst, as well as full re-oxidation (approximately 100%). Indeed, K-doping of M-doped ceria-zirconia (M = Fe3 + and Cu2 +) can have limited effects on redox performance with no significant changes in the oxygen released (Fe-CeZr: K-free 847 μmol/g, K-doped 747 μmol/g; Cu-CeZr: K-free 576 μmol/g, K-doped 559 μmol/g) and produced hydrogen (Fe-CeZr: K-free 1,437 μmol/g, K-doped 1,458 μmol/g; Cu-CeZr: K-free 1,070 μmol/g, K-doped 1,237 μmol/g) (Landi, 2019).
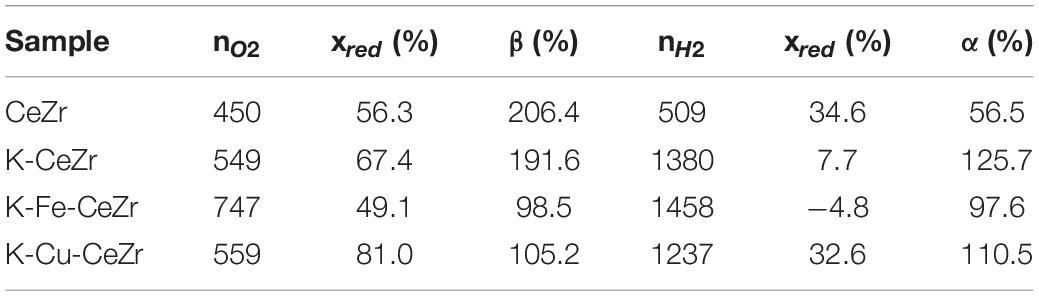
Table 2. Amount (in μmol/g) of released oxygen (as O2) and produced H2 during H2O splitting tests, reduction degrees after each step (xred), oxidation yields (α), and reduction yields (β).
The low temperature oxidation peak could be addressed to the contribution of surface and sub-surface layers and/or less ordered bulk structures to splitting reactions, while the high temperature peak could be related to the oxidation of bulk ceria-zirconia.
Figure 1 shows H2 and CO concentration profiles as obtained in CO2/H2O co-splitting tests and separate H2O and CO2 splitting experiments over co-doped materials. Figure 2 also shows the fuel amount produced over K-Fe-CeZr and K-Cu-CeZr catalysts.
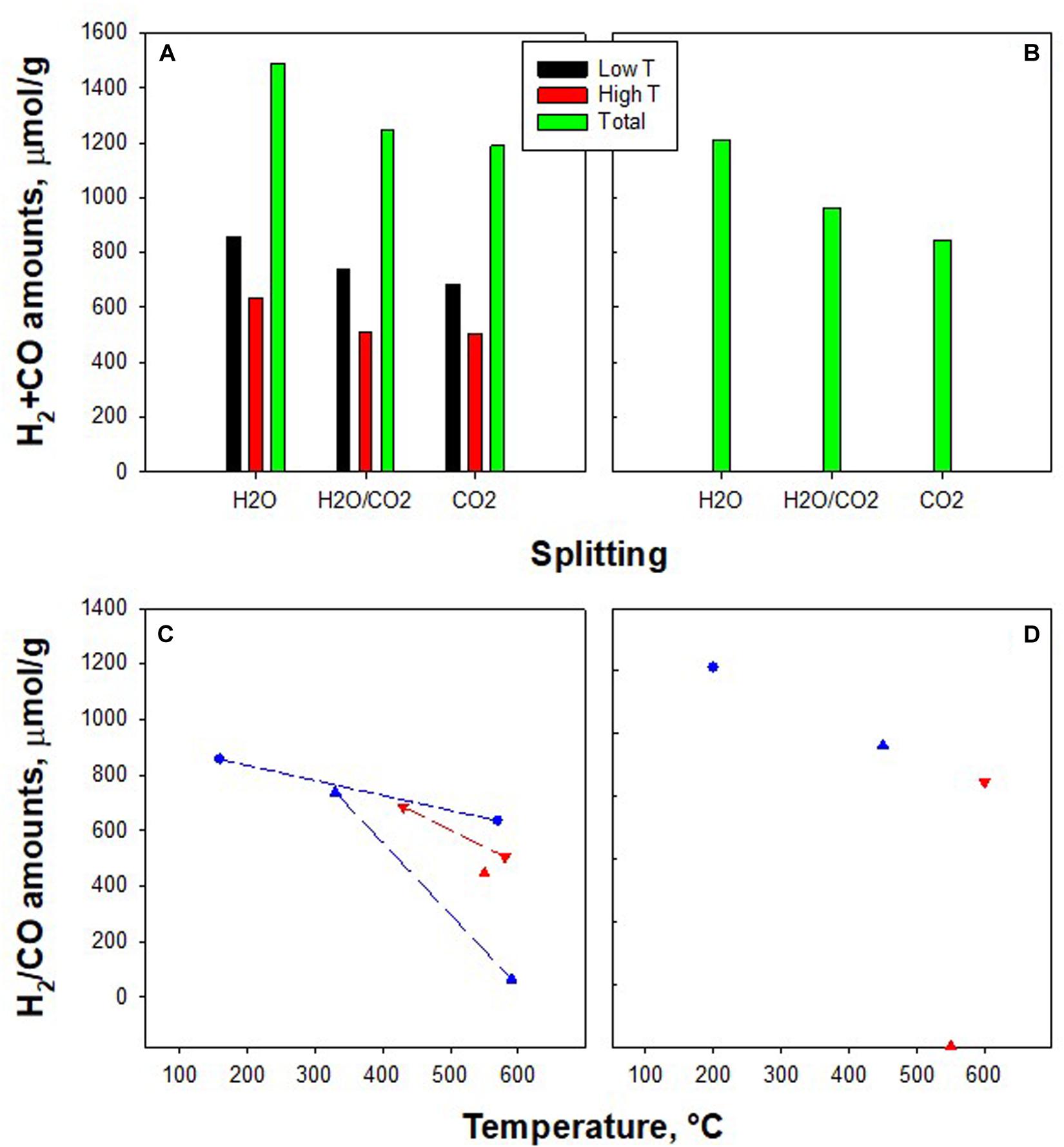
Figure 2. Quantitative analysis of splitting tests under different oxidants. Partial and overall amounts of H2 + CO on K-Fe-CeZr (A) and K-Cu-CeZr (B); H2 (blue symbols) and CO (red symbols) amounts during H2O splitting (•) co-splitting (▲), and CO2 splitting (▼) on K-Fe-CeZr (C) and K-Cu-CeZr (D).
