- 1Synthetic and Systems Biology Innovation Hub, Texas A&M University, College Station, TX, United States
- 2Department of Plant Pathology and Microbiology, Texas A&M University, College Station, TX, United States
- 3Department of Chemical & Biomolecular Engineering, The University of Tennessee, Knoxville, Knoxville, TN, United States
- 4Department of Chemical Engineering, Texas A&M University, College Station, TX, United States
- 5Joint Institute of Biological Sciences, Biosciences Division, Oak Ridge National Laboratory, Oak Ridge, TN, United States
- 6Department of Forestry, Wildlife, and Fisheries, Center for Renewable Carbon, The University of Tennessee Institute of Agriculture, Knoxville, TN, United States
- 7College of Life Science and Technology, Huazhong University of Science and Technology, Wuhan, China
The sustainability and economic feasibility of modern biorefinery depend on the efficient processing of both carbohydrate and lignin fractions for value-added products. By mimicking the biomass degradation process in white-rote fungi, a tailored two-step fractionation process was developed to maximize the sugar release from switchgrass biomass and to optimize the lignin processability for bioconversion. Biomimicking biomass processing using Formic Acid: Fenton: Organosolv (F2O) and achieved high processability for both carbohydrate and lignin. Specifically, switchgrass pretreated by the F2O process had 99.6% of the theoretical yield for glucose release. The fractionated lignin was also readily processable by fermentation via Rhodococcus opacus PD630 with a lipid yield of 1.16 g/L. Scanning electron microscope analysis confirmed the fragmentation of switchgrass fiber and the cell wall deconstruction by the F2O process. 2D-HSQC NMR further revealed the cleavage of aryl ether linkages (β-O-4) in lignin components. These results revealed the mechanisms for efficient sugar release and lignin bioconversion. The F2O process demonstrated effective mimicking of natural biomass utilization system and paved a new path for improving the lignin and carbohydrate processability in next generation lignocellulosic biorefinery.
Introduction
The demands for renewable energy have become urgent in recent years due to the increases in greenhouse gas emissions and various other environmental concerns associated with fossil fuel consumption (Himmel et al., 2007). Lignocellulosic biomass has been considered one of the most important sources of renewable energy (Yuan et al., 2008; Hu and Ragauskas, 2012). Perennial feedstock, such as switchgrass, could provide environmental and economic advantages over the current corn-based ethanol, considering the higher net energy gain and the fact that it requires only marginal land use (Yuan et al., 2011). Despite the potential it presents, lignocellulosic biomass conversion is a more challenging process due to the recalcitrant nature of biomass and the need to disrupt secondary cell wall structure to release carbohydrate and lignin (Isikgor and Becer, 2015; Balch et al., 2017).
To enable the cellulose for efficient hydrolysis, many pretreatment methods such as steam explosion, diluted acid, hot water, and organic solvents have been developed to overcome the recalcitrance (Lau et al., 2009; Zhao et al., 2009; Lima et al., 2013; Zhang Z. et al., 2016; Zhuang et al., 2016). Despite extensive research, most of these pretreatment processes were developed by solely considering the hydrolysis of carbohydrate, while lignin is considered as a waste stream. Such a strategy poses a significant challenge in the sustainability and economic feasibility of lignocellulosic biorefinery. For lignocellulosic biorefinery to be viable, all cell wall components need to be processed into value-added products in a similar way to corn ethanol refinery and petroleum refinery. For example, corn-ethanol biorefineries produce multiple product streams, including ethanol, dried distillers’ grains (DDG), and corn oil. Similarly, petroleum refineries convert the entire crude oil feedstock to multiple maximum-value products. Thus, a fundamental biomass processing strategy is required to synergistically improve the processability for both carbohydrate and lignin, to improve net energy gain, and thus enhance overall sustainability and profitability (Liu et al., 2018, 2019a). However, even though lignin is the main constituent of the plant cell wall and has higher energy content than cellulose, it has a recalcitrant complex structure with plentiful aromatic moieties, which present significant challenges for its potential use as a valuable resource for bioenergy and biomaterial (Matsakas et al., 2018). As a consequence, efficient biomass processing could not only improve the fermentable sugar release but also maximize lignin (Xie et al., 2017b; Xu et al., 2019).
Many natural biomass utilization systems (NBUS) have already evolved the capacity to co-process lignin and carbohydrates (Wei et al., 2011; Xie et al., 2014; Wang et al., 2018). One such system is white-rot fungi for biomass decomposition (Quinlan et al., 2011; Wei et al., 2011). White-rot fungi have a strong processing ability to overcome the recalcitrant lignin barriers, deconstruct both carbohydrate and lignin, and process all three components (i.e., cellulose, hemicellulose, and lignin) of the plant cell wall. The strong redox network exploited by white-rot fungi can effectively break down lignin for downstream processing, and such a system could be mimicked to maximize both carbohydrate and lignin processability. During the lignin biodegradation process (Rouches et al., 2016; Qin et al., 2018), white-rot fungi produce multiple types of reactive radicals by both enzymes and Fenton reaction reagents, as well as organic acid, such as oxalic acid to efficiently disrupt the lignocellulose matrix (Rudakiya and Gupte, 2017; Kameshwar and Qin, 2018). The integration of reactive radicals and organic acid can be exploited to establish a new process for lignocellulosic biomass conversion (Sun et al., 2016, 2017). The previous pretreatment, by mimicking the Fenton reaction, was designed to focus on the enzymatic hydrolysis performance of the lignocellulosic biomass (Jung et al., 2015), while the lignin processibility was not discussed. It is also reported that the combination of sonocatalytic reaction and Fenton reaction exhibited enhanced hydroxyl (⋅OH) radical generation and lignin degradation (Ninomiya et al., 2013). The low molecular weight lignin could be catabolized to central metabolites (Johnson and Beckham, 2015) and then converted to value-added products by microbial organisms (Abdelaziz et al., 2016).
Formic acid is an effective reagent to depolymerize lignin into low molecular-mass aromatics (Feng et al., 2016). As compared to dilute acid and other leading pretreatment technologies, formic acid can be used for lignocellulosic biomass treatment under relatively mild temperature and conditions (Kim et al., 2016; Zhang K. et al., 2016). Moreover, formic acid can be recycled throughout the pretreatment process to reduce the reagent cost. Formic acid can produce several reactive radicals, like HOO⋅ and ⋅COOH, in the presence of hydrogen peroxide (Vel Leitner and Dore, 1996; Davies et al., 2011). To mimic the redox environment with multiple reactive radicals in the aforementioned white-rot fungi, ferrous was also added with hydrogen peroxide to produce hydroxyl radical via Fenton reaction, which is critical for both lignin depolymerization and reduce cellulose crystallinity (Barr and Aust, 1994; Sun et al., 2016). Therefore, in this study, a biomimicking system was established by adding formic acid, hydrogen peroxide, and ferrous to improve the carbohydrates and lignin processability for bioconversion. The pretreatment performance of the formic acid together with the catalysis of low concentrations of Fenton reagent has been first investigated for switchgrass. The combination of formic acid and Fenton reaction mimic the natural conversion process for lignocellulosic biomass by white-rot fungi. The mimicking process can both guide the advancement of biorefinery design and elucidate the mechanisms of natural processes. Moreover, white-rot fungi can utilize both lignin and carbohydrate in nature. It is critical to evaluate if such a bio-mimicking process can help to address one of the most challenging issues in biorefinery, the lignin utilization. We, therefore, extracted the lignin from the F2O pretreatment using dioxane and evaluate the potential of valorization by microorganism conversion (Pothiraj et al., 2006; Lee et al., 2019). The results highlighted that this biomimicking process can synergistically improve both carbohydrate and lignin processibility in a similar way to white-rot fungus. The lignin residues from biomass pretreatment are always considered as waste (Pothiraj et al., 2006). Overall, the Formic Acid: Fenton: Organosolv (F2O) pretreatment process developed in this study could overcome the traditional refinery challenges of low fermentable sugar yield and inefficient lignin utilization. This study could thus open new avenues for biorefinery design towards the co-utilization of carbohydrate and lignin processibility.
