- 1Research and Innovation Center on CO2 and H2, Khalifa University, Abu Dhabi, United Arab Emirates
- 2Chemical Engineering Department, Khalifa University, Abu Dhabi, United Arab Emirates
- 3Center for Catalysis and Separation, Khalifa University, Abu Dhabi, United Arab Emirates
- 4Process Engineering Department, EAFIT University, Medellin, Colombia
- 5Chemistry Department, Khalifa University, Abu Dhabi, United Arab Emirates
The possibilities offered by molecular modeling tools to obtain relevant data at process conditions, while also gaining molecular insights on the techniques used for CO2 capture and separation, are presented here using selected case studies. Two different technologies, absorption with amine-based systems and adsorption on porous materials, were explored, using the molecular-based equation of state, soft-Statistical Associating Fluid Theory (SAFT), and Grand Canonical Monte Carlo simulations, respectively. The aqueous monoethanolamine (MEA) system was set as the benchmark for absorption and compared to the performance of 8 alternative amine-based systems, while 16 adsorbents belonging to different families (zeolites, metal–organic frameworks, amorphous silicas, and activated carbons), bare or functionalized with alkylamines, were investigated for the separation of CO2 by adsorption. In addition to obtaining molecular information on the CO2 capture process, the models were further used to examine the CO2 capture performance in terms of cyclic working capacity and energy index as key performance indicators, allowing the identification of promising systems that can improve the current ones to be further evaluated for separation in non-power industries. Results show that for the same total amine mass concentration, non-aqueous amine solvents have a 5–10% reduction in cyclic working capacity, and a 10–30% decrease in the energy index compared to their aqueous counterparts due to their lower heat of vaporization and specific heat capacity. In addition, M-MOF-74, NaX, and NaY structures present the best results for adsorption in temperature swing adsorption (TSA) processes. Similar values of energy requirements to those of amine-based systems (2–2.5 MJ kg CO2–1) were obtained for some of the adsorbent; however, the disadvantage of the TSA process versus absorption should be considered. These results confirm the reliability of molecular modeling as an attractive and valuable screening tool for CO2 capture and separation processes.
Introduction
Since the nineteenth century, the planet’s average temperature has risen ∼0.9°C, a change driven largely by the increased emissions into the atmosphere of anthropogenic carbon dioxide and other greenhouse gases (GHGs; Santer et al., 2003; Sreedhar et al., 2017), as a consequence of the energy intensive and industrialized society in which we live. Among the different human activities, industry accounts today for ∼40% of the global energy demand and 25% of the total CO2 emissions, the second largest source of emissions after the power sector (International Energy Agency [IEA], 2019). Steel and cement are the two highest-emitting industry subsectors, accounting for more than 10% of the total direct global CO2 emissions: over 2.1 Gt of CO2 per year from each cement and iron/steel industries in the past few years (the chemical subsector is the third largest emitter with around 1.1 Gt/year; International Energy Agency [IEA], 2018).
Although efforts for shifting toward renewable energy sources and more energy-efficient systems are already in place, fossil fuels are likely to remain an important energy source, in the near and medium term (Le Quéré et al., 2019), due to their availability and efficiency. Hence, the CO2 level into the atmosphere, which has already drastically surpassed the safety boundaries (above 400 ppm; Steffen et al., 2015) will continue to increase in a business as usual scenario. Therefore, controlling such emissions is already a critical environmental issue, with scientists and regulators taking actions toward reducing GHG emissions and mitigating climate change (Intergovernmental Panel on Climate Change [IPCC], 2014; National Oceanic and Atmospheric Administration [NOAA], 2019). Significant work and effort have been employed in recent years for the development of CO2 capture technologies to limit the degree of future climate change (Asif et al., 2018) while using fossil fuels. One of the most important alternatives to mitigate anthropogenic CO2 emissions in the short–medium term is by carbon capture, utilization, and storage (CCUS; Wang et al., 2012). Nowadays, 16 large-scale CCUS applications at industrial facilities are capturing more than 30 million tons of CO2 emissions each year from steel production, natural gas processing, and hydrogen and fertilizer (ammonia) production (International Energy Agency [IEA], 2019). Nevertheless, the IEA Clean Technology Scenario, which sets out a pathway consistent with the Paris Agreement climate ambition, mentions that CCUS can contribute with 20% of the emissions reductions needed across the industry sector (i.e., more than 28 Gt of CO2 captured in the period to 2060). A further 31 Gt is expected to be captured from fuel transformation and 56 Gt from the power sector (International Energy Agency [IEA], 2018, 2019). Moreover, the letter “U” appearing in CCUS stands for utilization, as the captured CO2 can also be used in several industrial sectors and products, such as the carbonation of beverages, food preservation, supercritical CO2 extraction processes, enhanced oil recovery (EOR), synthesis of materials for different applications and water treatment, as well as the building block or raw source for other chemicals (Vega, 2010), making the CCUS an alternative, attractive complementary route to CO2 capture and storage.
Several technologies have been explored for CO2 capture and separation (Abanades et al., 2005; MacDowell et al., 2010). Among them, absorption with aqueous solutions of amines is technically proven and considered the most industrially feasible technology for carbon capture at a large scale (Asif et al., 2018; Vega et al., 2019). Monoethanolamine (MEA) is the most used alkanolamine molecule in CO2 capture processes due to its high affinity and reactivity with CO2 and relatively low costs of production. However, an important barrier to the full-scale deployment of any aqueous amine solvents is the high energy required for solvent regeneration, primarily related to the sensible heat of the cosolvent, water (Oyenekan and Rochelle, 2007; Svendsen et al., 2011; Budzianowski, 2015; Zhang et al., 2015; Li et al., 2016). The reduction in this energy penalty can be accomplished through several strategies such as process optimization, the use of different amines, and/or alternative solvents with lower regeneration energy requirements, the use of different techniques such as adsorption on microporous solid materials, among others (Huck et al., 2014; Budzianowski, 2016; Bernhardsen and Knuutila, 2017; Heldebrant et al., 2017). Although alternative absorbents (e.g., water-free and water-lean solvents) and adsorbent (e.g., metal–organic frameworks, MOFs) are quite promising, many of them are still in early stages of development, lacking in most cases required data for rigorous modeling, or large-scale projections with the current state of available modeling tools (Prats et al., 2017; Alkhatib et al., 2019). In this regard, with the improvement in computing power and new algorithms, computational methods have become an emblematic complementary tool to experiments (Smit and Maesen, 2008; Garcia et al., 2017). Appropriate and reliable thermodynamic models that can reproduce the physicochemical behavior of the systems, combined with simple engineering calculations, can provide an efficient framework to assess and screen the performance of these promising materials in terms of key performance indicators (KPIs) such as cyclic working capacities and energy index of regeneration (Bahamon and Vega, 2016; Alkhatib et al., 2019) and determine potential systems to be further explored in pilot and large facilities (Bai et al., 2015).
Herein, we present some examples concerning the use of molecular modeling techniques based on statistical mechanics (molecular-based equations of state and molecular simulations) for the modeling of alternative amine-based absorbents and porous solid adsorbents for CO2 capture and separation. A list of the systems evaluated is presented in Table 1, together with the CO2 concentration explored and the operating conditions, representative of CO2 emissions from different industrial sectors. Accurate and transferable models able to characterize the absorption and adsorption processes are used as a step forward in developing predictive models for these systems, aiming to find a balance between accuracy, transferability, and simplicity. Such treatments have been successfully implemented by our group and others in previous studies (Vega and Bahamon, 2016; Lloret et al., 2017; Bahamon et al., 2018; Pereira and Vega, 2018; Alkhatib et al., 2020). This work serves as a bridge between the fundamental knowledge on the molecular behavior of the substances/materials and how this is translated into their performance at industrial conditions. We illustrate how molecular-based equations of state and molecular simulations can be used as a robust tool for a double purpose: (1) to understand the underlying phenomena of CO2 capture in absorbent solutions and adsorbent materials and (2) to complement experimental data for process design at industrial conditions of relevance for CO2 capture. In Figure 1, we show a schematic of the followed approach, including the length scales covered by the modeling tools used in this work.
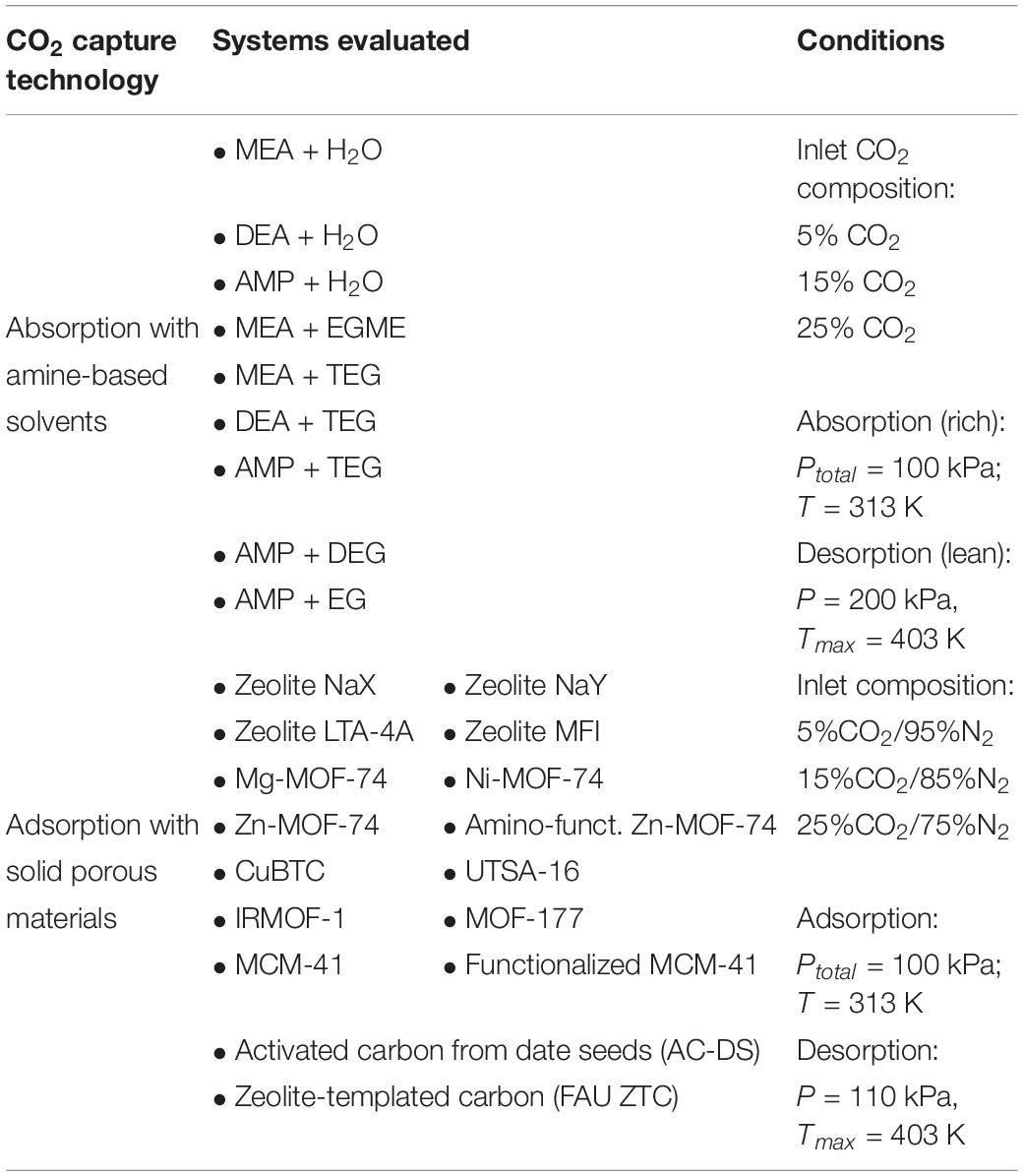
Table 1. Summary of the systems evaluated in this work for CO2 capture, together with the conditions at which they were evaluated.