K-Fe-CeZr sample shows two oxidation peaks independently from the oxidizing stream. In CO2 splitting, the former peak (450°C) occurs at a higher temperature than that found in H2O splitting. This result suggests that the H2O splitting reaction is faster than the CO2 reaction.
In H2O/CO2 co-splitting tests, the H2O was reduced first. However, H2O started reducing at 200°C reaching the peak at 330°C. This peak temperature is higher than bare H2O splitting conditions. This behavior can be addressed to the CO2 adsorption on the surface, inhibiting H2O adsorption and further reaction. Actually, in our previous papers, we showed that CO2 desorption on ceria-based materials occurs at about 200°C (Di Benedetto et al., 2013; Barbato et al., 2016).
Moreover, CO2 feed results in a lower produced H2 amount (Figure 2C). The second oxidation phenomenon occurs at the same temperature independently from the oxidizing stream (Figures 1, 2). As reported above, this peak is related to bulk oxidation. This reaction is controlled by the bulk-to-bulk oxygen diffusion and is thus not influenced by the chemical nature of the oxidant. However, during H2O/CO2 co-splitting only CO production is (unexpectedly) detected, suggesting a key role of gaseous molecules on the reaction pathway.
CO2 splitting on K-Cu-CeZr (Figure 1) features one CO production peak at about 600°C, confirming lower activity toward CO2 than the K-Fe-CeZr sample, which was also detected by TG analysis (Landi, 2019). In the H2O/CO2 co-splitting tests, the presence of CO2 shifts H2 production to higher temperatures (Figures 1, 2D) and reduces H2 amounts (Figures 2B,D), as reported for the K-Fe-CeZr sample. Furthermore, CO production is negligible, probably because the material is fully oxidized at a temperature lower than that of CO2 activation.
Kinetic Model of K-Fe-CeZr
The results of co-splitting tests (Figure 1) show that the H2O splitting reaction is faster than CO2. However, at high temperatures, the H2O splitting reaction is prevented and CO2 splitting is favored. To understand the observed behavior, we developed a kinetic model. As reported in section “Kinetic Model,” only the surface reactions have been modeled. The low temperature peaks of H2O and CO2 splitting tests were used as experimental data to calculate the kinetic parameters. Figures 3, 4 show the experimental and model curves as obtained in H2O and CO2 splitting tests, respectively. The results reported in Table 3, show a low value of the SRMSE, suggesting that the fitting is good.
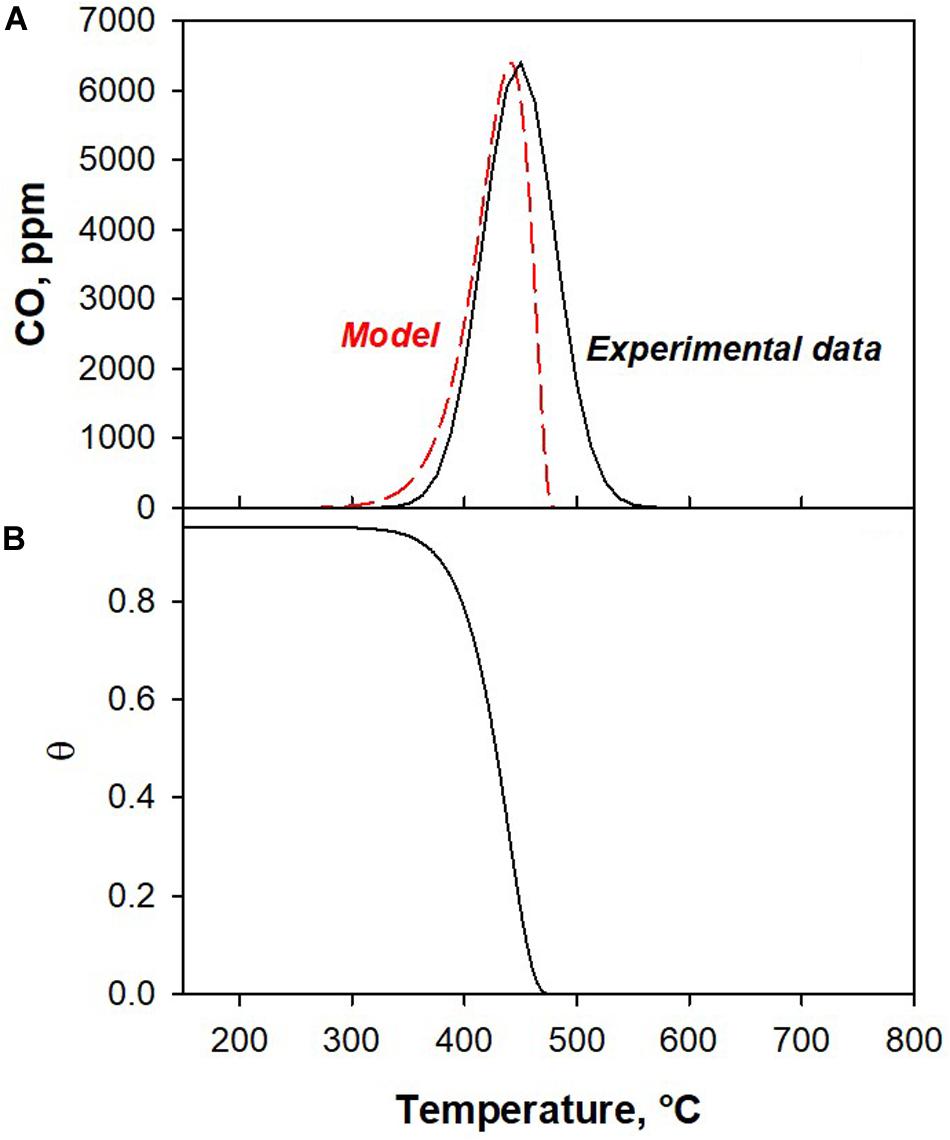
Figure 3. (A) Experimental and model CO production (low temperature peak, bare CO2 splitting) and (B) the corresponding surface site evolution calculated by the kinetic model.