Materials and Methods
F2O Pretreatment Condition
Switchgrass was air dried and ground to pass through a 20-mesh sieve, then stored in zip-lock bags before use. The pretreatment was conducted in a 1 L reactor equipped with a condenser under normal pressure. The reactor was filled with 10 g switchgrass and 100 ml formic acid at 100.8°C for 2 h on a benchtop heater followed by the addition of 5 mL H2O2 (30%), and 0.5 mL 0.05 M FeSO4. The mixture was cooked for 1 h and then another addition of the two Fenton reagents was provided and the mixture was cooked for a further 1 h.
After the first step pretreatment, formic acid was evaporated under the rotary evaporator. The solids were filled with 100 mL dioxane at 101.0°C for 1 h. The pretreated mixture was cooled at room temperature and centrifuged at 4000 rpm for 20 min. The solids were then washed with dioxane three times until the eluent was clear in color (Saha et al., 2019). The liquid was evaporated by rotary evaporated until a small volume remained, and dried up at 101°C. A scheme of the F2O process is provided in Supplementary Figure 1.
Enzymatic Hydrolysis
For enzymatic hydrolysis, 300 mg of pretreated solid and raw material was placed in a 25 mL glass vial. The filter paper activity (FPU) of Cellic CTec2 was 96 FPU/mL. To each vial we added 10 mL of sodium citrate buffer (pH 4.8), 0.05 mL (16 FPU/g solid loading) CTec2, and 0.005 mL HTec2. The vials were kept in a rotary incubator at 50°C and 60 rpm for 120 h. The sugar conversions were calculated by following previous studies (Jin et al., 2012; Alonso et al., 2013; Liu et al., 2019b).
Lipid Fermentation and Lignin Extraction Using F2O Lignin
Rhodococcus opacus PD630 was purchased from Leibniz Institute DSMZ-German Collection of Microorganisms and Cell Cultures (Braunschweig, Germany). Engineered R. opacus PD630_FA was used in this study. A single colony of R. opacus PD630 was inoculated in 10ml TSB medium at 28°C for three days and then inoculated into 100 mL minimal medium prepared as follows to an OD600 4.0.
About 1.4g (NH4)2SO4 and 1.0 g MgSO4.7H2O were added into 922 mL of ddH2O and autoclaved then cooled to room temperature. Forty milliliter sterilized 50%(w/v) glucose, 1 mL CaCL2.2H2O, 1 mL trace element solution, 1 mL stock A solution, 35.2 mL 1.0 M phosphate buffer were added to the solution and finalized to 1 L. Trace element and stock A solutions were prepared according to the methods used in previous studies by He Y. et al. (2017).
Formic Acid: Fenton: Organosolv lignin medium was dried and dissolved in minimal medium (without glucose) with a solid concentration of 30 g/L. The medium was autoclaved and then the PH was adjusted to 7.0 for three times. R. opacus PD630 was cultured in the F2O lignin medium with an initial OD600 5 for 3 days with an additional fed batch for another 3 days. The cells were harvested and lipid was purified through the hexane extraction method described by Xie et al. (2017b).
Cellulolytic Enzyme Lignin Isolation
Extractives-free untreated switchgrass and F2O pretreated switchgrass were balled milled in a dioxide vessel (50 mL) containing ZrO2 ball bearings (10 mm × 10) for 2 h (5 min grinding and 5 min break). The ball-milled biomass was then hydrolyzed by enzyme mixtures composed of cellulase, hemicellulase, and β-glucosidase [Cellic® CTec2 (0.1 mL/g biomass) and HTec2 (0.1 mL/g biomass) from Novozymes] following NREL’s standard procedure “enzymatic saccharification of lignocellulosic biomass” (Selig et al., 2008). The supernatants were removed by centrifugation, and the solid residues were hydrolyzed again under the same conditions with fresh enzyme mixtures. The residue was extracted by 96% dioxane at ambient temperature for 48 h. The cellulolytic enzyme lignin (CEL) was recovered from the dioxane extracts using a rotary evaporator, freeze-dried, and vacuum dried for further analysis.
Nuclear Magnetic Resonance Analysis of Lignin Fraction
The ball milled lignin samples (∼50 mg) were dissolved in a mixture of DMSO-d6 and HMPA-d18 (v/v = 4:1) for the 2D HSQC NMR analysis. A two-dimensional (2D) 1H–13C heteronuclear single quantum coherence (HSQC) nuclear magnetic resonance (NMR) experiment was performed at 300 K using a Bruker Avance-III 500 MHz spectrometer equipped with a 5 mm cryogenically cooled probe. The spectra were measured with a spectral width of 11 ppm in F2 (1H, 2048 data points) and 190 ppm in F1 (13C, 256 data points). 64 scans and 1 s delay was used for each sample (Yoo et al., 2017).
A stock solution of pyridine/CDCl3 (v/v = 1.6/1) was prepared first. The chromium acetylacetonate (relaxation reagent) and endo-N-hydroxy-5-norbornene-2,3-dicarboximide (internal standard) were then added to the stock solution. Lignin samples (25 mg) were dissolved in the solution mixture, and then derivatized using 2-chloro-4,4,5,5-tetramethyl-1,3,2-dioxaphospholane (TMDP). The 31P NMR spectra were acquired using a Bruker Avance-III 500 MHz spectrometer. The following parameters were employed in the NMR experiments: inverse-gated decoupling pulse sequence, 1.2 s acquisition time, 25 s pulse delay, 90° pulse angle, and 64 scans. The data were analyzed using Mestrenova software (Hao et al., 2018).
Gel-Permeation Chromatography
The lignin samples (dried under vacuum at 40°C overnight) were acetylated with acetic anhydride/pyridine (1/1, v/v) at ambient temperature for 24 h in a sealed flask under an inert atmosphere. The concentration of the lignin in the solution was approximately 2 mg/mL. After 24 h, the solution was diluted with ∼20 mL of ethanol and stirred for an additional 30 min, after which the solvents were removed with a rotary evaporator followed by rotary evaporation at 40 °C. Before gel-permeation chromatography (GPC) analysis, the acetylated lignin samples were dissolved in tetrahydrofuran (THF, 1.0 mg/mL), filtered through a 0.45 μm filter, and placed in a 1 mL auto-sampler vial. The molecular weight distributions of the acetylated lignin samples were then analyzed on an Agilent GPC SECurity 1200 system equipped with four Waters Styragel columns (HR1, HR2, HR4, HR6), an Agilent refractive index (RI) detector, and an Agilent UV detector (270 nm), using THF as the mobile phase (1.0 mL/min), with an injection volume of 20.0 μL. A standard polystyrene sample was used for calibration. The number-average molecular weight (Mn) and weight-average molecular weight (Mw) was determined by GPC.
Field-Emission Scanning Electron Microscope
The morphologies of the switchgrass biomass before and after the F2O pretreatment were observed by an FEI Quanta 600F Field-Emission Scanning Electron Microscope (FE-SEM; FEI Company, Hillsboro, OR). The samples were prepared as previously reported (Li et al., 2017). Briefly, the biomass was firstly mounted on a sample stands and then coated with 10 nm gold-The FE-SEM was operated at 5 kV accelerating voltage and 10 mm working distances.
Biomass Compositional Analysis
The cellulose, hemicellulose and lignin contents of the raw and F2O treated switchgrass were determined following NREL Laboratory Analytical Procedure (Sluiter et al., 2012). For composition analysis, switchgrass was dried to the moisture content of 5–10% (w/w). The sugars were analyzed by HPLC (HPLC 1260 Infinity; Agilent Technologies, CA, United States) equipped with an Aminex HPX-87P carbohydrate analysis column (Bio-Rad Laboratories, CA, United States) and a RI detector at 85°C with HPLC grade water as the mobile phase at a flow rate of 0.6 mL/min. The composition analysis was done in three replicates. For all significance tests, a student t.test was used, requiring a probability p-value < 0.05 to be significant.