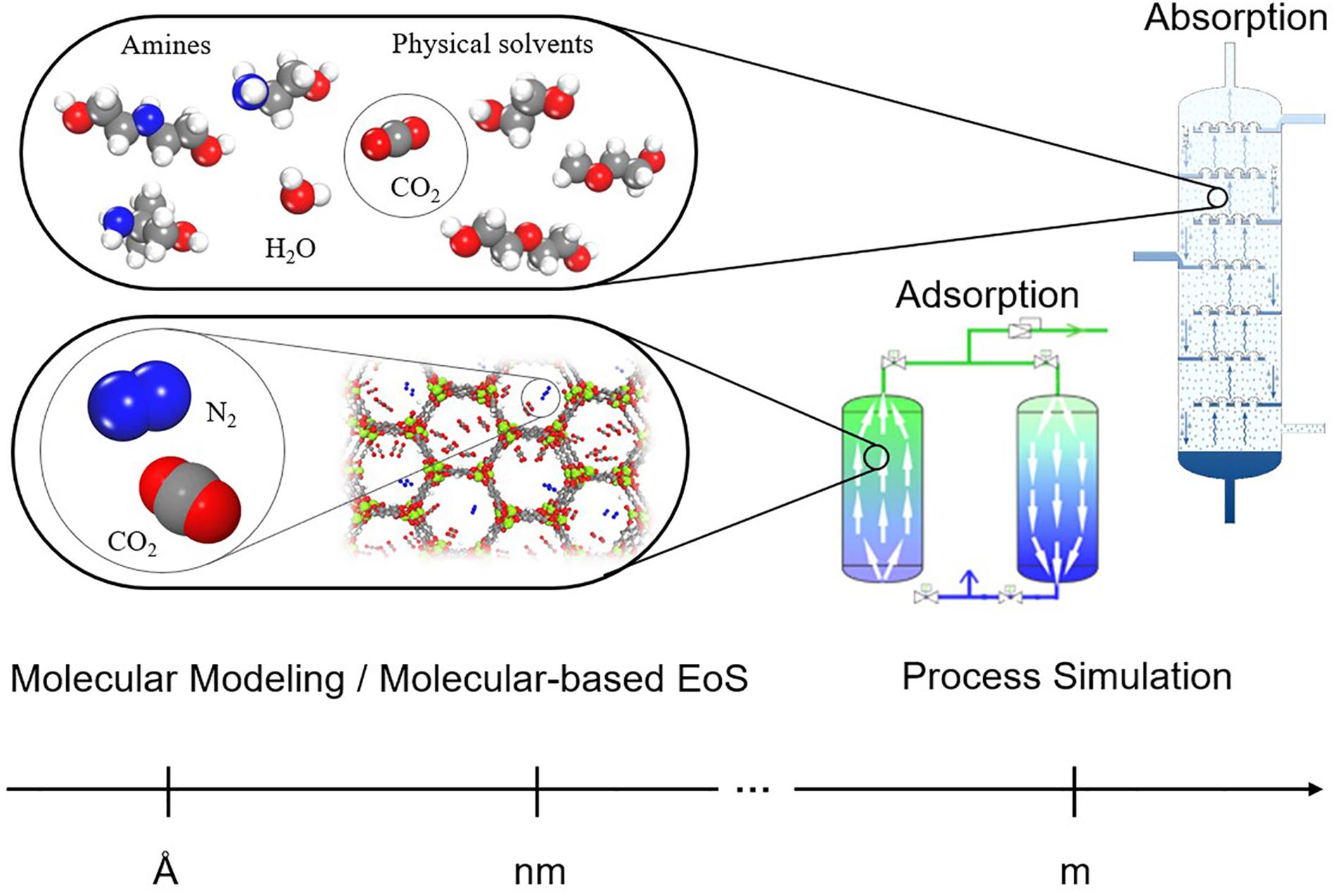
Figure 1. Schematic representation of the length scales involved in the modeling tools used in this work.
Methodology
The first step into applying molecular modeling tools to experimental systems is to propose and validate the models with available experimental data. Then, a further step includes data generation and data collection for novel systems obtained from these models at typical conditions used in industrial processes. Within this approach, it is possible to evaluate the theoretical limits of the energy requirements to capture CO2 under different specific conditions.
In this section, we present a brief introduction of the two different molecular methods used in this work, as well as the main information required and used for developing the transferable models. Absorption and adsorption results are presented for binary gas mixtures containing 25, 15, and 5% CO2 (see Table 1), in order to cover most of the possible typical compositions encountered in industrial processes, including but not limited to the steel and cement industries (Zhang et al., 2014). In all calculations, it was assumed that nitrogen accounted as the surplus of the composition and that the binary mixture was captured at a temperature of 313 K (i.e., 40°C) and atmospheric pressure. It is well known that flue gas contains roughly ∼5% H2O and trace amounts of impurities such as SO2 (Sumida et al., 2012). However, impurities effect is out of the scope of this work (for contributions on this subject, the reader is referred to Bahamon et al., 2018; Alkhatib et al., 2019 and references therein). Moreover, we constrained the range of operating temperatures, opening the possibility of using sources of energy at low temperatures (e.g., low-pressure steam for heating). A condensing steam was assumed to be available at 413 K, with a minimum approach temperature of 10 K.
Statistical Associating Fluid Theory
The Statistical Associating Fluid Theory (SAFT) proposed by Chapman et al. (1989, 1990) is possibly the most well-known Equation of State (EoS) based on statistical mechanics used today for associating and chain molecules in academia and industry. The equation contains key physical information of the fluids. Its parameters are both physically meaningful and transferable, making SAFT a powerful tool for engineering predictions, especially in some applications for which other classical EoSs fail. The success of the equation has been evidenced by the number of published works since its conception (Tan et al., 2008). Different versions of SAFT have been developed over the years. The most popular ones include the original SAFT and simplified SAFT (Chapman et al., 1989; Huang and Radosz, 1990), the soft-SAFT equation (Blas and Vega, 1997; Pàmies and Vega, 2001), the SAFT-VR equation (Gil-Villegas et al., 1997), PC-SAFT (Gross and Sadowski, 2001), and SAFT-γ-Mie (Lafitte et al., 2013).
Statistical Associating Fluid Theory equations are written in terms of the residual Helmholtz energy ares, where each term in the equation represents different microscopic contributions to the total free energy of the fluid. Results obtained from the soft-SAFT equation derived by Blas and Vega (1997, 1998) are presented and discussed in this contribution. The general expression for soft-SAFT is:
where the superscripts ref, chain, and assoc refer to the contributions from the Lennard–Jones (LJ) reference monomers (Johnson et al., 1992), the formation of the chain, and the association interactions (Wertheim, 1984a, b, 1986a, b). An additional term, apolar, can be added to explicitly account for polar interactions arising from dipole or quadrupole moments (Gubbins and Twu, 1978; Twu and Gubbins, 1978; Jog et al., 2001), respectively.
A molecular model for each compound should be proposed within the SAFT framework in order to apply it to experimental systems. This is usually a coarse-grain model where the most relevant features of the molecular structure are captured with a simplified yet reliable manner. In soft-SAFT, substances are treated as spherical LJ (12–6) segments or LJ chains characterized by a specific set of molecular parameters, accounting for the different interactions governing the behavior of the fluid. With this approach, an associating chain molecule is characterized by five molecular parameters: the diameter of the segments making the chains σ, the energy of interaction between segments ε, the chain length m, the association volume κHB, and the association energy εHB. In the case of polar fluids, two additional parameters are needed, being the dipole/quadrupole moment μ/Q, and the fraction of polar segments in the molecules xP, which are fixed a priori rather than regressed to preserve the robustness of the model (Alkhatib et al., 2020).
The molecular parameters are usually regressed simultaneously using experimental data of liquid density and vapor pressure of the pure substance and are further transferred for the description of other properties of the same fluid (such as heat capacities or speed of sound) as well as multicomponent mixtures. During fitting, it is possible to obtain several sets of parameters providing an accurate description of the data. Hence, apart from the numerical optimization, a critical analysis of the values of the parameters and their physical meaning is required to make them transferable and with extrapolative power (Ferreira et al., 2019). Furthermore, it is possible to extend the equation to calculate additional properties by coupling it to other theories. For instance, treating inhomogeneous systems with the Density Gradient Theory (DGT; Duque et al., 2004; Vilaseca et al., 2010; Vilaseca and Vega, 2011) and/or calculating transport properties with the Free-Volume Theory (FVT; Allal et al., 2001; Llovell et al., 2013). For more details on the implementation of soft-SAFT and other theories, the reader is referred to the original papers (Blas and Vega, 1997; Duque et al., 2004; Llovell et al., 2013; Alkhatib et al., 2020).
Molecular Models Used for Absorption With Alternative Amine-Based Solvents
Although a wide variety of aqueous amine solutions has been studied for the CO2 absorption process (Kohl and Nielsen, 1997; Rochelle, 2009; Liang et al., 2015; Puxty and Maeder, 2016), there is still work needed to obtain the best molecules or blends that can overcome the current limitations of the MEA + water system aforementioned. Therefore, absorption with alternative amine-based solvents have been studied in this work using soft-SAFT. Systems assessed include MEA as the benchmark, as well as diethanolamine (DEA), and amino-methyl propanol (AMP). Cosolvents besides water, such as ethylene glycol (EG), diethylene glycol (DEG), triethylene glycol (TEG), and ethylene glycol monomethyl ether (EGME), were also evaluated, effectively forming hybrid chemical–physical solvents when mixed with amines.
Following our previous work (Vega et al., 2009), water was modeled as an LJ sphere with four associating sites, two representing the negative charges lone pair of electrons on the oxygen atom and two representing the positive charges of the hydrogen atoms, while CO2 was modeled as a LJ chain with the quadrupolar moment placed in one of the groups (Dias et al., 2006). MEA, DEA, and AMP were modeled as homonuclear LJ chains, in which their multifunctional groups (amines and hydroxyls) were mimicked with a specific number of association sites for each of them. For details on the models and the allowed interactions, the reader is referred to published works (Lloret et al., 2017; Pereira and Vega, 2018; Pereira et al., 2018; Alkhatib et al., 2019). Conversely, EG, DEG, and TEG were modeled as LJ chainlike molecules with two associating sites of type OH representing the two hydroxyl groups placed at the molecule tails (Pedrosa et al., 2005). Figure 2 shows the soft-SAFT models and molecular structures used for CO2 and H2O, as well as MEA and EG as representatives of the amines and glycols studied. The large circles on the sketch of the soft-SAFT models stand for the LJ groups making the molecules, while the small circles represent the associating sites, which depend on the specific molecules and type of interactions. In the case of binary mixtures of water and amines, cross-association between the water molecule and the functional groups (NH2, NH, OH) of each amine molecule were explicitly considered in the calculations (Alkhatib et al., 2019).
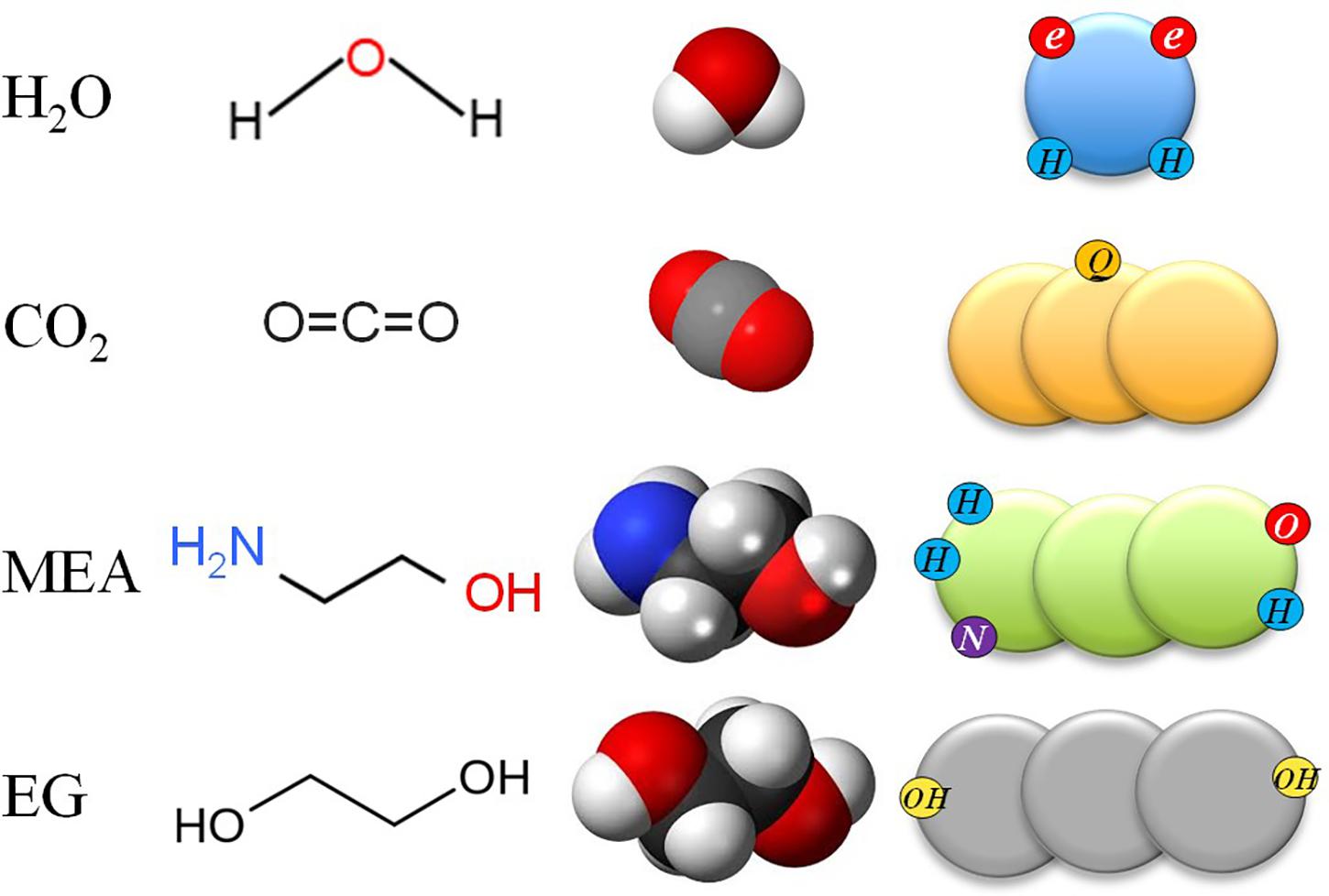
Figure 2. Sketch of the soft-Statistical Associating Fluid Theory (SAFT) molecular models for four of the molecules investigated in this work, from to top to bottom: water, CO2, monoethanolamine (MEA), and ethylene glycol (EG). From left to right: acronym, chemical structure, 3D model, and soft-SAFT model. See text for details.
In order to make the approach as predictive as possible, all the molecular models and parameters used for the soft-SAFT calculations presented in this work were transferred from previous works. Hence, the molecular parameters of the amines were taken from Lloret et al. (2017), Pereira and Vega (2018), Alkhatib et al. (2019), and Pereira et al. (2018); the molecular parameters for water were transferred from Vega et al. (2009), while the parameters for CO2 were taken from Dias et al. (2006). The glycol parameters were taken from Pedrosa et al. (2005).