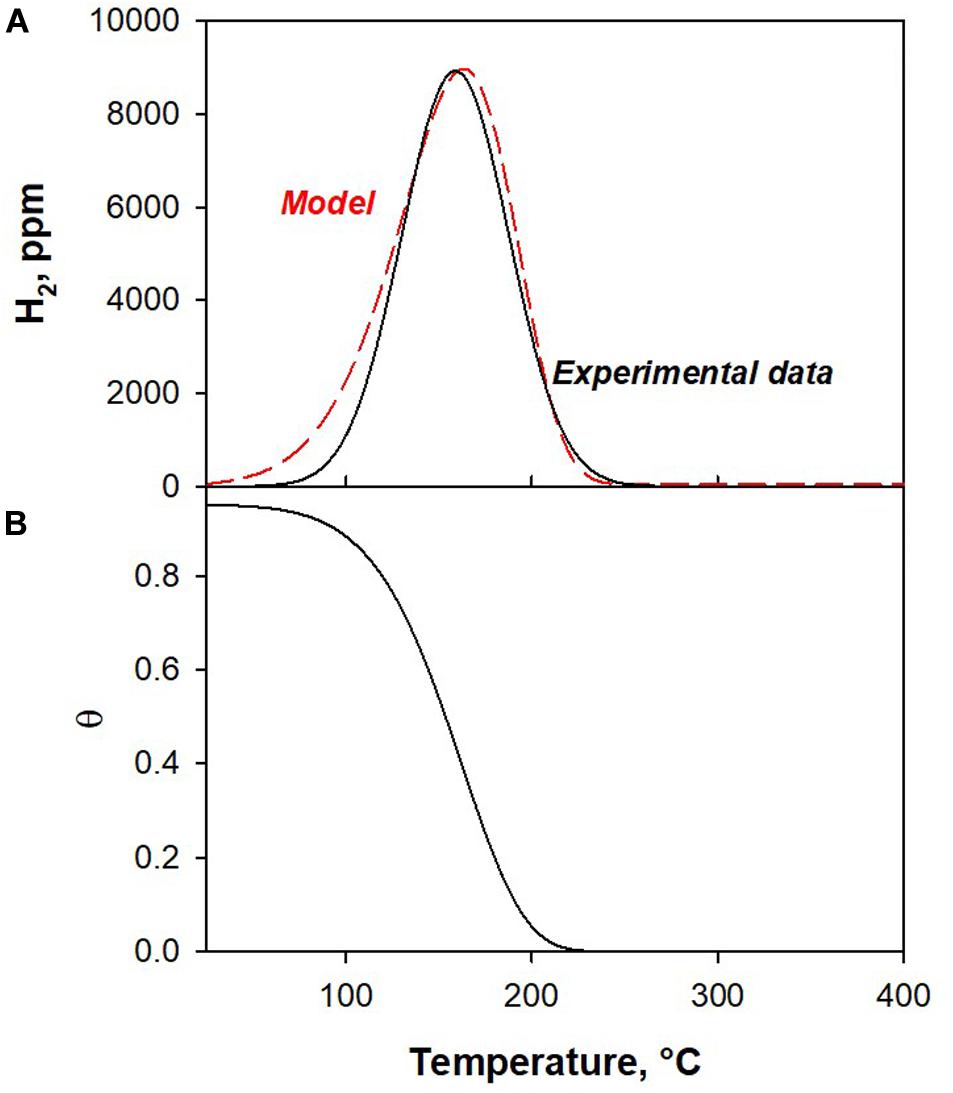
Figure 4. (A) Experimental and model H2 production (low temperature peak, bare H2O splitting) and (B) the corresponding surface site evolution calculated by the kinetic model.
From the fitting results, we conclude that the activation energy of the CO2 splitting reaction is higher than that of H2O splitting. This enables the H2O splitting reaction to proceed at a lower temperature than CO2, as indicated by the results of this experiment.
We can also note that the H2O oxidation reaction is in the first order for active sites concentration (nθ _H2O = 1); whereas the CO2 oxidation rate exhibits an order lower than 1 with respect to active sites concentration (nθ _CO2 = 0.75). This result suggests that CO2 needs a lower number of nearby reduced sites, as expected due to the different structure of the CO2 molecule.
To study the competition between the two reaction rates we defined the parameter R:
Figure 5 shows H2O and CO2 splitting reaction rates of on K-Fe-CeZr as calculated by the kinetic model as a function of the active sites concentration (θ), at different values of temperature. In these figures, θ values along the x-axis are decreasing.