Results
Chemical Composition Changes in the Solid Fraction
The pretreatment conditions have a significant impact on the chemical component in biomass and thus further conversion performance. The chemical composition changes in the solid fraction from different pretreatment were first analyzed. As shown in Figure 1A, for the hemicellulose, there were no significant changes in pretreated biomass composition after only dioxane treatment (organosolv) or combined dioxane and Fenton reaction treatment (organosolv and Fenton) as compared to the untreated biomass. However, when the switchgrass was treated with formic acid and dioxane, the hemicellulose content reduced to about 6.38%, indicating that the hemicellulose was almost removed from switchgrass biomass (Figure 1A). A slightly more efficient hemicellulose removal can be achieved with complete F2O pretreatment comparting to only the formic acid and dioxane treated group.
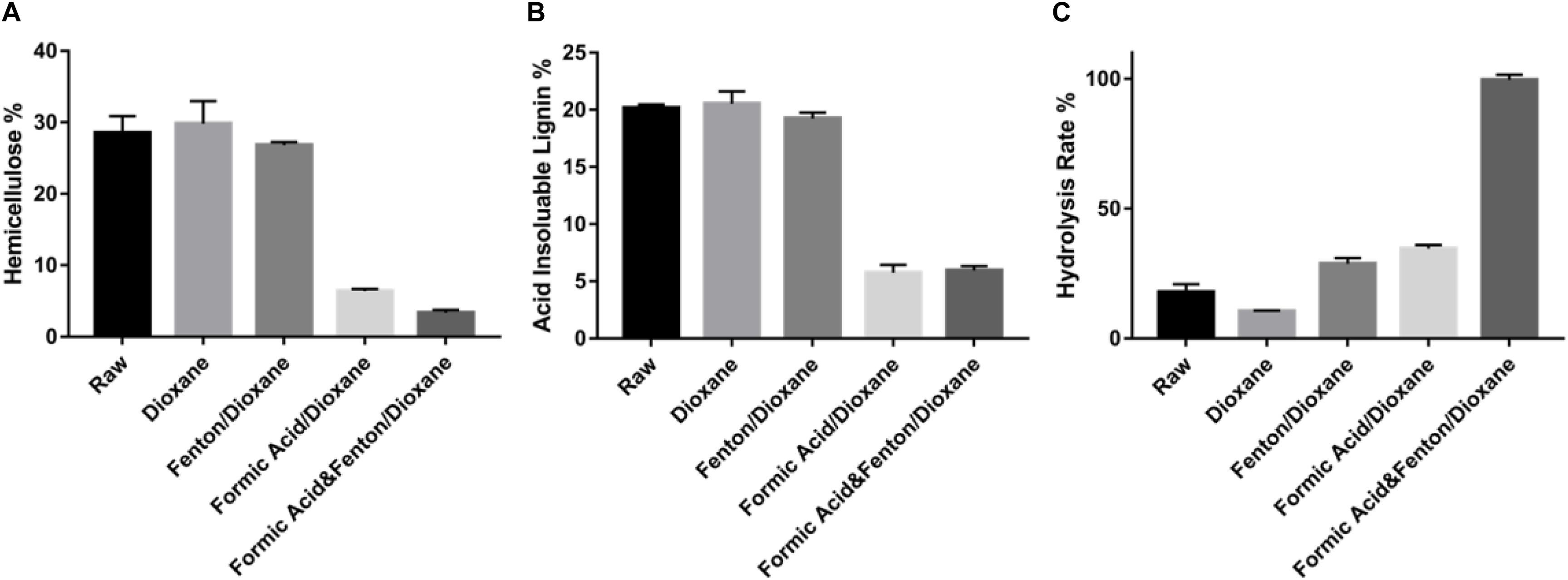
Figure 1. Removal of lignin and hemicellulose after different pretreatment methods. (A) hemicellulose; (B) acid insoluble lignin; (C) hydrolysis performance by different pretreatment method. The error bars in the hemicellulose and hemicellulose composition analysis represent the standard deviation from three replicates. The paired t test showed a significant difference between F2O and Raw with a p-value < 0.05 in the composition of hemicellulose, acid insoluble lignin, and hydrolysis rate.
As for lignin, the results highlighted the same pattern as the hemicellulose (Figure 1B). Efficient lignin removal was achieved when the switchgrass was pretreated with formic acid and then extracted with dioxane, with the solid fraction containing only about 5% lignin (Figure 1B and Supplementary Figure 2). Formic acid has been reported as an organosolv pretreatment to remove 90% hemicellulose and 70% lignin from corn cob feedstock through an 8 h reaction at 60°C (Huang et al., 2008). In the present study, the F2O process removed more than 88% of the hemicellulose and 71% of the lignin. Overall, the combination of formic acid with organosolv pretreatment with or without Fenton reagents can effectively remove most of hemicellulose and lignin and enrich the cellulose content in the solid fraction. It is critical to evaluate the hydrolysis efficiency of the solid fraction to determine the most effective pretreatment conditions.
Fermentable Sugar Released From the Solid Fraction
The enzymatic hydrolysis performance of the pretreated biomass was evaluated as shown in Figure 1C. Compared to the untreated biomass, the dioxane-only treated or Fenton-dioxane treated biomass showed a very ineffective sugar release. The poor performance of enzymatic hydrolysis could be caused by the recalcitrance of lignocellulosic biomass, which indicates that the Fenton reagent alone is not sufficient to release the cellulose and hemicellulose from the lignin. Interestingly, the glucan conversion of biomass treated with F2O is two-fold higher than that treated with Formic acid-dioxane even though their compositions were very similar, as mentioned earlier (Figure 1C). Previous studies confirmed that formic acid could cause cellulose to formulate, which decreases the enzyme hydrolysis (Zhao et al., 2009). The higher enzymatic hydrolysis efficiency of F2O pretreated switchgrass indicates that radicals produced from the Fenton reagent might play an essential role in reducing the crystallization of cellulose and the inhibition of cellulose formylation, which increased the processability of the cellulose by enzymes. Moreover, enzymatic hydrolysis is also related to the cellulose crystallinity, the degree of polymerization, and accessible surface area by enzyme (Zhu et al., 2008). The efficient radicals from the reaction between formic acid and Fenton reagents could have impacted these factors, thus enhancing the performance of enzymatic hydrolysis. Besides glucose release, F2O pretreatment degraded most of the hemicellulose (Supplementary Figure 2). As previously reported, in pretreatment the hemicellulose can release into xylose and may be further degraded to furfural. Formic acid may be formed when furfural are broken down under high pretreatment severity (Dunlop, 1948). It should be noted that the hemicellulose-derived compounds did not seem to inhibit cellulose hydrolysis, considering the high hydrolysis efficiency.
Lipid Fermentation Using F2O Switchgrass Treated Lignin Residue as a Carbon Source by R. opacus PD630
Rhodococcus opacus could accumulate lipid using suitable lignin as substrates. Moreover, R. opacus can tolerate inhibitory compounds from lignocellulosic biomass depolymerization and degradation such as furfural and phenolics (Kurosawa et al., 2015). To evaluate the efficacy of co-optimization of both carbohydrate and lignin processability, bioconversion of lignin residues from F2O pretreatment was carried out to produce lipids using R. opacus. As shown in Supplementary Table 2, by the fed batch fermentation, 6.02 g/L cell dry biomass and 1.16 g/L lipid were achieved, while 19.20% lipid content (per cell dry weight) was obtained. As reported by others studies, the lipid yield was 0.5 g/L by alkali-extracted lignin (He Y. et al., 2017), 72 mg/L by Kraft lignin (Mycroft et al., 2015), and 32 mg/L by AFEX lignin (Wang et al., 2019) through R. opacus fermentation. The F2O lignin from the F2O process yielded 1.16 g/L lipid which suggested easier processability of lignin by microorganisms, as reflected in the study on from natural white-rot fungi which originally inspired this examination of biomass utilization. This higher lipid yield suggests that the F2O pretreatment process delivered an easier digestibility of lignin for microorganism utilization. Overall, F2O pretreatment has co-optimized both lignin and carbohydrate processability and the efficient lignin conversion could be due to the effective lignin depolymerization by F2O pretreatment (Figure 4).