Depending on the type of amine and the type of cosolvent involved in the absorption process, there are different reaction mechanisms governing the absorption of CO2. The overall reactions can proceed by the formation of carbamate and bicarbonate (Caplow, 1968; Dancwerts, 1978). Note that other thermodynamic models based on classical EoSs, or on activity coefficients, have proved their ability in modeling CO2 absorption in traditional aqueous amines. However, these models require a considerable number of empirical parameters fitted to experimental data (Al-Rashed and Ali, 2012; Suleman et al., 2015) and the a priori knowledge of the reaction equilibrium constants of all relevant ionic species. Following our previous work (Pereira et al., 2018; Alkhatib et al., 2019), the formation of reaction products from the main reactions in the absorption process was implicitly accounted for when using soft-SAFT by the formation of CO2-amine physical aggregates connected through strong and highly directional association sites. The number of these association sites is dictated by the stoichiometry of the reaction between the CO2 and the amine. For the specific association values, the reader is referred to the previously mentioned studies (Pereira et al., 2018; Alkhatib et al., 2019). In this work, we are interested in equilibrium properties; however, examining the reaction mechanism governing the formation of carbamate is essential for understanding the effect on the absorption of CO2 in amines.
Molecular Simulations—Grand Canonical Monte Carlo
Molecular simulations comprise a series of techniques based on statistical mechanics, which are capable of predicting phase equilibria and dynamic and structural properties (Allen and Tildesley, 2017). In this regard, statistical mechanics provides a link between the properties of interacting molecules and the thermodynamics of their bulk phases, by introducing procedures for averaging over a multitude of possible molecular configurations. The fundamental information required to perform molecular simulations are molecular interactions, which are usually described by a forcefield consisting of bonded (e.g., stretching, bending, and torsional interactions), and non-bonded terms [van der Waals (vdW) and electrostatic interactions] as (Smit and Maesen, 2008):
where U refers to the total potential energy. Non-bonded interactions are usually considered with LJ potential for the vdW interactions, in a similar way as in the soft-SAFT model (i.e., σ and ε are comparable), and Coulombic interactions are used for electrostatic interactions.
The two main molecular simulation approaches are Monte Carlo (MC) and Molecular Dynamics (MD; Allen and Tildesley, 2017). MC methods are extensively used for complex molecules calculations, in systems where the temporal evolution is not required, as per calculation of equilibrium properties or for systems for which MD simulations are not affordable due to time scale limitations. Thus, a suitable tool for simulating phase equilibrium systems in confined media, such as adsorption phenomena, is the Grand Canonical Monte Carlo (GCMC) method, which has been extensively used to calculate thermophysical properties in porous materials (Deng et al., 2004; Frost et al., 2006; Herdes et al., 2007; Babarao et al., 2011). In GCMC, the temperature, volume, and chemical potential of the system are fixed. GCMC allows exchanging molecules between the simulation box containing the porous material and an imaginary ideal gas reservoir, by using a fixed number of MC movements (i.e., insertions, deletions, rotations, and translations; Frenkel and Smit, 2002). The procedure is performed over a large number of steps, and then, the adsorption isotherm can be constructed by calculating the average of the number of molecules adsorbed (after equilibration) for every single pressure (chemical potential) point. For a more comprehensive explanation of this and other molecular simulation methods, the reader is referred to excellent textbooks in the field (Frenkel and Smit, 2002; Allen and Tildesley, 2017).
Molecular Models Used for Adsorption on Porous Solid Materials
One of the advantages of using adsorption versus absorption for CO2 capture is that compared to the solvents used in the absorption approach, solid adsorbents materials are not volatile and generally do not pose health hazards (Zanco et al., 2018). However, such materials must present high mechanical and thermal stability and easiness of regeneration and availability, ensuring constant high performance during cyclic operation. Additional characteristics desirable for industrially scalable processes include good behavior under humid/impurity conditions and relatively low cost, to name a few (Plaza et al., 2017).
Zeolites and activated carbons have been traditionally used for gas adsorption and separation. These adsorbents can be tuned to the process needs, providing great opportunities for CO2 capture (Prats et al., 2017; Bahamon et al., 2020). Moreover, MOFs have been one of the fastest growing fields in chemistry and materials science, with a vast number of synthesized structures and with publications increasing year by year (Li et al., 2011). Therefore, in this work, we have used GCMC simulations to study the adsorption behavior of CO2/N2 mixtures on different families of solid materials. Structures evaluated include zeolites (e.g., NaX, NaY, LTA-4A, and MFI), MOFs (e.g., IRMOF-1, MOF-177, M-MOF-74, CuBTC, and UTSA-16), amorphous silica structures (e.g., MCM-41), carbonaceous materials [e.g., zeolite-templated carbon (FAU-ZTC)], activated carbons (AC-DS; Bahamon et al., 2020), and amino-functionalized structures (see Table 1). The molecular models for the adsorbents were taken from X-ray diffraction structures (when crystals; Olson, 1995; Yaghi et al., 2003; Xiang et al., 2012; Queen et al., 2014) or by representations reported in the literature (Lopez-Aranguren et al., 2015; Bahamon and Vega, 2017). Force fields used for each structure can be found elsewhere (Jorgensen and Tirado-Rives, 1988; Builes et al., 2011; Lopez-Aranguren et al., 2015; Martin-Calvo et al., 2015; Bahamon and Vega, 2016; Prats et al., 2017).
All structures were constructed with simulation box dimensions of at least ∼125 Å in each direction, with frameworks treated as rigid structures (Bahamon and Vega, 2016). Simulations were performed by means of LAMMPS (Plimpton, 1995) and Materials Studio software (Dassault Systemes BIOVIA, 2015). For the simulations at each pressure (chemical potential) condition, at least 1.0 × 106 MC moves were performed for data collection after equilibration. The total energy of the system was calculated as the sum of the adsorbate–adsorbate and the adsorbate–adsorbent interaction energies, modeled as a combination of LJ 12-6 and Coulomb potentials. CO2 molecules—as well as N2—were modeled using the TraPPE force field (Potoff and Siepmann, 2001). A cutoff radius of 12.5 Å was applied to the LJ interactions, while the long-range electrostatic interactions were calculated by using Ewald summation, and Lorentz–Berthelot combining rules were used to calculate adsorbate/framework and the LJ crossed parameters.
Results and Discussion
Alternative Amine-Based Solvents for CO2 Capture
Reliable and consistent molecular models were previously developed and validated with pure and binary mixture phase equilibrium data (Pedrosa et al., 2005; Lloret et al., 2017; Pereira and Vega, 2018; Pereira et al., 2018; Alkhatib et al., 2019), providing an accurate prediction with relative overall small deviations with respect to experimental data. Error measurements such as absolute average deviation (AAD) are commonly used to quantify the agreement [although coefficient of determination (R2), index of deviation and mean absolute deviation (MAD) are also reported] (Alkhatib et al., 2019). Models used for the studied amines show% AAD ranging from 2 to 5% for the vapor pressure and an accurate description of the phase behavior of the aqueous solution and CO2 solubility (%AAD from 0.1 to 1.0% for the liquid density; Pedrosa et al., 2005; Lloret et al., 2017; Pereira et al., 2018). The highest deviation was found to be 9% AAD for the vapor pressure of MDEA, related to the exclusion of one degree of freedom in the optimization of the parameters (Pereira et al., 2018; this molecule was not included in the current work). Moreover, glycols and glymes predict binary mixtures with deviations typically ∼1.5% or lower (Alkhatib et al., 2019). Additional details can be found in the respective studies. Moreover, since the available experimental data for amines with physical solvents systems studied such as glycols and glymes is very scarce or inexistent, we have taken advantage of the molecular nature of soft-SAFT to predict the behavior over a broad range of conditions (T, Pi). This makes the present approach an attractive method for comparison and as a tool to search for the best operation conditions.
Solubilities of CO2, αCO2, in the different amine-based solvents at a fixed amine concentration (i.e., 30 wt%) were calculated from soft-SAFT and are presented as isotherms at 313 and 393 K in Figure 3. If one focuses, for instance, in the absorption at a CO2 partial pressure of 25 kPa (i.e., 25% CO2 in the mixture inlet stream at 313 K), it can be seen that higher absorption capacities than MEA + water are achievable with AMP (either with water, EG, DEG, or TEG as solvent). However, if the concentration is decreased to 5% CO2 (i.e., 5 kPa), a different behavior is observed: in this case, only the aqueous solution with AMP shows higher capacity than MEA + water. In addition, most of the systems display a very low loading at high temperatures, which in principle implies that higher or, at least similar, cyclic capacities to the ones obtained with MEA could be achieved at lower lean temperatures. Moreover, it can be seen that MEA is more prone to change its absorptive behavior with changes in CO2 concentration than changes in temperature, as can be observed in Figure 3B. DEA and AMP molecules show a more sustained absorption capacity with partial pressure due to the mechanism of CO2 absorption in these systems, as the aqueous MEA solvent moves from carbamate to bicarbonate formation, depending on the amount of CO2 in the raw flue gas, while the remaining solvents, even with physical cosolvents, absorb CO2 mainly through the formation of carbamate. Although absorption of CO2 does not seem to be as efficient with non-aqueous solvents as in aqueous amines in terms of absorption capacity, as also observed by Park et al. (2005), this actually results in lower regeneration temperatures due to the lower enthalpy of vaporization and heat capacity of physical cosolvents compared to water, leading to potential significant reductions in the energy of regeneration (Rivas and Prausnitz, 1979; Hamborg et al., 2011; Zhang et al., 2018). Such a trade-off between solvent absorption capacity and energy of regeneration can be fine-tuned through process optimization.
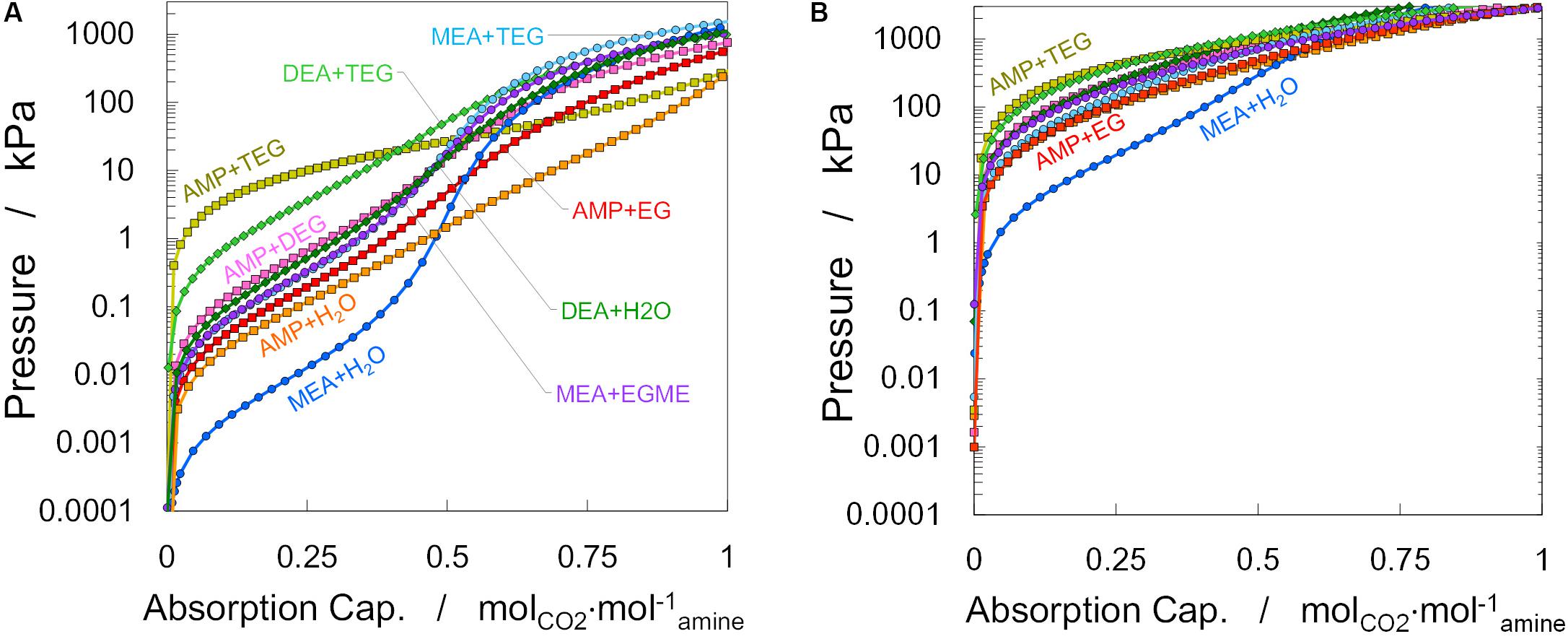
Figure 3. Comparison of calculated partial pressure of CO2 as function of CO2 absorption capacity (solubility) in aqueous and non-aqueous amine-based solutions (30 wt%) at different temperatures: (A) 313 K and (B) 393 K.