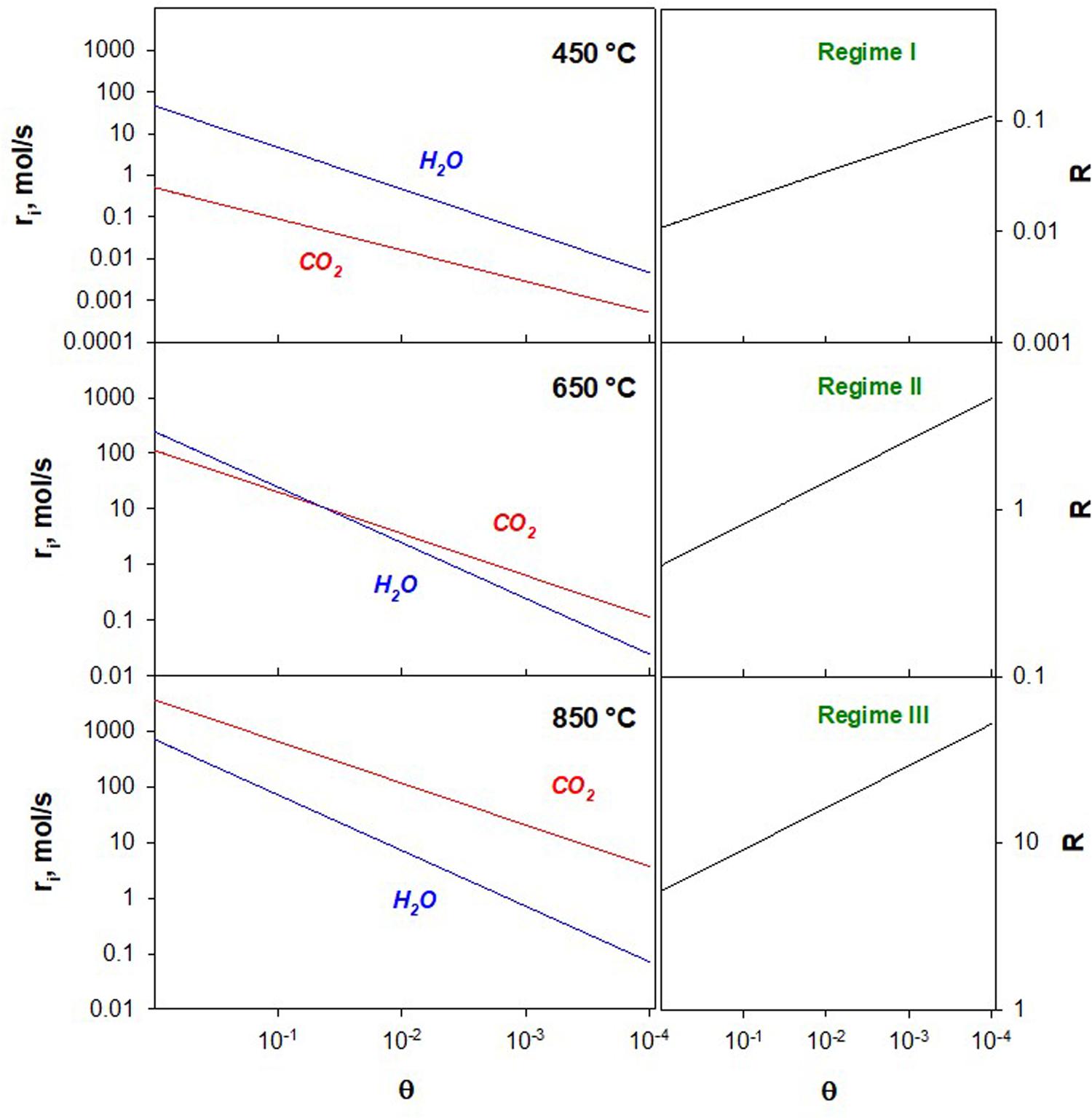
Figure 5. Surface reaction rates of H2O and CO2 splitting reaction (left) and their ratio R (right) as a function of decreasing reduced sites fraction on K-Fe-CeZr at different temperatures as calculated by the kinetic model.
Three regimes can be identified:
Regime I- H2O driven regime: @T ≤ 560°C, the reaction rates do not intersect and R < 1 in the whole θ range. H2 production prevails over CO production.
Regime II- mixed regime: @ 560 < T < 700°C, rCO2 and rH2O intersect. At low θ values R > 1, at high θ values R < 1. Reaction rates are of the same order of magnitude, suggesting a co-production of carbon monoxide and hydrogen.
Regime III: CO2 driven regime @ T > 700°C reaction rates do not intersect and R > 1 in the whole θ range. CO production prevails over H2 production.
Isothermal simulations were performed to compute the amount of H2 and CO produced as a function of temperature. Figure 6 shows the ratio between CO and H2 produced amounts (F, Eq. 32) as a function of temperature.