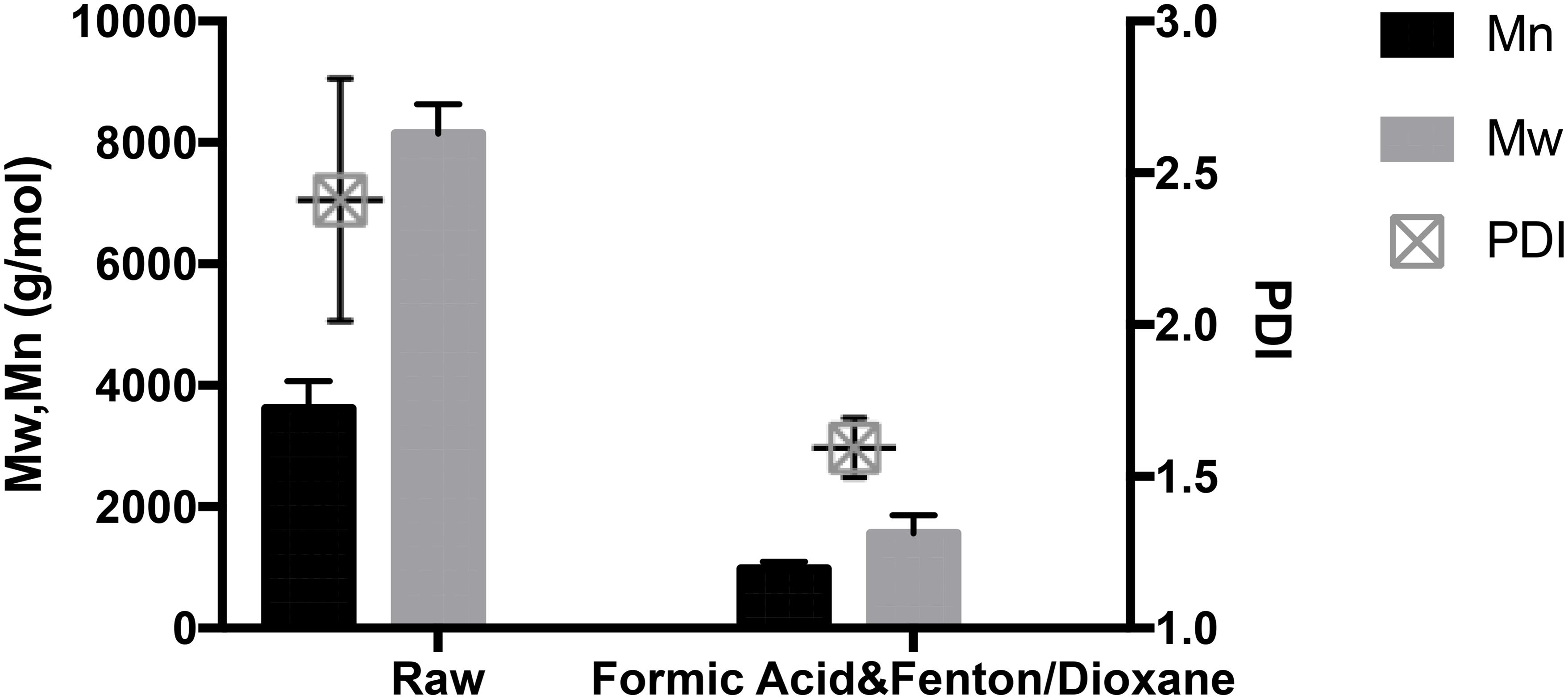
Figure 3. GPC reveals the lignin depolymerization after F2O pretreatment. Error bars in the figures represented the standard deviation of three replicates.
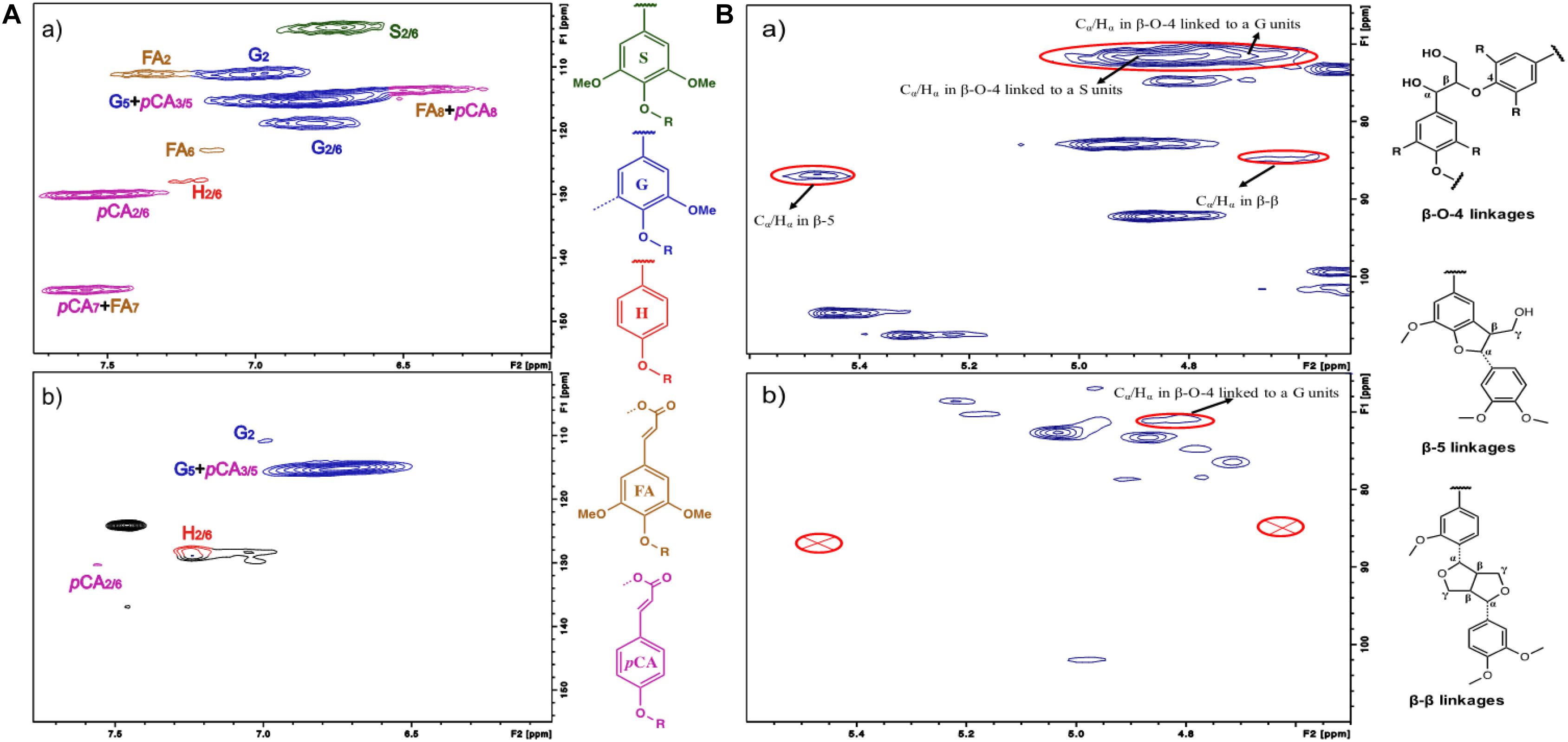
Figure 4. 2D HSQC NMR spectra for (a) the ball milled lignin from raw switchgrass and (b) lignin from F2O processed switchgrass. (A) Aromatic regions. S, syringyl; G, guaiacyl; H, p-hydroxyphenyl; FA, ferulate; pCA, p-coumarate. (B) Aliphatic regions.