Toward assessing the performance of solvents for the absorption of CO2, the absorption cyclic capacity and the regeneration energy index have been recognized as one of the most influential KPIs (Pereira and Vega, 2018) with direct implications on the overall technical and economic feasibility of absorption systems. Hence, for a proper and fair comparison between the different alternative amine-based systems evaluated in this work, we have calculated the cyclic working capacity (in kg CO2 m–3sln) and the energy index (in MJ kg CO2–1). The cyclic working capacity, ΔαCO2, is related to the amount of CO2 that is effectively separated from the feed gas, defined as the difference between the absorbed amount (solubility) at the feed gas partial pressure and temperature conditions (rich), and the absorbed amount at the operating regeneration conditions (lean):
The amount of CO2 at both rich and lean conditions were directly calculated from soft-SAFT for the VLE of CO2 + amine + cosolvent at the required conditions of temperature and pressure. The energy index is defined as the required energy per kilogram of CO2 desorbed from the rich condition to the lean condition (i.e., the cyclic working capacity). It includes the sensible heat Qsens, the latent heat (for highly volatile systems) Qvap, and the absorption heat Qabs.
The energy index and its individual contributions were calculated using the shortcut method of Kim et al. (2015), with all required thermophysical properties of pure components, such as heats of vaporization, heat capacities, heats of absorption, and concentrations of CO2 for the mass balance around the stripping column, obtained from soft-SAFT. Similar comparisons can be found in the literature (Kim et al., 2015; Zhang et al., 2015; Budzianowski, 2016; Li et al., 2016; Bernhardsen and Knuutila, 2017). Nevertheless, note that the comparison presented here is performed in volumetric units (cubic meter of solution, containing 30 wt% amine, and 70 wt% cosolvent), which implies the same volume of absorber equipment for all the different studied systems (Bahamon and Vega, 2016).
The evolution of the lean loading conditions (desorption) was evaluated assuming a stripper column operating at a total pressure of 200 kPa (Pereira and Vega, 2018). Figure 4 depicts the results for the three different CO2 compositions: 25, 15, and 5%. Values were compared with MEA as a benchmark, creating four quadrants in the chart: the best option(s) must show lower energy index with higher cyclic working capacities and hence must appear in the lower right quadrant. Moreover, the size of the sphere in Figure 4 represents the required temperature to achieve such conditions, since the desorption/lean temperature depends on the specific solvent: the larger the sphere size, the higher the temperature used for regeneration, evaluated with the same shortcut method and typical solvent regeneration process configuration.
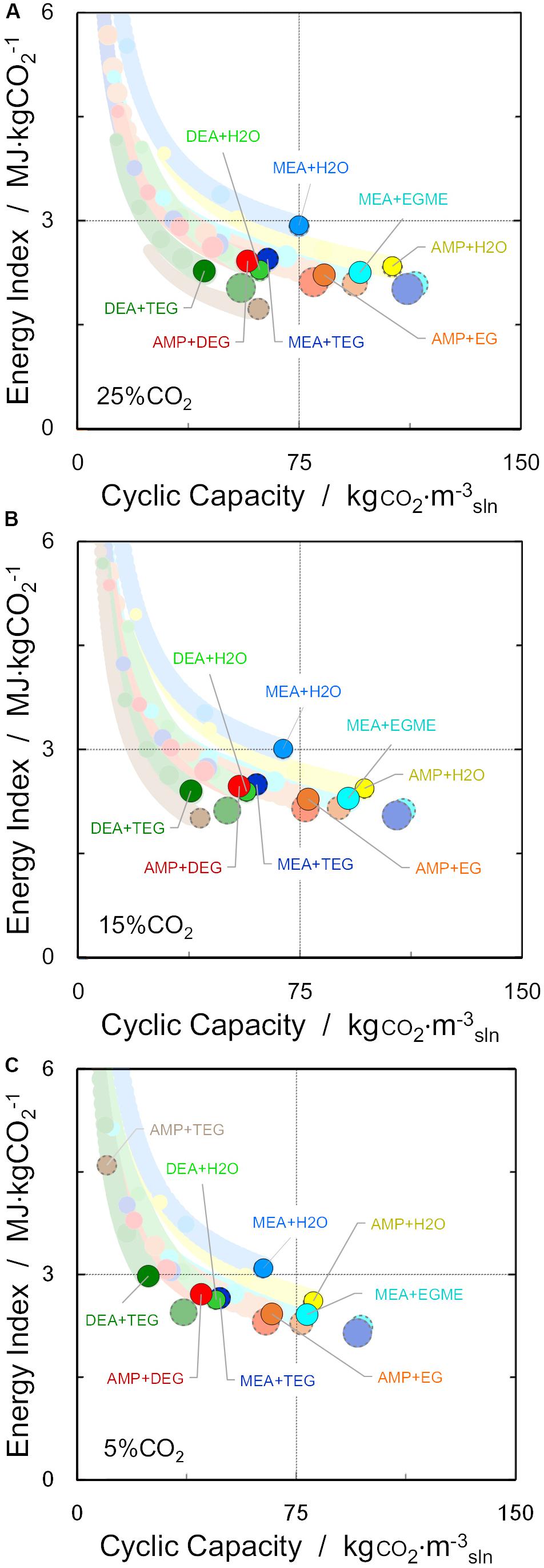
Figure 4. Estimated evolution of solvent cyclic capacity and energy index of alternative aqueous amines (30 wt%) for (A) 25% CO2/75% N2, (B) 15% CO2/85% N2, and (C) 5% CO2/95% N2 binary mixtures (Trich = 313 K, Plean = 0.2 MPa). Highlighted spheres correspond to a regeneration temperature of 403 K, while dashed spheres represent the maximum cyclic capacity achieved with each material (at Tlean > 403 K). Colors at the background indicate regeneration at lower regeneration temperatures.
Note that, depending on the operating conditions, there can be a trade-off between low regeneration energy and high cyclic working capacities; also when the CO2 concentration in the inlet stream is increased, the energy indexes tend to decrease (compensated by higher cyclic capacities). In the case of 30 wt% MEA + water, calculations show a cyclic working capacity in the range of 60–75 kg CO2⋅m–3sln, and a corresponding regeneration energy of 3.2–2.8 MJ kg CO2–1, in good agreement with literature estimates for a MEA-based CO2 capture plant with a conventional process configuration (Boot-Handford et al., 2014). It can be seen in Figure 4 that a number of amines can achieve higher cyclic capacities or lower energy indexes than MEA. In addition, results show that for the same total amine mass concentration, non-aqueous amine solvents possess a 5–10% reduction in cyclic working capacity, with a 10–30% decrease in the energy index compared to their aqueous counterparts, mainly due to lower heat of vaporization and sensible heat (Alkhatib et al., 2019). The lowest regeneration energy was estimated for MEA + EGME and for systems with AMP, due to the previously discussed in Figure 3. Moreover, the higher temperature of regeneration of these solvents compared to their aqueous counterparts is not reflective of the expected lower temperature of regeneration, partly due to the shortcut method being developed for typical stripper configurations with water as cosolvent, and partly due to the comparison. A more accurate estimation of the temperature of regeneration would entail examining different process configurations for these low volatility cosolvents.
As already mentioned, the shortcut model represents ideal cases indicating an upper bound of performance, where heat and mass transfer are instantaneous and no temperature or concentration gradients exist (Ajenifuja et al., 2020). Moreover, accurate prediction of the energy index is dependent on reliable estimates of the specific heat capacity of the studied solvents. Since specific heat capacities were taken constant, predictions of energy index should be interpreted with caution. Nevertheless, the simple analysis implemented in this work allows a systematic comparison for initial solvent screening, as all of them have been studied under the same conditions. Nonetheless, more rigorous process modeling for these solvents, once screened for the most promising ones, are required for a holistic understanding of their performance, which are outside the scope of the current work. Other thermophysical properties, such as viscosity and surface tension, and other relevant properties to process design such degradation and evaporation should be included. For an excellent example on the implications of including transport properties in the cost analysis of absorbent materials, the reader is referred to a work by Mota-Martinez et al. (2017).
Furthermore, the presence of impurity gases such as SOx and NOx can greatly complicate the CO2 separation process, producing unwanted reaction products, and/or degrading the solvents used for the separation (Mello and Eiü, 2002). For instance, in the benchmark absorption with MEA, the column cannot be fully regenerated because of irreversible degradation in reaction with oxygenated compounds that can produce foaming, fouling, and formation of unregenerable heat-stable salts in the amine, among others (Supap et al., 2006). SO2 control is particularly important because its concentration (500–3,000 ppm) is higher than any other acid gas components in the flue gas (Rao and Rubin, 2002). Hence, a previous desulfurization process is often included in industrial recovery processes. Nevertheless, although deep desulfurization with >99% SO2 removal is achievable, such an operation has economic implications (Lee et al., 2009). Moreover, regarding the modeling of impurities, the interactions of CO2/H2O/SOx/NOx in typical flue gas with absorbents are quite complex. As pointed out by Lee et al. (2009), the information on the reaction rates of heat-stable salt formation between MEA and acid gases can rarely be found in the literature. Nevertheless, as an example of the capabilities of using a molecular-based model, CO2 partial pressures in acid media were previously predicted in our group by using DEA, MEA, and MDEA via modeling with soft-SAFT (Alkhatib et al., 2019). Index of agreement values of 0.844, 0.766, and 0.705 were obtained, respectively, with differences in the performance of the model attributed to the availability of amines concentrations range used when optimizing the cross-association energy parameters for each acid gas–amine pair (Alkhatib et al., 2019). However, the model can be easily reparametrized to a broader range of conditions with recent progress in the field and more experimental data.
Novel Porous Solid Adsorbents for CO2 Capture
In this section, a computational study of several adsorbent materials for CO2 capture and separation is presented. Simulated adsorption isotherms were previously validated with experimental data in order to determine the accuracy of the models, as well as the quality of the multicomponent mixture predictions. Very good agreement was obtained for all pure component isotherms (AAD, ∼3–8% for CO2, and <4% for N2; Builes et al., 2011; Prats et al., 2017b; Bahamon et al., 2020), although some deviations above 11% were obtained at higher pressures for MOFs with uncoordinated metal centers (e.g., M-MOF-74), due to limitations in the force fields available in the literature (Bahamon and Vega, 2016). A comparison of the adsorption isotherms for pure CO2 at a temperature of 313 K is provided in Figure 5. As shown, the M-MOF-74 family displays a high affinity for carbon dioxide at low pressures (especially the structures with Mg and Ni as metal centers), followed by zeolites like NaX and NaY. Operation in the low-pressure range is beneficial for zeolites, which more easily attract the quadrupole present in CO2. However, when the operating pressures exceed atmospheric conditions, they become saturated due to their smaller pore volumes and because of the extra framework cations; higher uptake capacities can be then obtained with MOFs or ACs, instead. In addition, IRMOF-1 and MOF-177 display roughly linear trends of adsorption vs. pressure, indicating that the frameworks are not very selective for CO2 adsorption and saturation capacity can be reached at higher pressure conditions.
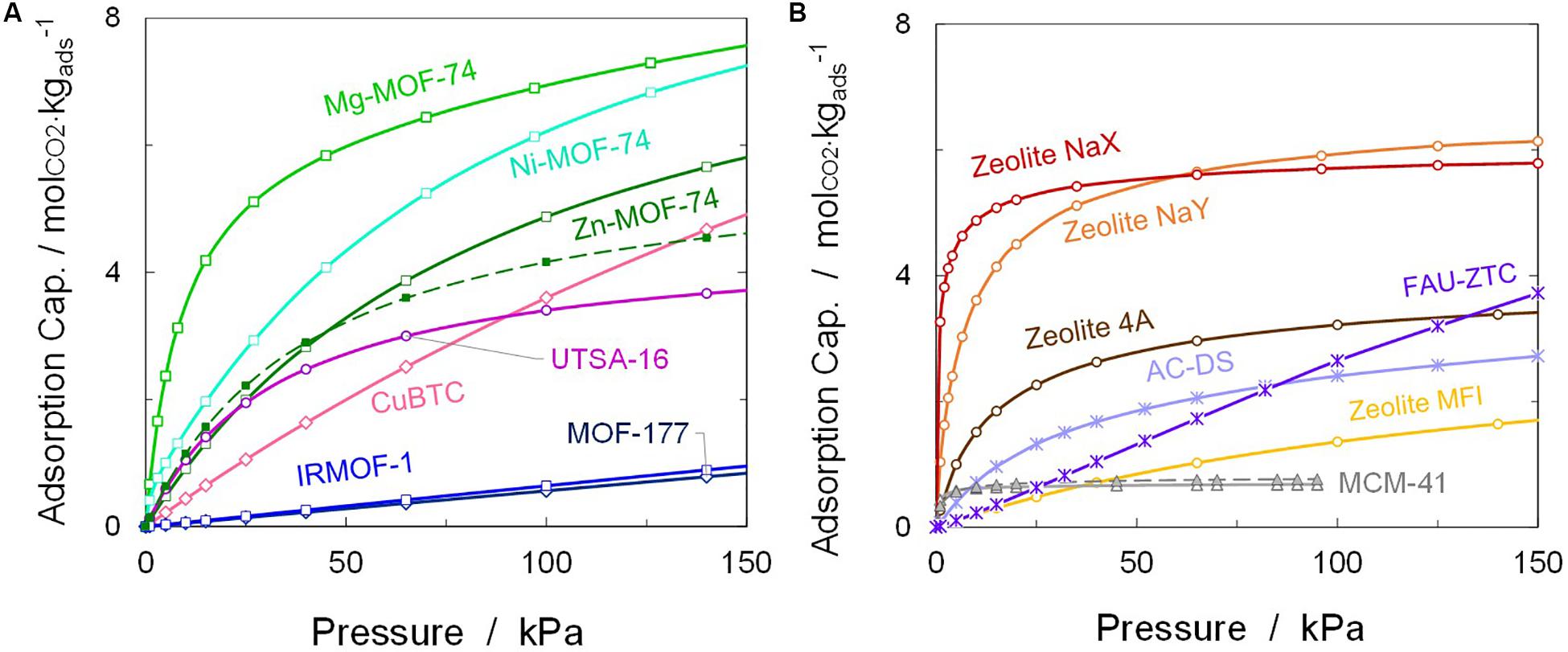
Figure 5. Comparison of simulated adsorption isotherms for pure CO2 on (A) representative metal–organic frameworks (MOFs) and (B) zeolites and other selected adsorbent materials. Dashed lines for functionalized materials. Tads = 313 K (MCM-41 at 323 K).