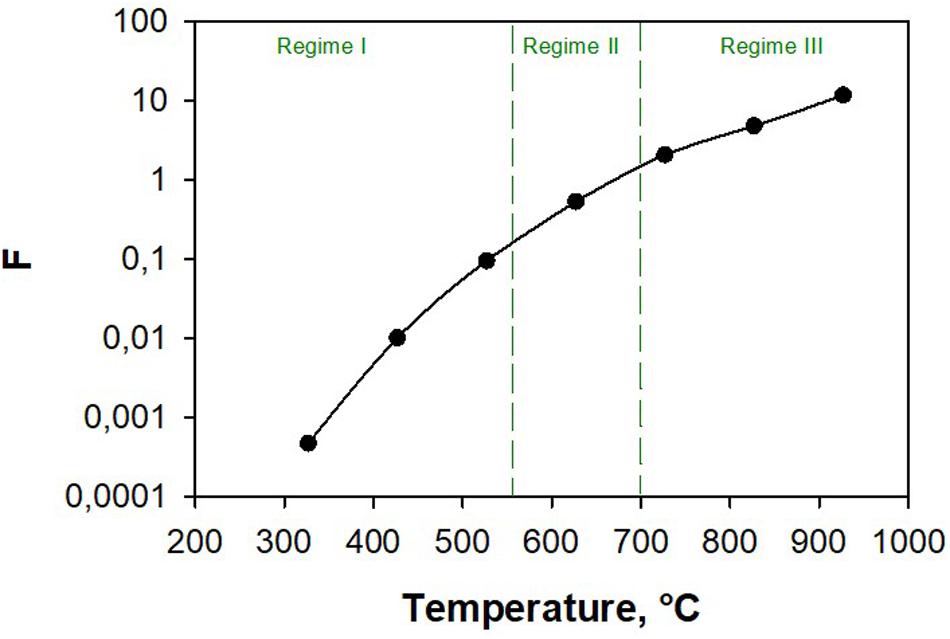
Figure 6. Ratio between CO and H2 production as a function of temperature during isothermal co-splitting simulations.
In this figure, the three regimes are also identified. It is worth noting that F = 1 at 670°C and inversion occurs at higher temperatures.
From these results, we conclude that by changing the temperature, the content of H2 and CO in the output current can be modulated.
Conclusion
In this work, ceria-zirconia was co-doped with transition metal (Cu2 +, Fe3 +) and potassium cations. We studied the co-doped ceria-zirconia to determine whether they act as catalysts for water and carbon dioxide co-splitting. All the investigated materials were able to convert separate feeds of CO2 and H2O into CO and H2, respectively. A single oxidation peak was detected on the K-Cu-CeZr catalyst, independently from the oxidant. Furthermore, the water splitting reaction rate appeared faster. Two oxidation peaks were detected on the K-Fe-CeZr catalyst for both the oxidants; the water reaction rate appeared faster at low temperature, while the high temperature peak seemed independent from the oxidant, suggesting that the reaction rate is limited by the oxygen diffusion from the surface to the bulk.
Co-splitting tests were carried out as temperature programmed oxidations. On the K-Cu-CeZr catalyst, water reacted faster and a negligible CO production was detected. the H2 production peak shifted to a higher temperature, probably due to CO2 adsorption on the catalyst surface, thus blocking active sites. The K-Fe-CeZr catalyst behavior was more complex. Two oxidation peaks were detected at low and high temperatures. For the low temperature, only H2 was produced in agreement with the faster H2O splitting kinetics measured during the separated H2O and CO2 splitting. In contrast, almost 100% selectivity to CO2 splitting was detected at high temperatures.
A kinetic model was developed to understand this behavior. This model revealed that the H2O splitting reaction featured a higher reaction order in terms of sites fraction compared to CO2. This suggests that H2 production requires a larger number of adjacent reduced sites. By comparing the calculated surface reaction rates at different temperatures, three regimes were identified. At temperatures below 560°C H2 production is faster than CO production independently from the surface reduced sites fraction; accordingly, isothermal co-splitting simulations showed a preeminent hydrogen production. At temperatures between 560 and 700°C, CO2 and H2O reaction rates are comparable and the pre-eminence depends on both temperature and surface reduced sites fraction. In this temperature range, co-production of CO and H2 occurs and their ratio can be tuned by an opportune choice of the co-splitting temperature. At temperatures higher than 700°C CO production is faster than H2 production independently from the surface reduced sites fraction, accordingly, isothermal co-splitting simulations showed a preeminent carbon monoxide production.
Data Availability Statement
The raw data supporting the conclusions of this article will be made available by the authors, without undue reservation.
Author Contributions
GLa and GLu prepared the materials and performed the experiments. AD developed the model and performed the simulations. GLa, GLu, and AD contributed to the planning and interpretation of results, and the writing of the manuscript. All authors contributed to the article and approved the submitted version.
Conflict of Interest
The authors declare that the research was conducted in the absence of any commercial or financial relationships that could be construed as a potential conflict of interest.
References
Abanades, S., and Le Gal, A. (2012). CO 2 splitting by thermo-chemical looping based on Zr xCe 1-xO 2 oxygen carriers for synthetic fuel generation. Fuel 102, 180–186. doi: 10.1016/j.fuel.2012.06.068
Abanades, S., Legal, A., Cordier, A., Peraudeau, G., Flamant, G., and Julbe, A. (2010). Investigation of reactive cerium-based oxides for H2 production by thermochemical two-step water-splitting. J. Mater. Sci. 45, 4163–4173. doi: 10.1007/s10853-010-4506-4504
Agrafiotis, C., Roeb, M., and Sattler, C. (2015). A review on solar thermal syngas production via redox pair-based water/carbon dioxide splitting thermochemical cycles. Renew. Sustain. Energy Rev. 42, 254–285. doi: 10.1016/j.rser.2014.09.039
Al-Shankiti, I., Al-Otaibi, F., Al-Salik, Y., and Idriss, H. (2013). Solar Thermal Hydrogen Production from Water over Modified CeO2 Materials. Top. Catal. 56, 1129–1138. doi: 10.1007/s11244-013-0079-71
Al-Shankiti, I., Ehrhart, B. D., and Weimer, A. W. (2017). Isothermal redox for H2O and CO2splitting – A review and perspective. Sol. Energy 156, 21–29. doi: 10.1016/j.solener.2017.05.028