Biomass Morphology Change After F2O Pretreatment
Field-emission scanning electron microscope was carried out to evaluate the deconstruction of switchgrass biomass by F2O pretreatment. Previous SEM analysis of formic acid treated sugarcane bagasse showed a rough surface and increased the enzyme accessibility (Sindhu et al., 2010). However, the cell wall of switchgrass treated by diluted acid did not show that obvious disruption compared to treated by the ionic liquid, which also showed lower hydrolysis rate and performance (Li et al., 2010). These results indicate that the sufficient disruption of the cell wall is essential for the next step in enzymatic hydrolysis. To evaluate the cell wall deconstruction, FE-SEM was carried out to compare the switchgrass before and after F2O pretreatment. As shown in Figure 2A, the raw switchgrass biomass before the F2O pretreatment was rod-like with smooth surfaces and suggested intact structures. After the F2O pretreatment, the fibrils in the biomass were found to be exposed toward the outer side (Figure 2B) which indicated that the biomass has been deconstructed with severe surface erosions. These visible changes in the biomass structure could be caused by removal of hemicellulose and lignin from the cell wall as well as the release of cellulose fiber during the pretreatment (Jönsson and Martín, 2016). The deconstructed structure after pretreatment could result in an increase in the surface area, which could contribute to the enhanced enzymatic hydrolysis of pretreated solids (Kumar and Sharma, 2017).
Lignin Molecular Weight Decrease After F2O Pretreatment
The changes in lignin molecular weight can both elucidate the biomass deconstruction mechanisms and be indicative of the residue’s capacity in bioconversion. Considering that the microorganism could effectively utilize smaller lignin molecules, effective lignin fractionation plays an important role in the lignin fermentation performance. First, the molecular weight from the lignin fraction after F2O pretreatment was measured. As shown in Figure 3, the CEL isolated from raw switchgrass feedstock showed Mn of 3617.9 g/mol and Mw of 8142.7 g/mol. However, lignin collected from F2O pretreatment had a number-average molecular weight (Mn) of 977.3 g/mol and weight-average molecular weight (Mw) of 1564.8 g/mol. The significantly decreased molecular weight confirmed that F2O treatment can effectively depolymerize lignin from switchgrass. Such efficient depolymerization contributed to the improved hydrolysis efficiency and also enhanced lignin processability. Correspondently, lignin from F2O pretreatment also has a decreased polydispersity index 1.595 as compared to that of CEL 2.275, which indicated a more uniform lignin fraction (Figure 3). Detailed information from the GPC chromatograph (Supplementary Figure 1) indicated that lignin residue collected from F2O pretreatment has lower molecular weight fractions, which was consistent with the improved lignin bioconversion. The changes in molecular weight provide important insights into lignin fragmentation. Previous organosolv pretreatment showed high purity and low molecular weight sulfur-free lignin (Zhao et al., 2009), which would provide high quality lignin for next step engineering. Therefore, the lignin obtained from F2O has a low molecular weight, which could be used as the optimal substrates for bioconversion and would be used as a potential substrate for lignin valorization to other products.
NMR Analysis Revealed Efficient Depolymerization of Lignin After F2O Pretreatment
2D HSQC NMR analysis was carried out to evaluate the breakage of lignin interunitary linkages and changes in lignin composition. Figure 4A showed the aromatic regions of the HSQC NMR spectra including lignin subunits and hydroxycinnamates. C-H signals in syringyl unit (S2/6), guaiacyl unit (G2), ρ-hydroxyphenyl unit (H2/6), ferulate (FA2), and ρ-coumarate (pCA2/6) observed at δC/δH 103.8/6.70 ppm, δC/δH 110.9/6.95 ppm, δC/δH 127.8/7.16 ppm, δC/δH 111.2/7.28 ppm, and δC/δH 130.1/7.46 ppm, respectively, were used for the semi-quantitative analysis. The semi-quantitative result of each aromatic unit and inter-unit linkages are presented in Supplementary Table 3. Fractionated lignin from switchgrass by F2O pretreatment had a lower S/G ratio (0.11) as compared to that of CEL from untreated switchgrass (S/G ratio 0.45). The contents of S units were reduced by F2O pretreatment, while the content of the H unit was increased after the pretreatment. This result could be because the H units have higher β-O-4 linkages and more easily cleaved during pretreatment, and the small molecular weight molecules are extracted to the solid fraction after first step pretreatment. The increased H- type lignin could facilitate lignin consumption and thus promote the lipid formation. A previous study has reported that the strong oxidation activity of reactive radicals means they can decompose the phenolate group (He W. et al., 2017). The opening of the benzene ring within lignin would also be a reasonable way of decreasing S units. The lignin in the pretreated solid residues had lower pCA units than the CEL of switchgrass. As pCA is involved in lignification, it has links within lignin monomers and the formation of lignin-carbohydrate complexes (Wang et al., 2013). The lower pCA in F2O treated switchgrass confirmed the biomass fractionation.
Besides the aromatic units, lignin inter-unit linkages were observed in the aliphatic HSQC regions. Major lignin inter-unit linkages including β-O-4, β-5, and β-β were shown in Figure 4B. The three inter-unit linkages (β-O-4, β-5, and β-β) were all largely reduced by the F2O pretreatment. It indicated that F2O pretreatment significantly decomposed the lignin structures by cleaving these linkages. Overall, the effective linkage cleavage accounted for efficient lignin depolymerization and co-optimized carbohydrate and lignin processability. The increased H-unit lignin, the fractionation of pCA, and the cleavage of lignin interlinkages could contribute to the improved processability of lignin and thus improved lipid fermentation performance.
31P-NMR Analysis Revealed Efficient Deconstruction of Lignin After F2O Pretreatment
The contents of the hydroxyl group in each lignin fraction were determined by lignin phosphitylation followed by 31P NMR analysis. Supplementary Table 4 shows the results of the hydroxyl groups in the F2O fractionated lignin and the CEL lignin from the raw switchgrass. The chemical shift regions of the aliphatic OH, C5 substituted phenolic OH, guaiacyl OH, p-hydroxy phenyl OH, and acid OH were assigned at 150.0–145.4, 140.0–141.5, 141.0–139.2, 139.2–138.0, and 136.5–134.0 ppm, respectively (Pu et al., 2011). As presented in Supplementary Table 4, the F2O pretreatment significantly reduced the aliphatic OH groups in the lignin residue from F2O pretreatment, compared with the 4.18 mmol/g aliphatic OH in the control native lignin. Lower aliphatic OH content would increase the cellulase adsorption affinity and binding strength to lignin (Li et al., 2016), which is important during the enzymatic hydrolysis. Guaiacyl and p-hydroxyphenyl hydroxyl groups were largely reduced, while the C5 substituted phenolic hydroxyl groups remained constant. In most studies, the reduced aliphatic OH content is accompanied by an increased phenolic hydroxyl group (Li et al., 2016). However, the phenolic hydroxyl group decreased after F2O pretreatment (0.50 mmol/g) compared to the raw switchgrass (1.15 mmol/g). As mentioned before, the decreased phenolic hydroxyl could be caused by oxidation of phenoxy groups which is consistent with the significant decrease of S units. These changes also indicated the depolymerization and destruction of lignin in switchgrass and account for the improved lignin processability for bioconversion by R. opacus.
Discussion
By mimicking the natural lignocellulosic biomass degradation of white-rot fungi, the present study developed a two-step organosolv pretreatment (F2O) to achieve high processability of both carbohydrates and lignin. Although biological processing is used as an effective approach for lignocellulosic biomass utilization, it takes a long time with ambient temperature, reducing the pretreatment efficiency. To improve both efficiency and reaction rate, we mimicked the mechanism of natural lignocellulosic biomass degradation by white-rot fungi in a way that stronger reagents and conditions were employed to enhance the processability of biomass within a short period. These reagents can be recovered and reused, which will reduce processing costs.