Phenomena as coadsorption and/or site competition should be taken into account when evaluating process streams, since they can affect the performance of the materials for capturing CO2 from an actual flue gas mixture (Bahamon et al., 2018); however, the amount of experimental data at these conditions is very scarce. Prediction of the mixture behavior can be obtained by the parametrization of pure isotherms, although the applicability can fail when preferential adsorption location at the intersections of the framework is present, as it is the case for highly selective materials for CO2-over-N2 such as of NaX, UTSA-16, and Mg-MOF-74. Conversely, GCMC simulations allow direct calculation of the interactions between surface and molecules, explicitly including the adsorption competition between substances (Bahamon et al., 2020). This is one of the cases in which molecular simulations can be used as a robust, complementary, tool to the experiments.
In order to make a realistic comparison for gas streams at process conditions, results for selected materials are presented in Figure 6 for the same CO2 concentrations as for absorption in amine-based solvents. It was observed that CuBTC and MOF-177 show poor performance due to competition with nitrogen, which reduces the adsorbent capacity (and therefore, the purity that can be achieved in a swing adsorption cycle). In contrast, Mg-MOF-74 and zeolite NaX exhibit exceptional CO2 storage capacity and selectivity (Bahamon et al., 2018). Moreover, in addition to the adsorption isotherms, GCMC also provides useful molecular insights into physical phenomena occurring at such operating conditions; hence, we present in Figure 6 some snapshots of CO2/N2 binary mixture for the different adsorbent families. It can be seen, for instance, that the honeycomb-like structure of Mg-MOF-74, with large one-dimensional pores and open-metal centers, is highly attractive for carbon dioxide molecules (especially with adsorption close to the metal centers).
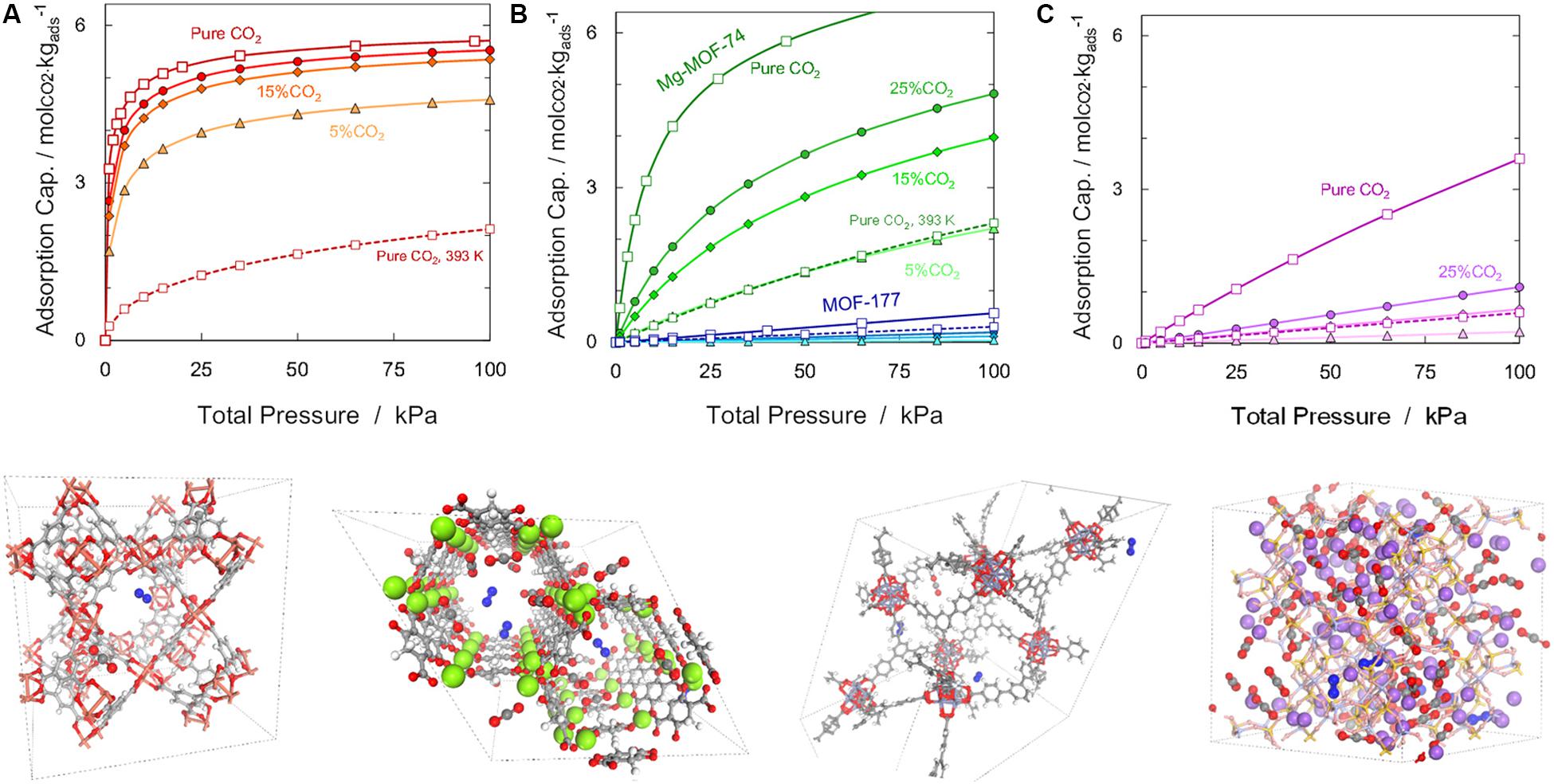
Figure 6. Top: Comparison of simulated adsorption isotherms for pure carbon dioxide (squares) and the behavior under different mixture conditions: 25% CO2/75% N2 binary mixture (circles), 15% CO2/85% N2 binary mixture (diamonds), and 5% CO2/95% N2 binary mixture (triangles) for (A) NaX, (B) Mg-MOF-74 and MOF-177, and (C) CuBTC at 313 K. Dashed lines for pure CO2 at 393 K. Bottom: Snapshot of competition sites on selected crystallographic structures at 100 kPa and 313 K for 5% CO2/95% N2 binary mixture: color key—O, red; C, gray; Mg, green; H, white; Cu, orange; Zn, purple; N, blue; Si, yellow; Al, pink; and Na, violet.
Molecular simulations were further used to evaluate the behavior at operating conditions for temperature swing adsorption (TSA) processes, by assuming one can take advantage of low-grade thermal energy from the same industrial plant at which the CO2 capture unit is going to be located. A simplified model was used for the screening (Ajenifuja et al., 2020), as in the previous section with absorption. The cyclic working capacity was calculated as the actual amount Δn, recovered between adsorption and desorption conditions. Adsorption was obtained at 313 K and CO2 compositions of 25, 15, and 5%, while desorption was obtained as pure CO2 at temperatures up to 403 K:
In addition, the energy index (i.e., energy per amount recovered) was calculated considering the energy required to desorb the molecules from the solid material (also called isosteric heat qST) and the energy to heat those molecules up to the desorption temperature (i.e., sensible heat), as follows:
where CP are the heat capacities of the gases taken as an average constant value between adsorption and desorption temperatures.
A comparison of solid adsorbents in terms of cyclic working capacity and energy index is presented in Figure 7, analogous to the absorption case. Since in an adsorption process, both CO2 and N2 can be captured on the adsorbent material, a high selectivity for carbon dioxide is prerequisite in order to achieve high purities at the outlet, achieving similar specifications as for absorption. In this case, a minimum value of 90% CO2 on the adsorbent was set as limit to compare the materials for industrial processes. In the literature, a few examples can be found utilizing theoretical methods to screen cost-effective materials for CO2 capture (Krishna and van Baten, 2012; Lin et al., 2012; Wilmer et al., 2012; Maring and Webley, 2013; Huck et al., 2014; Dunstan et al., 2016), with typical adsorbent evaluation criteria such as structure–property relationships (e.g., surface area and pore volume), working CO2 capacity, selectivity, or sorbent selection parameter. Nevertheless, as the case of absorbents, the proper metric for comparing different adsorbents should be a volumetric basis, taking the bed volume and space requirements of the facilities as constraints. Furthermore, it is worth mentioning that because the gas stream is typically discharged at near atmospheric conditions, compressing or extracting a vacuum to such huge amounts of the dry gas stream is predicted to be more costly than heating the solid material (Hedin et al., 2013). A fair comparison of energy requirements between the two technologies studied here (absorption and adsorption) is presented using the same thermal energy concept. In this case, the crystal densities of the materials were used, with the void fraction of the bed held constant and equal to 0.4.
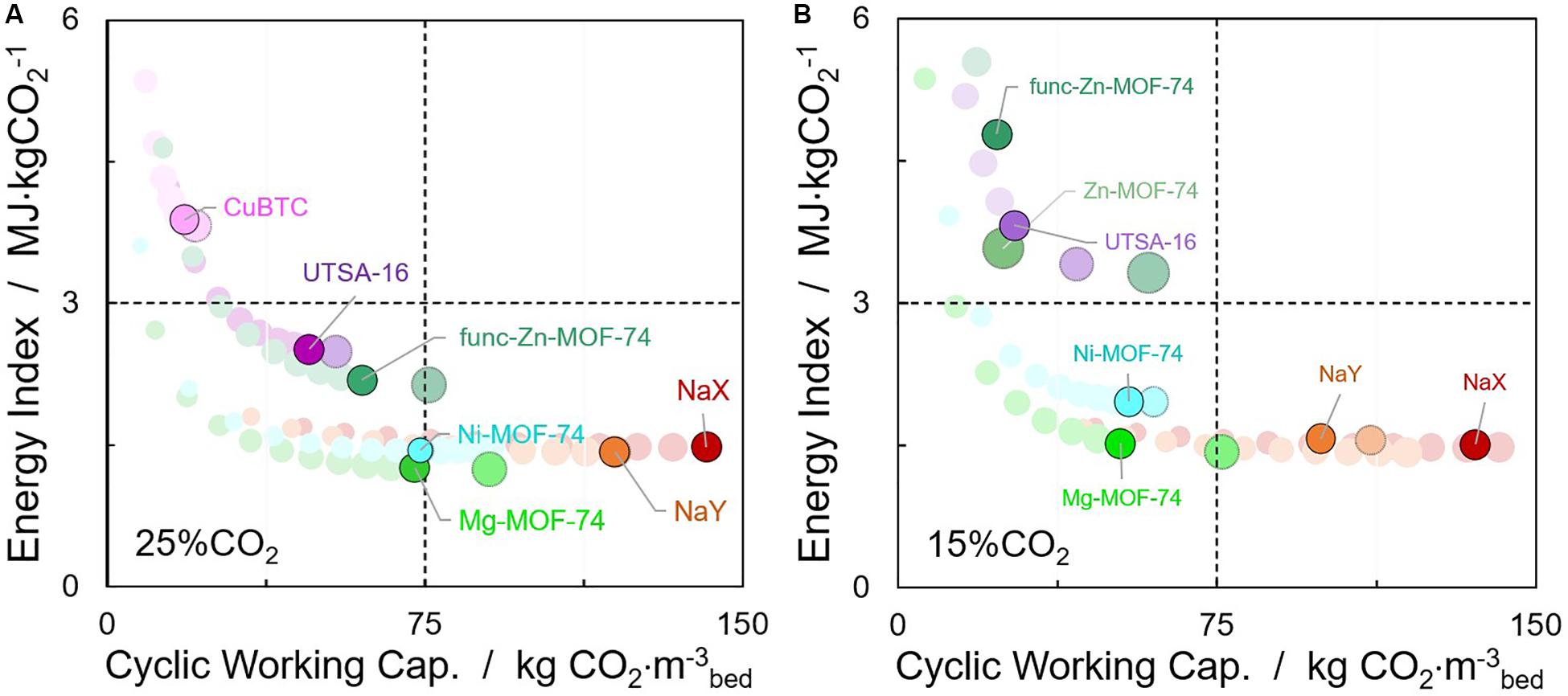
Figure 7. Estimated evolution of cyclic working capacities and energy indexes of adsorbent materials achieving purities > 90% for temperature swing adsorption (TSA) process with (A) 25% CO2/75% N2 and (B) 15% CO2/85% N2 binary mixtures. Sphere size related to the desorption temperature (Tads = 313 K). Highlighted spheres correspond to a regeneration temperature of 403 K, while dashed spheres indicate optimized values at higher desorption temperatures. Binary 5% CO2/95% N2 composition plot have been omitted since none of the materials allow achieving the specified purity.