Barbato, P. S., Colussi, S., Di Benedetto, A., Landi, G., Lisi, L., Llorca, J., et al. (2016). Origin of high activity and selectivity of CuO/CeO 2 catalysts prepared by solution combustion synthesis in CO-PROX reaction. J. Phys. Chem. C 120, 13039–13048. doi: 10.1021/acs.jpcc.6b02433
Bhosale, R. R., and AlMomani, F. (2020). Hydrogen production via solar driven thermochemical cerium oxide – cerium sulfate water splitting cycle. Int. J. Hydrogen Energy 45, 10381–10390. doi: 10.1016/j.ijhydene.2019.05.159
Bhosale, R. R., Kumar, A., Almomani, F., Ghosh, U., Al-Muhtaseb, S., Gupta, R., et al. (2016). Assessment of CexZryHfzO2based oxides as potential solar thermochemical CO2splitting materials. Ceram. Int. 42, 9354–9362. doi: 10.1016/j.ceramint.2016.02.100
Bhosale, R. R., Takalkar, G., Sutar, P., Kumar, A., AlMomani, F., and Khraisheh, M. (2019). A decade of ceria based solar thermochemical H2O/CO2 splitting cycle. Int. J. Hydrogen Energy 44, 34–60. doi: 10.1016/j.ijhydene.2018.04.080
Bhosale, R. R., and Takalkar, G. D. (2018). Nanostructured co-precipitated Ce0.9Ln0.1O2 (Ln = La, Pr, Sm, Nd, Gd, Tb, Dy, or Er) for thermochemical conversion of CO2. Ceram. Int. 44, 16688–16697. doi: 10.1016/j.ceramint.2018.06.096
Call, F., Roeb, M., Schmücker, M., Bru, H., Curulla-Ferre, D., Sattler, C., et al. (2013). Thermogravimetric analysis of Zirconia-Doped Ceria for thermochemical production of solar fuel. Am. J. Anal. Chem. 04, 37–45. doi: 10.4236/ajac.2013.410A1005
Charvin, P., Abanades, S., Beche, E., Lemont, F., and Flamant, G. (2009). Hydrogen production from mixed cerium oxides via three-step water-splitting cycles. Solid State Ionics 180, 1003–1010. doi: 10.1016/j.ssi.2009.03.015
Chen, Y., Zhu, X., Li, K., Wei, Y., Zheng, Y., and Wang, H. (2019). Chemical Looping Co-splitting of H 2 O–CO 2 for Efficient Generation of Syngas. ACS Sustain. Chem. Eng. 7, 15452–15462. doi: 10.1021/acssuschemeng.9b02996
Cho, H. S., Kodama, T., Gokon, N., Kim, J. K., Lee, S. N., and Kang, Y. H. (2017). “Development and experimental study for hydrogen production from the thermochemical two-step water splitting cycles with a CeO2 coated new foam device design using solar furnace system,” in Proceedings of the AIP Conference, (College Park, MD: AIP), 100003. doi: 10.1063/1.4984460
Chuayboon, S., and Abanades, S. (2020). An overview of solar decarbonization processes, reacting oxide materials, and thermochemical reactors for hydrogen and syngas production. Int. J. Hydrogen Energy. 45, 25783–25810. doi: 10.1016/j.ijhydene.2020.04.098
Cooper, T., Scheffe, J. R., Galvez, M. E., Jacot, R., Patzke, G., and Steinfeld, A. (2015). Lanthanum Manganite Perovskites with Ca/Sr A-site and Al B-site doping as effective oxygen exchange materials for solar thermochemical fuel production. Energy Technol. 3, 1130–1142. doi: 10.1002/ente.201500226
Costa Oliveira, F. A., Barreiros, M. A., Abanades, S., Caetano, A. P. F., Novais, R. M., and Pullar, R. C. (2018). Solar thermochemical CO 2 splitting using cork-templated ceria ecoceramics. J. CO2 Util. 26, 552–563. doi: 10.1016/j.jcou.2018.06.015
Costa Oliveira, F. A., Barreiros, M. A., Haeussler, A., Caetano, A. P. F., Mouquinho, A. I, Oliveira e Silva, P. M., et al. (2020). High performance cork-templated ceria for solar thermochemical hydrogen productionviatwo-step water-splitting cycles. Sustain. Energy Fuels 4, 3077–3089. doi: 10.1039/d0se00318b
de la Calle, A., and Bayon, A. (2019). Annual performance of a thermochemical solar syngas production plant based on non-stoichiometric CeO 2. Int. J. Hydrogen Energy 44, 1409–1424. doi: 10.1016/j.ijhydene.2018.11.076
Di Benedetto, A., Landi, G., Lisi, L., and Russo, G. (2013). Role of CO2 on CO preferential oxidation over CuO/CeO2 catalyst. Appl. Catal. B Environ. 142–143, 169–177. doi: 10.1016/j.apcatb.2013.05.001
Falter, C., and Pitz-Paal, R. (2018). Energy analysis of solar thermochemical fuel production pathway with a focus on waste heat recuperation and vacuum generation. Sol. Energy 176, 230–240. doi: 10.1016/j.solener.2018.10.042
Furler, P., Scheffe, J. R., and Steinfeld, A. (2012). Syngas production by simultaneous splitting of H 2O and CO 2via ceria redox reactions in a high-temperature solar reactor. Energy Environ. Sci. 5, 6098–6103. doi: 10.1039/c1ee02620h
Gokon, N., Sagawa, S., and Kodama, T. (2013). Comparative study of activity of cerium oxide at thermal reduction temperatures of 1300-1550 C for solar thermochemical two-step water-splitting cycle. Int. J. Hydrogen Energy 38, 14402–14414. doi: 10.1016/j.ijhydene.2013.08.108
Gokon, N., Suda, T., and Kodama, T. (2015a). Oxygen and hydrogen productivities and repeatable reactivity of 30-mol%- Fe-, Co-, Ni-, Mn-doped CeO2-δ for thermochemical two-step water-splitting cycle. Energy 90, 1280–1289. doi: 10.1016/j.energy.2015.06.085