The chemical composition, enzymatic hydrolysis, and lignin conversion analyses were conducted to evaluate the performance of the F2O process in a holistic way. The carbohydrate yield in enzymatic hydrolysis is affected by the lignin content in pretreated solid, and the accessibility of enzymes to the carbohydrate (Palonen et al., 2004). Acid insoluble lignin (AIL) has been confirmed as a major inhibitor during the enzymatic hydrolysis process (Zhai et al., 2018). After F2O pretreatment, the AIL was almost entirely removed, enabling improved enzymatic hydrolysis efficiency. Moreover, by mimicking the mechanism of natural lignocellulosic biomass degradation of white-rot fungi, the radicals produced during the pretreatment, such as HOO⋅ and ⋅COOH, could account for the improved deconstruction of switchgrass fiber and increased porosity for better accessibility of the enzyme, which would further enhance the performance of enzymatic hydrolysis.
Lignin is considered an important renewable carbon source for bioconversion into valuable bioproducts. However, the complexity of lignin polymers and the inhibitors produced in pretreatment makes it difficult for microorganisms to utilize (Kumar et al., 2009). Biomimicking the natural degradation process could be an effective approach to enhance the lignin processability by tuning the lignin chemistry. Using F2O treated lignin, efficient conversion from lignin to lipid were achieved by fermentation using R. opacus PD630. The mechanisms for improved lignin processability were illustrated through GPC and NMR analysis. Results showed that F2O produced lignin with a decreased molecular weight and improved uniformity in molecular weight distribution, suggesting efficient depolymerization of lignin by breaking the linkages. Further 31P NMR confirmed the breakdown of lignin polymers. Because aliphatic and phenolic OH content indicate the presence of condensed aromatic units (El Hage et al., 2009; Pu et al., 2011), F2O pretreated lignin showed a decreased OH content, indicating efficient lignin deconstruction. 2D NMR characterized the structural changes of the F2O pretreated lignin. The breakdown of β-O-4 linkages and decreased content of S unit confirm the efficient lignin degradation, which is essential for improved bioconversion.
Overall, by mimicking the biomass degradation mechanism of white-rot fungi, the present study provided a new path to enhance both the carbohydrates and lignin processability by deconstructing the lignin-carbohydrate complex and depolymerizing lignin. F2O pretreatment could be further optimized by fine-tuning reaction conditions and reagent usage to achieve complete utilization of carbohydrate and lignin. Considering the more uniform lignin structure and the fractionation, the lignin from the F2O process could also be used to manufacture carbon fiber, asphalt binder modifier, lignin nanoparticle, and other value-added materials (Li et al., 2017; Xie et al., 2017a; Liu et al., 2019a). This biomimicking strategy could have broad applicability for future research into the bioconversion of lignocellulosic biomass to bioproducts.
Data Availability Statement
All datasets generated for this study are included in the article/Supplementary Material.
Author Contributions
ML, SX, and JY conceived, designed, and drafted the manuscript. ML, NJH, QL, SB, SS, and MLO performed the experiment and collected the data. ML wrote the manuscript. SX, Z-HL, and JY revised this manuscript. All authors read and approved the final manuscript.
Funding
This work was financially supported by the U.S. DOE (Department of Energy), EERE (Energy Efficiency and Renewable Energy), BETO (Bioenergy Technology Office) (Grant Nos. DE-EE000612 and DE-EE0007104 to JY). This research was also supported by the Texas A&M Agrilife Research’s biofuel initiative (JY).
Conflict of Interest
The authors declare that the research was conducted in the absence of any commercial or financial relationships that could be construed as a potential conflict of interest.
Supplementary Material
The Supplementary Material for this article can be found online at: https://www.frontiersin.org/articles/10.3389/fenrg.2020.00194/full#supplementary-material
Abbreviations
F2O, Formic Acid: two-step Fenton:Organosolv; NMR, nuclear magnetic resonance; FE-SEM, field-emission scanning electron microscope; AFEX, ammonia fiber expansion; DDG, dried distillers’ grains; 2D-HSQC, 2D-heteronuclear single quantum coherence; NBUS, natural biomass utilization systems; TSB, tryptic soy broth; TMDP, 2-chloro-4,4,5,5-tetramethyl-1,3,2-dioxaphospholane; GPC, gel permeation chromatography; RI, refractive index; THF, tetrahydrofuran; CEL, cellulolytic enzyme lignin; AIL, acid insoluble lignin; ASL, acid soluble lignin; S2/6, syringyl unit; G2, guaiacyl unit; H2/6, ρ-hydroxyphenyl unit; FA2, ferulate; pCA2/6, ρ-coumarate.
References
Abdelaziz, O. Y., Brink, D. P., Prothmann, J., Ravi, K., Sun, M., García-Hidalgo, J., et al. (2016). Biological valorization of low molecular weight lignin. Biotechnol. Adv. 34, 1318–1346. doi: 10.1016/j.biotechadv.2016.10.001
Alonso, D. M., Wettstein, S. G., Mellmer, M. A., Gurbuz, E. I., and Dumesic, J. A. (2013). Integrated conversion of hemicellulose and cellulose from lignocellulosic biomass. Energy Environ. Sci. 6, 76–80. doi: 10.1039/c2ee23617f
Balch, M. L., Holwerda, E. K., Davis, M. F., Sykes, R. W., Happs, R. M., Kumar, R., et al. (2017). Lignocellulose fermentation and residual solids characterization for senescent switchgrass fermentation by Clostridium thermocellum in the presence and absence of continuous in situ ball-milling. Energy Environ. Sci. 10, 1252–1261. doi: 10.1039/c6ee03748h
Barr, D. P., and Aust, S. D. (1994). Mechanisms white rot fungi use to degrade pollutants. Environ. Sci. Technol. 28, 78A–87A.
Davies, S. M., Linforth, R. S., Wilkinson, S. J., Smart, K. A., and Cook, D. J. (2011). Rapid analysis of formic acid, acetic acid, and furfural in pretreated wheat straw hydrolysates and ethanol in a bioethanol fermentation using atmospheric pressure chemical ionisation mass spectrometry. Biotechnol. Biofuels 4:28. doi: 10.1186/1754-6834-4-28
Dunlop, A. P. (1948). Furfural formation and behavior. Industr. Engin. Chem. 40, 204–209. doi: 10.1021/ie50458a006
El Hage, R., Brosse, N., Chrusciel, L., Sanchez, C., Sannigrahi, P., and Ragauskas, A. (2009). Characterization of milled wood lignin and ethanol organosolv lignin from miscanthus. Polym. Degradat. Stabil. 94, 1632–1638. doi: 10.1016/j.polymdegradstab.2009.07.007
Feng, Y., Li, G., Li, X., Zhu, N., Xiao, B., Li, J., et al. (2016). Enhancement of biomass conversion in catalytic fast pyrolysis by microwave-assisted formic acid pretreatment. Bioresour. Technol. 214, 520–527. doi: 10.1016/j.biortech.2016.04.137
Hao, N., Lu, K., Ben, H., Adhikari, S., Lacerda, T. B., and Ragauskas, A. J. (2018). Effect of autohydrolysis pretreatment conditions on sugarcane bagasse structures and product distribution resulting from pyrolysis. Energy Technol. 6, 640–648. doi: 10.1002/ente.201700490
He, W., Gao, W., and Fatehi, P. (2017). Oxidation of Kraft lignin with hydrogen peroxide and its application as a dispersant for kaolin suspensions. ACS Sustainab. Chem. Engin. 5, 10597–10605.
He, Y., Li, X., Ben, H., Xue, X., and Yang, B. (2017). Lipid production from dilute alkali corn stover lignin by rhodococcus strains. ACS Sustainab. Chem. Engin. 5, 2302–2311. doi: 10.1021/acssuschemeng.6b02627
Himmel, M. E., Ding, S.-Y., Johnson, D. K., Adney, W. S., Nimlos, M. R., Brady, J. W., et al. (2007). Biomass recalcitrance: Engineering plants and enzymes for biofuels production. Science 315, 804–807. doi: 10.1126/science.1137016
Hu, F., and Ragauskas, A. (2012). Pretreatment and lignocellulosic chemistry. Bio Energy Res. 5, 1043–1066. doi: 10.1007/s12155-012-9208-0
Huang, R. L., Su, R., Qi, W., Zhang, M. J., and He, Z. M. (2008). Fractionation of lignocellulose by formic acid pretreatment. Biotech 8:221.