As shown in Figures 7, 4 of the 16 adsorbent materials examined (including zeolite NaX) are able to achieve low energy indexes with high cyclic working capacities (lower right quadrant) for 25% CO2, as well as to satisfy the purity specifications. If the concentration is reduced to 15% CO2, only three of these adsorbents are able to meet the performance specifications, and none of the materials is capable of achieving concentrations over 90% CO2 on the adsorbent starting with a stream with 5% CO2. M-MOF-74 and zeolites NaX and NaY offer the best results, demonstrating that these materials can be appropriate for this TSA technology. Adsorption-based processes with values as low as 1.45 MJ kg CO2–1 have been obtained, without including the waste heat required to build steam [estimated in 1.26 MJ kg CO2–1 (Joss et al., 2017)]. In similar screening exercises for post-combustion carbon capture by VSA processes, UTSA-16 and Mg-MOF-74 were identified as the top-ranked materials by energy index (Khurana and Farooq, 2016), showing the tendency to adapt to changes in T,P of the latter. It should be mentioned that materials with less affinity for carbon dioxide such as ACs, MCM-41, etc. present low cyclic working capacities for the condition presented; however, higher adsorption pressures will result in a much higher amount of CO2 recovered with these materials.
As seen, useful insights about the sensitivity of adsorbent performance to changes in operating conditions can be extracted. In some cases, higher working capacities than the ones obtained for the minimum of the energy index are achievable with a slight increase of ∼10% in the energy requirements (see dashed spheres in Figure 7). However, this comes as an expense of higher temperatures needed for regeneration. Nevertheless, this characteristic of some adsorbents must be addressed. Since less cycles are required to capture the same amount of CO2, the whole capture process is faster and cheaper (Vujic and Lyubartsev, 2017). Furthermore, the election of the desorption temperature must be counterbalanced with the energy demand of the process (Plaza et al., 2017) and with the cost of heating the bed depending on the quality of heating required. If higher pressure steam were used for heating, which is a more valuable utility, higher desorption temperatures, and higher working capacities might be achieved (Ajenifuja et al., 2020). In addition, as the adsorbent is more selective for CO2 than N2, CO2 uptake is more sensitive to increase in the desorption temperature, thus leading to an increase in CO2 purity.
From an engineering point of view, swing adsorption cycles can be fine-tuned to fulfill a variety of demanding requirements. Increasing the complexity of the cycle by adding additional recycle, purge, secondary heating steps, heat recovery, or low adsorption temperatures by using chilled water or refrigerants could improve the performance of the adsorbent (Grande and Rodrigues, 2008; Radosz et al., 2008; Plaza et al., 2010; Ntiamoah et al., 2016; Sinha et al., 2017; Zhao et al., 2019), but such integration of the process is out of the scope of this work. In addition, with the assumption of equilibrium adsorption in the model, cycle productivity cannot be directly estimated. Consideration of heat-transfer rates and other transport properties, although important for the detailed design, is not critical for the initial screening of candidate adsorbents under process conditions (Ajenifuja et al., 2020), although detailed process optimization will definitely provide the most accurate ranking (Balashankar et al., 2019).
Finally, it is worth mentioning that some adsorbent structures achieve similar values to the ones obtained in the previous section for alternative amine-based systems (2–2.5 MJ kg CO2–1). Nevertheless, TSA processes present the disadvantage of the time delay imposed by the heating and cooling steps and the purity reached under very low CO2 concentrations in the inlet stream. Further improvements in the cycle time process optimization are required, intrinsically associated with the feed flowrate, the height of the adsorber, adsorbent characteristics, and the cycle design, among others. Its future applicability will rely upon the stability of the novel material and the price.
In addition, capturing CO2 from typical flue gases encompasses an additional difficult related to the presence of water, NOx, SOx, and other impurities, which are detrimental to most CO2-selective adsorbents. It is well known that water adsorption excludes CO2 from the adsorption sites and hence could potentially annul all separation capacity of the adsorbent (Zhang et al., 2009). For instance, the equilibrium adsorption capacity of CO2 on NaX decreased by 99% when using untreated flue gas (i.e., in the presence of H2O; Li et al., 2008). Therefore, it is necessary to use prepurification units or prelayers of adsorbent with excellent water working capacity for effective CO2/H2O swing adsorption processes (Cavenati et al., 2006). Activated alumina and silica gel are common adsorbents used in industries for removing water vapor (Kumar et al., 2003; Serbezov, 2003). As aforementioned for the absorption process, such pretreatment stages could considerably increase the overall cost. With respect to molecular simulation studies focused on this endeavor, Huang et al. (2012) found that the interaction between water molecules and the framework plays a crucial role when evaluating the effect of a trace amount of water on CO2 capture. Liu et al. (2014) showed that the presence of H2O reduces the CO2 adsorption ability but increases the CO2/N2 separation factor. In a previous work from our group, the impact of the impurities on the performance of the materials was evaluated at the molecular level and process conditions for selected MOFs and comparing with the benchmark NaX material (Bahamon et al., 2018). Results showed that the zeolite is a more hydrophilic adsorbent than CuBTC and Mg-MOF-74, becoming useless with <0.1% of water content in the mixture, and just a 0.01% of moisture is able to significantly reduce the CO2 adsorption capacity. Moreover, the energy requirements grows exponentially with the inclusion of H2O and SO2, although it is remarkable that there are “sweet spots” in MOF-74 where the use of small traces of impurities allow an enhancement in the KPIs due to the competition with nitrogen for the remaining adsorption sites (Bahamon et al., 2018). Nevertheless, more fundamental work needs to be done to gain a better understanding on the mechanism of these interactions and their effect on the process performance (Zhang et al., 2009) to be further transferred to the proposed methodology used in this contribution. Production of ad hoc experimental data is required for validating purposes.
Conclusion
In this work, we have shown, through some specific cases, how molecular modeling techniques (specifically soft-SAFT and GCMC) can be used to obtain thermophysical properties of alternative selected solvents and adsorbents for CO2 capture, allowing a systematic comparison of the effect of the molecular structure on the performance of both technologies. The cyclic working capacity and energy index were used as the two KPIs to evaluate the performance of the selected systems under the same operating conditions. In the case of solvents, aqueous MEA was used as the benchmark, systematically searching for alternatives requiring lower energy index with higher cyclic working capacities. We have obtained that, for same total amine mass concentration, non-aqueous amine solvents provide a 5–10% reduction in cyclic working capacity, with a 10–30% decrease in the energy index compared to their aqueous counterparts. Sixteen adsorbents, belonging to different types of materials, were also compared under the same conditions, obtaining that M-MOF-74 and zeolites NaX and NaY provide the best results for adsorption in TSA processes. A comparison between the different cases studied for solvents and adsorbents show that some adsorbent materials need similar values of energy requirement to the ones obtained for alternative amine-based systems (2–2.5 MJ kg CO2–1), although the disadvantage of the TSA process versus absorption should be taken into account, as already explained.
Overall, this work shows that a simplified analysis can be used to guide the development and selection of the most promising systems for CO2 capture, based on key process parameters. A wide range of operating conditions can be studied, and useful insights into the impact of operating conditions on the performance can be gained with this approach. Considering the high degree of transferability of the model parameters, the applied approach stands as a robust and valuable/effective tool for the screening and discovery of new possible systems for carbon capture. Further work will include extensions to hypothetical systems (not evaluated experimentally yet) and implementation in engineering simulators for process simulation and design. It has been shown that CO2 capture technologies, with lower energy requirements than the current ones, can be implemented in the industrial sector. However, in combination with the Paris Agreement climate ambition, establishing markets for premium lower-carbon materials can also accelerate the adoption of such CCUS processes.
Data Availability Statement
The raw data supporting the conclusions of this article will be made available by the authors, without undue reservation.
Author Contributions
DB is a research scientist who carried out some of the GCMC simulations work, data analyses and interpretation, and writing of the manuscript. NA is a research assistant who performed the GCMC calculations for MOFs at different concentrations. SB is an assistant professor who performed GCMC simulations of silica-based and functionalized silica-based materials. IA is a Ph.D. student who performed the soft-SAFT calculations and interpretation. MS is an assistant professor who helped in the design and interpretation of the simulation work. LV is a professor and the academic supervisor of the work; together with DB, she defined the strategy of the work, followed up on the results and interpretation and revised the overall work. All authors have contributed in the drafting and the revision of the article.
Funding
Financial support for this work has been provided by Khalifa University (project RCII2019-007, RICH Center). Partial financial support by ADNOC Gas Processing and their shareholders, the Abu Dhabi National Oil Company (ADNOC), Shell Abu Dhabi, Total SA and Partex, through the Gas Research Center (project no. GRC18003) was provided for the early development of this work. Computational resources from KU are highly appreciated.
Conflict of Interest
The authors declare that the research was conducted in the absence of any commercial or financial relationships that could be construed as a potential conflict of interest.
References
Abanades, J. C., Anthony, E. J., Wang, J., and Oakey, J. E. (2005). Fluidized bed combustion systems integrating CO2 capture with CaO. Environ. Sci. Technol. 39, 2861–2866. doi: 10.1021/es0496221
Ajenifuja, A., Joss, L., and Jobson, M. (2020). A new equilibrium shortcut temperature swing adsorption model for fast adsorbent screening. Ind. Eng. Chem. Res. 59, 3485–3497. doi: 10.1021/acs.iecr.9b05579
Alkhatib, I. I. I., Bahamon, D., Llovell, F., Abu-Zahra, M. R. M., and Vega, L. F. (2020). Perspectives and guidelines on thermodynamic modelling of deep eutectic solvents. J. Mol. Liquids 298:112183. doi: 10.1016/j.molliq.2019.112183
Alkhatib, I. I. I., Pereira, L. M. C., AlHajaj, A., and Vega, L. F. (2019). Performance of non-aqueous amine hybrid solvents mixtures for CO2 capture: a study using a molecular-based model. J. CO2 Util. 35, 126–144. doi: 10.1016/j.jcou.2019.09.010
Alkhatib, I. I. I., Pereira, L. M. C., Torne, J., and Vega, L. F. (2020). Polar soft-SAFT: theory and comparison with molecular simulations and experimental data of pure polar fluids. Phys. Chem. Chem. Phys. 22, 13171–13191. doi: 10.1039/D0CP00846J
Alkhatib, I. I. I., Pereira, L. M. C., and Vega, L. F. (2019). 110th anniversary: accurate modelling of the simultaneous absorption of h2s and co2 in aqueous amine solvents. Ind. Eng. Chem. Res. 58, 6870–6886. doi: 10.1021/acs.iecr.9b00862
Allal, A., Moha-ouchane, M., and Boned, C. A. (2001). New free volume model for dynamic viscosity and density of dense fluids versus pressure and temperature. Phys. Chem. Liq. 39, 1–30. doi: 10.1080/00319100108030323
Allen, M. P., and Tildesley, D. J. (2017). Computer Simulation of Liquids, 2nd Edn, Oxford: Oxford University Press.