Gokon, N., Suda, T., and Kodama, T. (2015b). Thermochemical reactivity of 5–15mol% Fe, Co, Ni, Mn-doped cerium oxides in two-step water-splitting cycle for solar hydrogen production. Thermochim. Acta 617, 179–190. doi: 10.1016/j.tca.2015.08.036
Haeussler, A., Abanades, S., Julbe, A., Jouannaux, J., Drobek, M., Ayral, A., et al. (2020). Remarkable performance of microstructured ceria foams for thermochemical splitting of H2O and CO2 in a novel high–temperature solar reactor. Chem. Eng. Res. Des. 156, 311–323. doi: 10.1016/j.cherd.2020.02.008
Hao, Y., Jin, J., and Jin, H. (2020). Thermodynamic analysis of isothermal CO2 splitting and CO2-H2O co-splitting for solar fuel production. Appl. Therm. Eng. 166:113600. doi: 10.1016/j.applthermaleng.2019.04.010
Jacot, R., Moré, R., Michalsky, R., Steinfeld, A., and Patzke, G. R. (2017). Trends in the phase stability and thermochemical oxygen exchange of ceria doped with potentially tetravalent metals. J. Mater. Chem. A 5, 19901–19913. doi: 10.1039/C7TA04063F
Jarrett, C., Chueh, W., Yuan, C., Kawajiri, Y., Sandhage, K. H., and Henry, A. (2016). Critical limitations on the efficiency of two-step thermochemical cycles. Sol. Energy 123, 57–73. doi: 10.1016/j.solener.2015.09.036
Kaneko, H., Taku, S., and Tamaura, Y. (2011). Reduction reactivity of CeO2-ZrO2 oxide under high O2 partial pressure in two-step water splitting process. Sol. Energy 85, 2321–2330. doi: 10.1016/j.solener.2011.06.019
Kang, M., Zhang, J., Wang, C., Wang, F., Zhao, N., Xiao, F., et al. (2013). CO2 splitting via two step thermochemical reactions over doped ceria/zirconia solid solutions. RSC Adv. 3, 18878–18885. doi: 10.1039/C3RA43742F
Landi, G. (2019). “Improving thermochemical splitting performance of ceria based materials: novel preparation routes, doping and co-doping,” in International Conference on Nanoscience and Materials World (NSMW 2019) Barcelona, Spain. Available online at: https://www.coalesceresearchgroup.com/conferences/nanoscience
Le Gal, A., and Abanades, S. (2011). Catalytic investigation of ceria-zirconia solid solutions for solar hydrogen production. Int. J. Hydrogen Energy 36, 4739–4748. doi: 10.1016/j.ijhydene.2011.01.078
Le Gal, A., and Abanades, S. (2012). Dopant incorporation in ceria for enhanced water-splitting activity during solar thermochemical hydrogen generation. J. Phys. Chem. C 116, 13516–13523. doi: 10.1021/jp302146c
Le Gal, A., Abanades, S., Bion, N., Le Mercier, T., and Harlé, V. (2013). Reactivity of doped ceria-based mixed oxides for solar thermochemical hydrogen generation via two-step water-splitting cycles. Energy Fuels 27, 6068–6078. doi: 10.1021/ef4014373
Lin, F., Samson, V. A., Wismer, A. O., Grolimund, D., Alxneit, I., and Wokaun, A. (2016). Zn-modified ceria as a redox material for thermochemical H 2 O and CO 2 splitting: effect of a secondary ZnO phase on its thermochemical activity. CrystEngComm 18, 2559–2569. doi: 10.1039/C6CE00430J
Lorentzou, S., Dimitrakis, D., Zygogianni, A., Karagiannakis, G., and Konstandopoulos, A. G. (2017). Thermochemical H2O and CO2 splitting redox cycles in a NiFe2O4 structured redox reactor: design, development and experiments in a high flux solar simulator. Sol. Energy 155, 1462–1481. doi: 10.1016/j.solener.2017.07.001
Lorentzou, S., Karagiannakis, G., Pagkoura, C., Zygogianni, A., and Konstandopoulos, A. G. (2014). Thermochemical CO2 and CO2/H2O splitting over NiFe2O4 for solar fuels synthesis. Energy Procedia 47, 1999–2008. doi: 10.1016/j.egypro.2014.03.212
Luciani, G., Landi, G., Aronne, A., and Di Benedetto, A. (2018). Partial substitution of B cation in La0.6Sr0.4MnO3perovskites: a promising strategy to improve the redox properties useful for solar thermochemical water and carbon dioxide splitting. Sol. Energy 171, 1–7. doi: 10.1016/j.solener.2018.06.058
Luciani, G., Landi, G., Imparato, C., Vitiello, G., Deorsola, F. A., Di Benedetto, A., et al. (2019). Improvement of splitting performance of Ce0.75Zr0.25O2 material: tuning bulk and surface properties by hydrothermal synthesis. Int. J. Hydrogen Energy 44, 17565–17577. doi: 10.1016/j.ijhydene.2019.05.021
Mao, Y., Gao, Y., Dong, W., Wu, H., Song, Z., Zhao, X., et al. (2020). Hydrogen production via a two-step water splitting thermochemical cycle based on metal oxide – A review. Appl. Energy 267:114860. doi: 10.1016/j.apenergy.2020.114860
Mostrou, S., Büchel, R., Pratsinis, S. E., and van Bokhoven, J. A. (2017). Improving the ceria-mediated water and carbon dioxide splitting through the addition of chromium. Appl. Catal. A Gen. 537, 40–49. doi: 10.1016/j.apcata.2017.03.001
Muhich, C., and Steinfeld, A. (2017). Principles of doping ceria for the solar thermochemical redox splitting of H 2 O and CO 2. J. Mater. Chem. A 5, 15578–15590. doi: 10.1039/C7TA04000H