Isikgor, F. H., and Becer, C. R. (2015). Lignocellulosic biomass: a sustainable platform for the production of bio-based chemicals and polymers. Polym. Chem. 6, 4497–4559. doi: 10.1039/c5py00263j
Jin, M., Gunawan, C., Uppugundla, N., Balan, V., and Dale, B. E. (2012). A novel integrated biological process for cellulosic ethanol production featuring high ethanol productivity, enzyme recycling and yeast cells reuse. Energy Environ. Sci. 5, 7168–7175. doi: 10.1039/c2ee03058f
Johnson, C. W., and Beckham, G. T. (2015). Aromatic catabolic pathway selection for optimal production of pyruvate and lactate from lignin. Metabol. Engin. 28, 240–247. doi: 10.1016/j.ymben.2015.01.005
Jönsson, L. J., and Martín, C. (2016). Pretreatment of lignocellulose: Formation of inhibitory by-products and strategies for minimizing their effects. Bioresour. Technol. 199, 103–112. doi: 10.1016/j.biortech.2015.10.009
Jung, Y. H., Kim, H. K., Park, H. M., Park, Y.-C., Park, K., Seo, J.-H., et al. (2015). Mimicking the Fenton reaction-induced wood decay by fungi for pretreatment of lignocellulose. Bioresour. Technol. 179, 467–472. doi: 10.1016/j.biortech.2014.12.069
Kameshwar, A. K. S., and Qin, W. (2018). Molecular networks of postia placenta involved in degradation of lignocellulosic biomass revealed from metadata analysis of open access gene expression data. Int. J. Biol. Sci. 14, 237–252. doi: 10.7150/ijbs.22868
Kim, J. S., Lee, Y. Y., and Kim, T. H. (2016). A review on alkaline pretreatment technology for bioconversion of lignocellulosic biomass. Bioresour. Technol. 199, 42–48. doi: 10.1016/j.biortech.2015.08.085
Kumar, A. K., and Sharma, S. (2017). Recent updates on different methods of pretreatment of lignocellulosic feedstocks: a review. Bioresour. Bioproc. 4:7.
Kumar, P., Barrett, D. M., Delwiche, M. J., and Stroeve, P. (2009). Methods for pretreatment of lignocellulosic biomass for efficient hydrolysis and biofuel production. Industr. Engin. Chem. Res. 48, 3713–3729. doi: 10.1021/ie801542g
Kurosawa, K., Laser, J., and Sinskey, A. J. (2015). Tolerance and adaptive evolution of triacylglycerol-producing Rhodococcus opacus to lignocellulose-derived inhibitors. Biotechnol. Biofu. 8:76.
Lau, M. W., Gunawan, C., and Dale, B. E. (2009). The impacts of pretreatment on the fermentability of pretreated lignocellulosic biomass: a comparative evaluation between ammonia fiber expansion and dilute acid pretreatment. Biotechnol. Biofu. 2:30. doi: 10.1186/1754-6834-2-30
Lee, S., Kang, M., Bae, J.-H., Sohn, J.-H., and Sung, B. H. (2019). Bacterial valorization of lignin: Strains, enzymes, conversion pathways, biosensors, and perspectives. Front. Bioengin. Biotechnol. 7:209. doi: 10.3389/fbioe.2019.00209
Li, C., Knierim, B., Manisseri, C., Arora, R., Scheller, H. V., Auer, M., et al. (2010). Comparison of dilute acid and ionic liquid pretreatment of switchgrass: Biomass recalcitrance, delignification and enzymatic saccharification. Bioresour. Technol. 101, 4900–4906. doi: 10.1016/j.biortech.2009.10.066
Li, M., Pu, Y., and Ragauskas, A. J. (2016). Current understanding of the correlation of lignin structure with biomass recalcitrance. Front. Chem. 4:45. doi: 10.3389/fchem.2016.00045
Li, Q., Xie, S., Serem, W. K., Naik, M. T., Liu, L., and Yuan, J. S. (2017). Quality carbon fibers from fractionated lignin. Gr. Chem. 19, 1628–1634. doi: 10.1039/c6gc03555h
Lima, M. A., Lavorente, G. B., Da Silva, H. K., Bragatto, J., Rezende, C. A., Bernardinelli, O. D., et al. (2013). Effects of pretreatment on morphology, chemical composition and enzymatic digestibility of eucalyptus bark: a potentially valuable source of fermentable sugars for biofuel production - part 1. Biotechnol. Biofu. 6:75. doi: 10.1186/1754-6834-6-75
Liu, Z.-H., Hao, N., Shinde, S., Olson, M. L., Bhagia, S., Dunlap, J. R., et al. (2018). Codesign of combinatorial organosolv pretreatment (COP) and lignin nanoparticles (LNPs) in biorefineries. America: ACS Sustainable Chemistry & Engineering.
Liu, Z.-H., Hao, N., Shinde, S., Pu, Y., Kang, X., Ragauskas, A. J., et al. (2019a). Defining lignin nanoparticle properties through tailored lignin reactivity by sequential organosolv fragmentation approach (SOFA). United Kingdom: Green Chemistry.
Liu, Z.-H., Shinde, S., Xie, S., Hao, N., Lin, F., Li, M., et al. (2019b). Cooperative valorization of lignin and residual sugar to polyhydroxyalkanoate (PHA) for enhanced yield and carbon utilization in biorefineries. Sustainab. Energy Fuels 3, 2024–2037. doi: 10.1039/c9se00021f
Matsakas, L., Nitsos, C., Raghavendran, V., Yakimenko, O., Persson, G., Olsson, E., et al. (2018). A novel hybrid organosolv: steam explosion method for the efficient fractionation and pretreatment of birch biomass. Biotechnol. Biofu. 11:160.
Mycroft, Z., Gomis, M., Mines, P., Law, P., and Bugg, T. D. H. (2015). Biocatalytic conversion of lignin to aromatic dicarboxylic acids in Rhodococcus jostii RHA1 by re-routing aromatic degradation pathways. Gr. Chem. 17, 4974–4979. doi: 10.1039/c5gc01347j
Ninomiya, K., Takamatsu, H., Onishi, A., Takahashi, K., and Shimizu, N. (2013). Sonocatalytic–Fenton reaction for enhanced OH radical generation and its application to lignin degradation. Ultrason. Sonochem. 20, 1092–1097. doi: 10.1016/j.ultsonch.2013.01.007
Palonen, H., Tjerneld, F., Zacchi, G., and Tenkanen, M. (2004). Adsorption of Trichoderma reesei CBH I and EG II and their catalytic domains on steam pretreated softwood and isolated lignin. J. Biotechnol. 107, 65–72. doi: 10.1016/j.jbiotec.2003.09.011
Pothiraj, C., Kanmani, P., and Balaji, P. (2006). Bioconversion of lignocellulose materials. Mycobiology 34, 159–165. doi: 10.4489/myco.2006.34.4.159
Pu, Y., Cao, S., and Ragauskas, A. J. (2011). Application of quantitative 31P NMR in biomass lignin and biofuel precursors characterization. Energy Environ. Sci. 4, 3154–3166. doi: 10.1039/c1ee01201k
Qin, X., Su, X., Luo, H., Ma, R., Yao, B., and Ma, F. (2018). Deciphering lignocellulose deconstruction by the white rot fungus Irpex lacteus based on genomic and transcriptomic analyses. Biotechnol. Biofu. 11:58.
Quinlan, R. J., Sweeney, M. D., Lo Leggio, L., Otten, H., Poulsen, J.-C. N., Johansen, K. S., et al. (2011). Insights into the oxidative degradation of cellulose by a copper metalloenzyme that exploits biomass components. Proc. Nat. Acad. Sci. 108, 15079–15084.