Al-Rashed, O. A., and Ali, S. H. (2012). Modeling the solubility of CO2 and H2S in DEA-MDEA alkanolamine solutions using the electrolyte-UNIQUAC model. Sep. Purif. Technol. 94, 71–83. doi: 10.1016/j.seppur.2012.04.007
Asif, M., Suleman, M., Haq, I., and Jamal, S. A. (2018). Post-combustion CO2 capture with chemical absorption and hybrid system: current status and challenges. Greenh. Gas Sci. Technol. 34, 1–34. doi: 10.1002/ghg.1823
Babarao, R., Dai, S., and Jiang, D.-E. (2011). Functionalizing porous aromatic frameworks with polar organic groups for high-capacity and selective CO2 separation: a molecular simulation study. Langmuir 27, 3451–3460. doi: 10.1021/la104827p
Bahamon, D., Díaz-Márquez, A., Gamallo, P., and Vega, L. F. (2018). Energetic evaluation of swing adsorption processes for CO2 capture in selected MOFs and zeolites: effect of impurities. Chem. Eng. J. 342, 458–473. doi: 10.1016/j.cej.2018.02.094
Bahamon, D., Ogungbenro, A. E., Khaleel, M., Abu-Zahra, M. R. M., and Vega, L. F. (2020). Performance of activated carbons derived from date seeds in CO2 swing adsorption determined by combining experimental and molecular simulation data. Ind. Eng. Chem. Res. 59, 7161–7173. doi: 10.1021/acs.iecr.9b05542
Bahamon, D., and Vega, L. F. (2016). Systematic evaluation of materials for post-combustion CO2 capture in a temperature swing adsorption process. Chem. Eng. J. 284, 438–447. doi: 10.1016/j.cej.2015.08.098
Bahamon, D., and Vega, L. F. (2017). Pharmaceuticals removal from water effluents by adsorption in activated carbons using Monte Carlo simulations. Comput. Aided Chem. Eng. 40, 2695–2700. doi: 10.1016/b978-0-444-63965-3.50451-7
Bai, P., Jeon, M. Y., Ren, L., Knight, C., Deem, M. W., Tsapatsis, M., et al. (2015). Discovery of optimal zeolites for challenging separations and chemical transformations using predictive materials modeling. Nat. Commun. 6:5912. doi: 10.1038/ncomms6912
Balashankar, V. S., Rajagopalan, A. K., De Pauw, R., Avila, A. M., and Rajendran, A. (2019). Analysis of a batch adsorber analogue for rapid screening of adsorbents for postcombustion CO2 Capture. Ind. Eng. Chem. Res. 58, 3314–3328. doi: 10.1021/acs.iecr.8b05420
Bernhardsen, I. M., and Knuutila, H. K. (2017). A review of potential amine solvents for CO2 absorption process: absorption capacity, cyclic capacity and pKa. Int. J. Greenh. Gas Control 61, 27–48. doi: 10.1016/j.ijggc.2017.03.021
Blas, F. J., and Vega, L. F. (1997). Thermodynamic behaviour of homonuclear and heteronuclear Lennard-Jones chains with association sites from simulation and theory. Mol. Phys. 92, 135–150. doi: 10.1080/002689797170707
Blas, F. J., and Vega, L. F. (1998). Prediction of binary and ternary diagrams using the statistical associating fluid theory (SAFT) equation of state. Ind. Eng. Chem. Res. 37, 660–674. doi: 10.1021/ie970449
Boot-Handford, M. E., Abanades, J. C., Anthony, E. J., Blunt, M. J., Brandani, S., Mac Dowell, N., et al. (2014). Carbon capture and storage. Energy Environ. Sci. 7, 130–189. doi: 10.1039/c3ee42350f
Budzianowski, W. M. (2015). Single solvents, solvent blends, and advanced solvent systems in CO2 capture by absorption: a review. Int. J. Glob. Warm. 7, 184–225. doi: 10.1504/ijgw.2015.067749
Budzianowski, W. M. (2016). Explorative analysis of advanced solvent processes for energy efficient carbon dioxide capture by gas - liquid absorption. Int. J. Greenh. Gas Control 49, 108–120. doi: 10.1016/j.ijggc.2016.02.028
Builes, S., Roussel, T., Ghimbeu, C. M., Parmentier, J., Gadiou, R., Vix-Guterl, C., et al. (2011). Microporous carbon adsorbents with high CO2 capacities for industrial applications. PCCP 13, 16063–16070. doi: 10.1039/c1cp21673b
Caplow, M. (1968). Kinetics of carbamate formation and breakdown. J. Am. Chem. Soc. 90, 6795–6803. doi: 10.1021/ja01026a041
Cavenati, S., Grande, C. A., and Rodrigues, A. E. (2006). Separation of CH4/CO2/N2 mixtures by layered pressure swing adsorption for upgrade of natural gas. Chem. Eng. Sci. 61, 3893–3906. doi: 10.1016/j.ces.2006.01.023
Chapman, W. G., Gubbins, K. E., Jackson, G., and Radosz, M. (1989). SAFT: equation-of-state solution model for associating fluids. Fluid Phase Equilib. 52, 31–38. doi: 10.1016/0378-3812(89)80308-5
Chapman, W. G., Gubbins, K. E., Jackson, G., and Radosz, M. (1990). New reference equation of state for associating liquids. Ind. Eng. Chem. Res. 29, 1709–1721. doi: 10.1021/ie00104a021
Dancwerts, P. V. (1978). The reactions of CO2 with ethanoamines. Chem. Eng. Sci. 34, 443–446. doi: 10.1016/0009-2509(79)85087-3
Deng, W.-Q., Xu, X., and Goddard, W. A. (2004). New alkali doped pillared carbon materials designed to achieve practical reversible hydrogen storage for transportation. Phys. Rev. Lett. 92:166103. doi: 10.1103/physrevlett.92.166103
Dias, A. M. A., Carrier, H., Daridon, J. L., Pàmies, J. C., Vega, L. F., Coutinho, J. A. P., et al. (2006). Vapor-liquid equilibrium of carbon dioxide-perfluoroalkane mixtures: experimental data and SAFT modeling. Ind. Eng. Chem. Res. 45, 2341–2350. doi: 10.1021/ie051017z
Dunstan, M. T., Jain, A., Liu, W., Ong, S. P., Liu, T., Lee, J., et al. (2016). Large scale computational screening and experimental discovery of novel materials for high temperature CO2 capture. Energy Environ. Sci. 9, 1346–1360. doi: 10.1039/c5ee03253a
Duque, D., Pàmies, J. C., and Vega, L. F. (2004). Interfacial properties of lennard-jones chains by direct simulation and density gradient theory. J. Chem. Phys. 121, 11395–11401. doi: 10.1063/1.1818679
Ferreira, M. L., Araújo, J. M. M., Pereiro, A. B., and Vega, L. F. (2019). Insights into the influence of the molecular structure of Fluorinated Ionic Liquids on their thermophysical properties. A soft-SAFT based approach. PCCP 21, 6362–6380. doi: 10.1039/C8CP07522K
Frenkel, D., and Smit, B. (2002). Understanding Molecular Simulation, 2nd Edn, San Diego, CA: Academic Press.
Frost, H., Duren, T., and Snurr, R. Q. (2006). Effects of surface area, free volume, and heat of adsorption on hydrogen uptake in metal-organic frameworks. J. Phys. Chem. B 110, 9565–9570. doi: 10.1021/jp060433
Garcia, E. J., Perez-Pellitero, J., Pirngruber, G. D., and Jallut, C. (2017). Sketching a portrait of the optimal adsorbent for CO2 separation by pressure swing adsorption. Ind. Eng. Chem. Res. 56, 4818–4829. doi: 10.1021/acs.iecr.6b04877
Gil-Villegas, A., Galindo, A., Whitehead, P. J., Mills, S. J., Jackson, G., and Burgess, A. N. (1997). Statistical associating fluid theory for chain molecules with attractive potentials of variable range. J. Chem. Phys. 106, 4168–4186. doi: 10.1063/1.473101
Grande, C. A., and Rodrigues, A. E. (2008). Electric swing adsorption for CO2 removal from flue gases. Int. J. Greenhouse Gas Control 2, 194–202. doi: 10.1016/s1750-5836(07)00116-8
Gross, J., and Sadowski, G. (2001). Perturbed-chain saft:an equation of state based on a perturbation theory for chain molecules. Ind. Eng. Chem. Res. 40, 1244–1260. doi: 10.1021/ie0003887
Gubbins, K. E., and Twu, C. H. (1978). Thermodynamics of polyatomic fluid mixtures - I theory. Chem. Eng. Sci. 33, 863–878. doi: 10.1016/0009-2509(78)85176-8
Hamborg, E. S., Derks, P. W. J., Van Elk, E. P., and Versteeg, G. F. (2011). Carbon dioxide removal by alkanolamines in aqueous organic solvents. A method for enhancing the desorption process. Energy Proc. 4, 187–194. doi: 10.1016/j.egypro.2011.01.040
Hedin, N., Andersson, L., Bergström, L., and Yan, J. (2013). Adsorbents for the post-combustion capture of CO2 using rapid temperature swing or vacuum swing adsorption. Appl. Energy 104, 418–433. doi: 10.1016/j.apenergy.2012.11.034
Heldebrant, D. J., Koech, P. K., Glezakou, V., Rousseau, R., Malhotra, D., and Cantu, D. C. (2017). Water-lean solvents for post-combustion CO2 Capture: fundamentals, uncertainties, opportunities, and outlook. Chem. Rev. 117, 9594–9624. doi: 10.1021/acs.chemrev.6b00768
Herdes, C., Valente, A., Lin, Z., Rocha, J., Coutinho, J. A. P., Medina, F., et al. (2007). Selective adsorption of volatile organic compounds in micropore aluminum methylphosphonate-α: a combined molecular simulation-experimental approach. Langmuir 23, 7299–7305. doi: 10.1021/la063518a
Huang, H., Zhang, W., Liu, D., and Zhong, C. (2012). Understanding the effect of trace amount of water on CO2 capture in natural gas upgrading in metal-organic frameworks: a molecular simulation study. Ind. Eng. Chem. Res. 51, 10031–10038. doi: 10.1021/ie202699r
Huang, S. H., and Radosz, M. (1990). Equation of state for small, large, polydisperse, and associating molecules. Ind. Eng. Chem. Res. 29, 2284–2294. doi: 10.1021/ie00107a014
Huck, J. M., Lin, L.-C., Berger, A. H., Shahrak, M. N., Martin, R. L., Bhown, A. S., et al. (2014). Evaluating different classes of porous materials for carbon capture. Energy Environ. Sci. 7, 4132–4146. doi: 10.1039/c4ee02636e
Intergovernmental Panel on Climate Change [IPCC] (2014). “Climate change 2014: synthesis report,” in Contribution of Working Groups I, II and III to the Fifth Assessment Report of the Intergovernmental Panel on Climate Change, eds Core Writing Team, R. K. Pachauri, and L. A. Meyer (Geneva: IPCC).
International Energy Agency [IEA] (2018). Technology Roadmap - Low-Carbon Transition in the Cement Industry. Paris: International Energy Agency.
International Energy Agency [IEA] (2019). Technology Report - Transforming Industry through CCUS. Paris: International Energy Agency.
Jog, P. K., Sauer, S. G., Blaesing, J., and Chapman, W. G. (2001). Application of dipolar chain theory to the phase behavior of polar fluids and mixtures. Ind. Eng. Chem. Res. 40, 4641–4648. doi: 10.1021/ie010264
Johnson, J. K., Zollweg, J. A., and Gubbins, K. E. (1992). The lennard-jones equation of state revisited. J. Mol. Phys. 78, 591–618. doi: 10.1080/00268979300100411
Jorgensen, W. L., and Tirado-Rives, J. (1988). The OPLS [optimized potentials for liquid simulations] potential functions for proteins, energy minimizations for crystals of cyclic peptides and crambin. J. Am. Chem. Soc. 110, 1657–1666. doi: 10.1021/ja00214a001
Joss, L., Gazzani, M., and Mazzotti, M. (2017). Rational design of temperature swing adsorption cycles for post-combustion CO2 capture. Chem. Eng. Sci. 158, 381–394. doi: 10.1016/j.ces.2016.10.013
Khurana, M., and Farooq, S. (2016). Adsorbent screening for postcombustion CO2 capture: a method relating equilibrium isotherm characteristics to an optimum vacuum swing adsorption process performance. Ind. Eng. Chem. Res. 55, 2447–2460. doi: 10.1021/acs.iecr.5b04531
Kim, H., Hwang, S. J., and Lee, K. S. (2015). Novel shortcut estimation method for regeneration energy of amine solvents in an absorption-based carbon capture process. Environ. Sci. Technol. 49, 1478–1485. doi: 10.1021/es504684x
Kohl, A. L., and Nielsen, R. B. (1997). Gas Purification, 5th Edn, Houston: Gulf Professional Publishing.
Krishna, R., and van Baten, J. M. (2012). A comparison of the CO2 capture characteristics of zeolites and metal-organic frameworks. Sep. Purif. Technol. 87, 120–126. doi: 10.1016/j.seppur.2011.11.031
Kumar, R., Huggahalli, M., Deng, S., and Andrecovich, M. (2003). Trace impurity removal from air. Adsorption 9, 243–250. doi: 10.1023/A:1024701917647
Lafitte, T., Apostolakou, A., Avendaño, C., Galindo, A., Adjiman, C. S., Müller, E. A., et al. (2013). Accurate statistical associating fluid theory for chain molecules formed from Mie segments. J. Chem Phys. 139, 154504. doi: 10.1063/1.4819786
Le Quéré, C., Korsbakken, J. I., Wilson, C., Tosun, J., Andrew, R., Andres, R. J., et al. (2019). Drivers of declining CO2 emissions in 18 developed economies. Nat. Clim. Chang. 9, 213–217. doi: 10.1038/s41558-019-0419-7
Lee, J.-Y., Keener, T. C., and Yang, Y. J. (2009). Potential flue gas impurities in carbon dioxide streams separated from coal-fired power plants. J. Air Waste Manag. Assoc. 59, 725–732. doi: 10.3155/1047-3289.59.6.725
Li, G., Xiao, P., Webley, P. A., Zhang, J., Singh, R., and Marshall, M. (2008). Capture of CO2 from high humidity flue gas by vacuum swing adsorption with zeolite 13X. Adsorption 14, 415–422. doi: 10.1007/s10450-007-9100-y
Li, J.-R., Ma, Y., McCarthy, M. C., Sculley, J., Yu, J., Jeong, H.-K., et al. (2011). Carbon dioxide capture-related gas adsorption and separation in metal-organic frameworks. Coord. Chem. Rev. 255, 1791–1823. doi: 10.1016/j.ccr.2011.02.012
Li, K., Leigh, W., Feron, P., Yu, H., and Tade, M. (2016). Systematic study of aqueous mono-ethanolamine (MEA)-based CO2 capture process: techno-economic assessment of the MEA process and its improvements. Appl. Energy 165, 648–659. doi: 10.1016/j.apenergy.2015.12.109
Liang, Z., Rongwong, W., Liu, H., Fu, K., Gao, H., Cao, F., et al. (2015). Recent progress and new developments in post-combustion carbon-capture technology with amine based solvents. Int. J. Greenh. Gas Control 40, 26–54. doi: 10.1016/j.ijggc.2015.06.017
Lin, L. C., Berger, A. H., Martin, R. L., Kim, J., Swisher, J. A., Jariwala, K., et al. (2012). In silico screening of carbon-capture materials. Nat. Mater. 11, 633–641. doi: 10.1038/nmat3336
Liu, Y., Liu, J., Lin, Y. S., and Chang, M. (2014). Effects of water vapor and trace gas impurities in flue gas on CO2/N2 separation using ZIF-68. J Phys. Chem. C 118, 6744–6751. doi: 10.1021/jp4113969
Lloret, J. O., Vega, L. F., and Llovell, F. (2017). A consistent and transferable thermodynamic model to accurately describe CO2 capture with monoethanolamine. J. CO2 Util. 21, 521–533. doi: 10.1016/j.jcou.2017.08.018
Llovell, F., Marcos, R. M., and Vega, L. F. (2013). Free-volume theory coupled with soft-SAFT for viscosity calculations: comparison with molecular simulation and experimental data. J. Phys. Chem. B 117, 8159–8171. doi: 10.1021/jp401307t
Lopez-Aranguren, P., Builes, S., Fraile, J., Lopez-Periago, A., Vega, L. F., and Domingo, C. (2015). Hybrid aminopolymer-silica materials for efficient CO2 adsorption. RSC Adv. 5, 104943–104953. doi: 10.1039/c5ra20583b
MacDowell, N., Florin, N., Buchard, A., Hallett, J., Galindo, A., Jackson, G., et al. (2010). An overview of CO2 capture technologies. Energy Environ. Sci. 3, 1645–1669. doi: 10.1039/C004106H
Maring, B. J., and Webley, P. A. (2013). A new simplified pressure/vacuum swing adsorption model for rapid adsorbent screening for CO2 capture applications. Int. J. Greenhouse Gas Control 15, 16–31. doi: 10.1016/j.ijggc.2013.01.009
Martin-Calvo, A., Gutiérrez-Sevillano, J. J., Parra, J. B., Ania, C. O., and Calero, S. (2015). Transferable force fields for adsorption of small gases in zeolites. PCCP 17, 24048–24055. doi: 10.1039/c5cp03749b
Mello, M., and Eiü, M. (2002). Adsorption of sulfur dioxide from pseudo binary mixtures on hydrophobic zeolites: modelling of breakthrough curves. Adsorption 8, 279–289. doi: 10.1023/a:1021581312948
Mota-Martinez, M. T., Hallett, J. P., and Mac Dowell, N. (2017). Solvent selection and design for CO2 capture-how we might have been missing the point. Sustain. Energ. Fuels 1, 2078–2090. doi: 10.1039/C7SE00404D
National Oceanic and Atmospheric Administration [NOAA] (2019). U.S. Department of Commerce. NOAA: Silver Spring, MD.