Muhich, C. L., Ehrhart, B. D., Al-Shankiti, I., Ward, B. J., Musgrave, C. B., and Weimer, A. W. (2016). A review and perspective of efficient hydrogen generation via solar thermal water splitting. Wiley Interdiscip. Rev. Energy Environ. 5, 261–287. doi: 10.1002/wene.174
Naghavi, S. S., He, J., and Wolverton, C. (2020). CeTi2O6 - A Promising Oxide for Solar Thermochemical Hydrogen Production. ACS Appl. Mater. Interfaces 12, 21521–21527. doi: 10.1021/acsami.0c01083
Nguyen, V. N., and Blum, L. (2015). Syngas and Synfuels from H 2 O and CO 2: current status. Chemie Ing. Tech. 87, 354–375. doi: 10.1002/cite.201400090
Pappacena, A., Boaro, M., Armelao, L., Llorca, J., and Trovarelli, A. (2016). Water splitting reaction on Ce0.15Zr0.85O2 driven by surface heterogeneity. Catal. Sci. Technol. 6, 1–9. doi: 10.1039/C5CY01337B
Pappacena, A., Rancan, M., Armelao, L., Llorca, J., Ge, W., Ye, B., et al. (2017). New Insights into the Dynamics That Control the Activity of Ceria-Zirconia Solid Solutions in Thermochemical Water Splitting Cycles. J. Phys. Chem. C 121, 17746–17755. doi: 10.1021/acs.jpcc.7b06043
Petkovich, N. D., Rudisill, S. G., Venstrom, L. J., Boman, D. B., Davidson, J. H., and Stein, A. (2011). Control of heterogeneity in nanostructured Ce1-xZr xO2 Binary oxides for enhanced thermal stability and water splitting activity. J. Phys. Chem. C 115, 21022–21033. doi: 10.1021/jp2071315
Portarapillo, M., Aronne, A., Di Benedetto, A., Imparato, C., Landi, G., and Luciani, G. (2019). Syngas production through H2O/CO2 thermochemical splitting. Chem. Eng. Trans. 74, 43–48. doi: 10.3303/CET1974008
Rao, C. N. R., and Dey, S. (2015). Generation of H2 and CO by solar thermochemical splitting of H2O and CO2 by employing metal oxides. J. Solid State Chem. 242, 107–115. doi: 10.1016/j.jssc.2015.12.018
Takacs, M., Scheffe, J. R., and Steinfeld, A. (2015). Oxygen nonstoichiometry and thermodynamic characterization of Zr doped ceria in the 1573–1773 K temperature range. Phys. Chem. Chem. Phys. 17, 7813–7822. doi: 10.1039/C4CP04916K
Takalkar, G., Bhosale, R. R., and AlMomani, F. (2019). Thermochemical splitting of CO2 using Co-precipitation synthesized Ce0.75Zr0.2M0.05O2-δ (M = Cr, Mn, Fe, CO, Ni, Zn) materials. Fuel 256:115834. doi: 10.1016/j.fuel.2019.115834
Takalkar, G., Bhosale, R. R., AlMomani, F., and Rashid, S. (2020a). Co-precipitation synthesized nanostructured Ce0.9Ln0.05Ag0.05O2-δ materials for solar thermochemical conversion of CO2 into fuels. J. Mater. Sci. 55, 9748–9761. doi: 10.1007/s10853-020-04567-w
Takalkar, G., Bhosale, R. R., Rashid, S., AlMomani, F., Shakoor, R. A., and Al Ashraf, A. (2020b). Application of Li-, Mg-, Ba-, Sr-, Ca-, and Sn-doped ceria for solar-driven thermochemical conversion of carbon dioxide. J. Mater. Sci. 55, 11797–11807. doi: 10.1007/s10853-020-04875-4871
Takalkar, G. D., Bhosale, R. R., Kumar, A., AlMomani, F., Khraisheh, M., Shakoor, R. A., et al. (2018). Transition metal doped ceria for solar thermochemical fuel production. Sol. Energy 172, 204–211. doi: 10.1016/j.solener.2018.03.022
Tou, M., Jin, J., Hao, Y., Steinfeld, A., and Michalsky, R. (2019). Solar-driven co-thermolysis of CO2 and H2O promoted by: in situ oxygen removal across a non-stoichiometric ceria membrane. React. Chem. Eng. 4, 1431–1438. doi: 10.1039/c8re00218e
Zhao, B., Huang, C., Ran, R., Wu, X., and Weng, D. (2016). Two-step thermochemical looping using modified ceria-based materials for splitting CO2. J. Mater. Sci. 51, 2299–2306. doi: 10.1007/s10853-015-9534-9537
Keywords: thermochemical water splitting, thermochemical CO2 splitting, sustainable energy, transition metals, potassium, ceria-zirconia, kinetic modeling
Citation: Luciani G, Landi G and Di Benedetto A (2020) Syngas Production Through H2O/CO2 Thermochemical Splitting Over Doped Ceria-Zirconia Materials. Front. Energy Res. 8:204. doi: 10.3389/fenrg.2020.00204
Received: 26 May 2020; Accepted: 31 July 2020;
Published: 05 October 2020.
Edited by:
Alfonso Chinnici, University of Adelaide, AustraliaReviewed by:
Mahyar Silakhori, University of Adelaide, AustraliaGang Liu, Central South University, China
Copyright © 2020 Luciani, Landi and Di Benedetto. This is an open-access article distributed under the terms of the Creative Commons Attribution License (CC BY). The use, distribution or reproduction in other forums is permitted, provided the original author(s) and the copyright owner(s) are credited and that the original publication in this journal is cited, in accordance with accepted academic practice. No use, distribution or reproduction is permitted which does not comply with these terms.
*Correspondence: Gianluca Landi, Z2lhbmx1Y2EubGFuZGlAY25yLml0