Rouches, E., Herpoël-Gimbert, I., Steyer, J. P., and Carrere, H. (2016). Improvement of anaerobic degradation by white-rot fungi pretreatment of lignocellulosic biomass: A review. Renew. Sustainab. Energy Rev. 59, 179–198. doi: 10.1016/j.rser.2015.12.317
Rudakiya, D. M., and Gupte, A. (2017). Degradation of hardwoods by treatment of white rot fungi and its pyrolysis kinetics studies. Int. Biodeteriorat. Biodegrad. 120, 21–35. doi: 10.1016/j.ibiod.2017.02.004
Saha, M., Saynik, P. B., Borah, A., Malani, R. S., Arya, P., and Moholkar, V. S. (2019). Dioxane-based extraction process for production of high quality lignin. Bioresour. Technol. Rep. 5, 206–211. doi: 10.1016/j.biteb.2019.01.018
Selig, M., Weiss, N., and Ji, Y. (2008). Enzymatic saccharification of lignocellulosic biomass. America: Laboratory Analytical rocedure (LA).
Sindhu, R., Binod, P., Satyanagalakshmi, K., Janu, K. U., Sajna, K. V., Kurien, N., et al. (2010). Formic Acid as a potential pretreatment agent for the conversion of sugarcane bagasse to bioethanol. Appl. Biochem. Biotechnol. 162, 2313–2323. doi: 10.1007/s12010-010-9004-2
Sluiter, B. H. A., Ruiz, R., Scarlata, C., Sluiter, J., Templeton, D., and Crocker, D. (2012). Determination of structural carbohydrates and lignin in biomass. Colorado: National Renewable Energy Laboratory.
Sun, S., Xie, S., Chen, H., Cheng, Y., Shi, Y., Qin, X., et al. (2016). Genomic and molecular mechanisms for efficient biodegradation of aromatic dye. J. Hazard. Mater. 302, 286–295. doi: 10.1016/j.jhazmat.2015.09.071
Sun, S., Xie, S., Cheng, Y., Yu, H., Zhao, H., Li, M., et al. (2017). Enhancement of environmental hazard degradation in the presence of pignin: a proteomics study. Sci. Rep. 7:11356.
Vel Leitner, N. K., and Dore, M. (1996). Hydroxyl radical induced decomposition of aliphatic acids in oxygenated and deoxygenated aqueous solutions. J. Photochem. Photobiol. A Chem. 99, 137–143. doi: 10.1016/s1010-6030(96)04360-2
Wang, S., Zhao, W., Lee, T. S., Singer, S. W., Simmons, B. A., Singh, S., et al. (2018). Dimethyl sulfoxide assisted ionic liquid pretreatment of switchgrass for isoprenol production. ACS Sustainab. Chem. Engin. 6, 4354–4361. doi: 10.1021/acssuschemeng.7b04908
Wang, Y., Chantreau, M., Sibout, R., and Hawkins, S. (2013). Plant cell wall lignification and monolignol metabolism. Front. Plant Sci. 4:220. doi: 10.3389/fpls.2013.00220
Wang, Z., Li, N., and Pan, X. (2019). Transformation of ammonia fiber expansion (AFEX) corn stover lignin into microbial lipids by Rhodococcus opacus. Fuel 240, 119–125. doi: 10.1016/j.fuel.2018.11.081
Wei, H., Donohoe, B. S., Vinzant, T. B., Ciesielski, P. N., Wang, W., Gedvilas, L. M., et al. (2011). Elucidating the role of ferrous ion cocatalyst in enhancing dilute acid pretreatment of lignocellulosic biomass. Biotechnol. Biofu. 4:48. doi: 10.1186/1754-6834-4-48
Xie, S., Li, Q., Karki, P., Zhou, F., and Yuan, J. S. (2017a). Lignin as renewable and superior asphalt binder modifier. ACS Sustainab. Chem. Engin. 5, 2817–2823. doi: 10.1021/acssuschemeng.6b03064
Xie, S., Sun, Q., Pu, Y., Lin, F., Sun, S., Wang, X., et al. (2017b). Advanced chemical design for efficient lignin bioconversion. ACS Sustainab. Chem. Engin. 5, 2215–2223. doi: 10.1021/acssuschemeng.6b02401
Xie, S., Syrenne, R., Sun, S., and Yuan, J. S. (2014). Exploration of natural biomass utilization systems (NBUS) for advanced biofuel—from systems biology to synthetic design. Curr. Opin. Biotechnol. 27, 195–203. doi: 10.1016/j.copbio.2014.02.007
Xu, Z., Lei, P., Zhai, R., Wen, Z., and Jin, M. (2019). Recent advances in lignin valorization with bacterial cultures: microorganisms, metabolic pathways, and bio-products. Biotechnol. Biofu. 12:32.
Yoo, C. G., Yang, Y., Pu, Y., Meng, X., Muchero, W., Yee, K. L., et al. (2017). Insights of biomass recalcitrance in natural Populus trichocarpa variants for biomass conversion. Gr. Chem. 19, 5467–5478. doi: 10.1039/c7gc02219k
Yuan, J. S., Tiller, K. H., Al-Ahmad, H., Stewart, N. R., and Stewart, C. N. (2008). Plants to power: bioenergy to fuel the future. Trends Plant Sci. 13, 421–429. doi: 10.1016/j.tplants.2008.06.001
Yuan, J. S., Wang, X., and Stewart, C. N. (2011). Biomass feedstock: diversity as a solution. Biofuels 2, 491–493. doi: 10.4155/bfs.11.135
Zhai, R., Hu, J., and Saddler, J. N. (2018). Extent of enzyme inhibition by phenolics derived from pretreated biomass is significantly influenced by the size and carbonyl group content of the phenolics. ACS Sustainab. Chem. Engin. 6, 3823–3829. doi: 10.1021/acssuschemeng.7b04178
Zhang, K., Pei, Z., and Wang, D. (2016). Organic solvent pretreatment of lignocellulosic biomass for biofuels and biochemicals: A review. Bioresour. Technol. 199, 21–33. doi: 10.1016/j.biortech.2015.08.102
Zhang, Z., Harrison, M. D., Rackemann, D. W., Doherty, W. O. S., and O’hara, I. M. (2016). Organosolv pretreatment of plant biomass for enhanced enzymatic saccharification. Gr. Chem. 18, 360–381. doi: 10.1039/c5gc02034d
Zhao, X., Cheng, K., and Liu, D. (2009). Organosolv pretreatment of lignocellulosic biomass for enzymatic hydrolysis. Appl. Microbiol. Biotechnol. 82:815. doi: 10.1007/s00253-009-1883-1
Zhu, L., O’dwyer, J. P., Chang, V. S., Granda, C. B., and Holtzapple, M. T. (2008). Structural features affecting biomass enzymatic digestibility. Bioresour. Technol. 99, 3817–3828. doi: 10.1016/j.biortech.2007.07.033
Keywords: lignocellulosic biomass, lipid, organosolv, Fenton, formic acid, pretreatment, biomimicking processing
Citation: Li M, Liu Z-H, Hao N, Olson ML, Li Q, Bhagia S, Shinde S, Kao KC, Ragauskas AJ, Xie S and Yuan JS (2021) Synergistic Improvement of Carbohydrate and Lignin Processability by Biomimicking Biomass Processing. Front. Energy Res. 8:194. doi: 10.3389/fenrg.2020.00194
Received: 10 March 2020; Accepted: 22 July 2020;
Published: 04 February 2021.
Edited by:
Eulogio Castro, University of Jaén, SpainReviewed by:
Jalel Labidi, University of the Basque Country, SpainHéctor A. Ruiz, Universidad Autónoma de Coahuila, Mexico
Copyright © 2021 Li, Liu, Hao, Olson, Li, Bhagia, Shinde, Kao, Ragauskas, Xie and Yuan. This is an open-access article distributed under the terms of the Creative Commons Attribution License (CC BY). The use, distribution or reproduction in other forums is permitted, provided the original author(s) and the copyright owner(s) are credited and that the original publication in this journal is cited, in accordance with accepted academic practice. No use, distribution or reproduction is permitted which does not comply with these terms.
*Correspondence: Shangxian Xie, c2hhbmd4aWFuX3hpZUBodXN0LmVkdS5jbg==; Joshua S. Yuan, c3l1YW5AdGFtdS5lZHU=