Ntiamoah, A., Ling, J., Xiao, P., Webley, P. A., and Zhai, Y. (2016). CO2 capture by temperature swing adsorption: use of hot co2-rich gas for regeneration. Ind. Eng. Chem. Res. 55, 703–713. doi: 10.1021/acs.iecr.5b01384
Olson, D. H. (1995). The crystal structure of dehydrated NaX. Zeolites 15, 439–443. doi: 10.1016/0144-2449(95)00029-6
Oyenekan, B. A., and Rochelle, G. T. (2007). Alternative stripper configurations for CO2 capture by aqueous amines. AICHE J. 53, 3144–3154. doi: 10.1002/aic.11316
Pàmies, J. C., and Vega, L. F. (2001). Vapor-liquid equilibria and critical behavior of heavy n-alkanes using transferable parameters from the soft-SAFT equation of state. Ind. Eng. Chem. Res. 40, 2532–2543. doi: 10.1021/ie000944x
Park, S. W., Lee, J. W., and Sik, B. (2005). Reaction kinetics of carbon dioxide with diethanol amine in polar organic solvents. Sci. Technol. 40, 1885–1898. doi: 10.1081/SS-200064536
Pedrosa, N., Pámies, J. C., Coutinho, J. A. P., Marrucho, I. M., and Vega, L. F. (2005). Phase equilibria of ethylene glycol oligomers and their mixtures. Ind. Eng. Chem. Res. 44, 7027–7037. doi: 10.1021/ie050361t
Pereira, L. M. C., Llovell, F., and Vega, L. F. (2018). Thermodynamic characterisation of aqueous alkanolamine and amine solutions for acid gas processing by transferable molecular models. Appl. Energy 222, 687–703. doi: 10.1016/j.apenergy.2018.04.021
Pereira, L. M. C., and Vega, L. F. (2018). A systematic approach for the thermodynamic modelling of CO2-amine absorption process using molecular-based models. Appl. Energy 232, 273–291. doi: 10.1016/j.apenergy.2018.09.189
Plaza, M. G., García, S., Rubiera, F., Pis, J. J., and Pevida, C. (2010). Post-combustion CO2 capture with a commercial activated carbon: comparison of different regeneration strategies. Chem. Eng. J. 163, 41–47. doi: 10.1016/j.cej.2010.07.030
Plaza, M. G., Rubiera, F., and Pevida, C. (2017). Evaluating the feasibility of a TSA process based on steam stripping in combination with structured carbon adsorbents to capture CO2 from a coal power plant. Energy Fuels 31, 9760–9775. doi: 10.1021/acs.energyfuels.7b01508
Plimpton, S. (1995). Fast parallel algorithms for short-range molecular dynamics. J. Comp. Phys. 117, 1–19. doi: 10.1006/jcph.1995.1039
Potoff, J. J., and Siepmann, J. I. (2001). Vapor-liquid equilibria of mixtures containing alkanes, carbon dioxide, and nitrogen. AICHE J. 47, 1676–1682. doi: 10.1002/aic.690470719
Prats, H., Bahamon, D., Alonso, G., Giménez, X., Gamallo, P., and Sayós, R. (2017). Optimal Faujasite structures for post combustion CO2 capture and separation in different swing adsorption processes. J. CO2 Util. 19, 100–111. doi: 10.1016/j.jcou.2017.03.007
Prats, H., Bahamon, D., Giménez, X., Gamallo, P., and Sayós, R. (2017). Computational simulation study of the influence of faujasite Si/Al ratio on CO2 capture by temperature swing adsorption. J. CO2 Util. 21, 261–269. doi: 10.1016/j.jcou.2017.07.013
Puxty, G., and Maeder, M. (2016). “2-The fundamentals of post-combustion capture,” in Absorption-Based Post-Combustion Capture of Carbon Dioxide, ed. P. H. M. Feron (Sawston: Woodhead Publishing), 13–33.
Queen, W. L., Hudson, M. R., Bloch, E. D., Mason, J. A., Gonzalez, M. I., Lee, J. S., et al. (2014). Comprehensive study of carbon dioxide adsorption in the metal-organic frameworks M2(dobdc) (M = Mg, Mn, Fe, Co, Ni, Cu, Zn). Chem. Sci. 5, 4569–4581. doi: 10.1039/c4sc02064b
Radosz, M., Hu, X., Krutkramelis, K., and Shen, Y. (2008). Flue-gas carbon capture on carbonaceous sorbents:toward a low-cost multifunctional carbon filter for “green” energy producers. Ind. Eng. Chem. Res. 47, 3783–3794. doi: 10.1021/ie0707974
Rao, A. B., and Rubin, E. A. (2002). A technical, economic, and environmental assessment of amine-based CO2 capture technology for power plant greenhouse gas control. Environ. Sci. Technol. 36, 4467–4475. doi: 10.1021/es0158861
Rivas, O. R., and Prausnitz, J. M. (1979). Sweetening of sour natural gases by mixed-solvent ab-sorption: solubilities of ethane, carbon dioxide, and hydrogen chemical solvents. AICHE J. 25, 975–984. doi: 10.1002/aic.690250608
Rochelle, G. T. (2009). Amine scrubbing for CO2 capture. Science 325, 1652–1654. doi: 10.1126/science.1176731
Santer, B. D., Wehner, M. F., Wigley, T. M. L., Sausen, R., Meehl, G. A., Taylor, K. E., et al. (2003). Contributions of anthropogenic and natural forcing to recent tropopause height changes. Science 301:479. doi: 10.1126/science.1084123
Serbezov, A. (2003). Adsorption equilibrium of water vapor on F-200 activated alumina. J. Chem. Eng. Data 48, 421–425. doi: 10.1021/je025616d
Sinha, A., Darunte, L. A., Jones, C. W., Real, M. J., and Kawajiri, Y. (2017). Systems design and economic analysis of direct air capture of CO2 through temperature vacuum swing adsorption using MIL-101(Cr)-PEI-800 and mmen-Mg2(dobpdc) MOF adsorbents. Ind. Eng. Chem. Res. 56, 750–764. doi: 10.1021/acs.iecr.6b03887
Smit, B., and Maesen, T. L. M. (2008). Molecular simulations of zeolites: adsorption, diffusion, and shape selectivity. Chem. Rev. 108, 4125–4184. doi: 10.1021/cr8002642
Sreedhar, I., Nahar, T., Venugopal, A., and Srinivas, B. (2017). Carbon capture by absorption - path covered and ahead. Renew. Sust. Energ. Rev. 76, 1080–1107. doi: 10.1016/j.rser.2017.03.109
Steffen, W., Richardson, K., Rockstrom, J., Cornell, S. E., Fetzer, I., Bennett, E. M., et al. (2015). Planetary boundaries: guiding human development on a changing planet. Science 347:1259855. doi: 10.1126/science.1259855
Suleman, H., Maulud, A. S., and Man, Z. (2015). Review and selection criteria of classical thermodynamic models for acid gas absorption in aqueous alkanolamines. Int. Rev. Chem. Eng. 31, 599–639. doi: 10.1515/revce-2015-0030
Sumida, K., Rogow, D. L., Mason, J. A., McDonald, T. M., Bloch, E. D., Herm, Z. R., et al. (2012). Carbon dioxide capture in metal-organic frameworks. Chem. Rev. 112, 724–781. doi: 10.1021/cr2003272
Supap, T., Idem, R., Tontiwachwuthikul, P., and Saiwan, C. (2006). Analysis of monoethanolamine and its oxidative degradation products during CO2 absorption from flue gases: a comparative study of GC-MS, HPLC-RID, and CE-DAD analytical techniques and possible optimum combinations. Ind. Eng. Chem. Res. 45, 2437–2451. doi: 10.1021/ie050559d
Svendsen, H. F., Hessen, E. T., and Mejdell, T. (2011). Carbon dioxide capture by absorption, challenges and possibilities. Chem. Eng. J. 171, 718–724. doi: 10.1016/j.cej.2011.01.014
Tan, S. P., Adidharma, H., and Radosz, M. (2008). Recent advances and applications of statistical associating fluid theory. Ind. Eng. Chem. Res. 47, 8063–8082. doi: 10.1021/ie8008764
Twu, C. H., and Gubbins, K. E. (1978). Thermodynamics of polyatomic fluid mixtures—II: polar, quadrupolar and octopolar molecules. Chem. Eng. Sci. 33, 879–887. doi: 10.1016/0009-2509(78)85177-X
Vega, F., Cano, M., Camino, S., Gallego Fernandez, L. M., Portillo, E., and Navarrete, B. (2019). Solvents for carbon dioxide capture solvents. Carbon Dioxide Chem. Capt. Oil Recov. 1, 141–163.
Vega, L. F. (2010). “CO2 as a resource. From capture to industrial uses,” El CO2 Como Recurso: de la Captura a los Usos Industriales (Madrid: Naturgy). Available online at: https://www.fundacionnaturgy.org/en/product/co2-recurso-la-captura-los-usos-industriales/
Vega, L. F., and Bahamon, D. (2016). “Comparative study of MOFs and Zeolites for CO2 capture and separation at process conditions,” in Proceedings of the 2016 AIChE Annual Meeting, San Francisco, CA.
Vega, L. F., Llovell, F., and Blas, F. J. (2009). Capturing the solubility minima of n-Alkanes in water by Soft-SAFT. J. Phys. Chem. B 113, 7621–7630. doi: 10.1021/jp9018876
Vilaseca, O., Llovell, F., Yustos, J., Marcos, R. M., and Vega, L. F. (2010). Phase equilibria, surface tensions and heat capacities of hydrofluorocarbons and their mixtures including the critical region. J. Supercrit. Fluids 55, 755–768. doi: 10.1016/j.supflu.2010.10.015
Vilaseca, O., and Vega, L. F. (2011). Direct calculation of interfacial properties of fluids close to the critical region by a molecular-based equation of state. Fluid Phase Equilib. 306, 4–14. doi: 10.1016/j.fluid.2010.09.018
Vujic, B., and Lyubartsev, A. P. (2017). Computationally based analysis of the energy efficiency of a CO2 capture process. Chem. Eng. Sci. 174, 174–188. doi: 10.1016/j.ces.2017.09.006
Wang, L., Liu, Z., Li, P., Yu, J., and Rodrigues, A. E. (2012). Experimental and modeling investigation on post-combustion carbon dioxide capture using zeolite 13X-APG by hybrid VTSA process. Chem. Eng. J. 197, 151–161. doi: 10.1016/j.cej.2012.05.017
Wertheim, M. S. (1984a). Fluids with highly directional attractive forces. I. Statistical thermodynamics. J. Stat. Phys. 35, 19–34. doi: 10.1007/bf01017362
Wertheim, M. S. (1984b). Fluids with highly directional attractive forces. II. Thermodynamic perturbation theory and integral equations. J. Stat. Phys. 35, 35–47. doi: 10.1007/bf01017363
Wertheim, M. S. (1986a). Fluids with highly directional attractive forces. III. Multiple attraction sites. J. Stat. Phys. 42, 459–476. doi: 10.1007/bf01127721
Wertheim, M. S. (1986b). Fluids with highly directional attractive forces. IV. Equilibrium polymerization. J. Stat. Phys. 42, 477–492. doi: 10.1007/bf01127722
Wilmer, C. E., Farha, O. K., Bae, Y. S., Hupp, J. T., and Snurr, R. Q. (2012). Structure-property relationships of porous materials for carbon dioxide separation and capture. Energy Environ. Sci. 5, 9849–9856. doi: 10.1039/c2ee23201d
Xiang, S., He, Y., Zhang, Z., Wu, H., Zhou, W., Krishna, R., et al. (2012). Microporous metal-organic framework with potential for carbon dioxide capture at ambient conditions. Nat. Commun. 3:954. doi: 10.1038/ncomms1956
Yaghi, O. M., O’Keeffe, M., Ockwig, N. W., Chae, H. K., Eddaoudi, M., and Kim, J. (2003). Reticular synthesis and the design of new materials. Nature 423, 705–714. doi: 10.1038/nature01650
Zanco, S. E., Mazzotti, M., Gazzani, M., Romano, M. C., and Martínez, I. (2018). Modeling of circulating fluidized beds systems for post-combustion CO2 capture via temperature swing adsorption. AICHE J. 64, 1744–1759. doi: 10.1002/aic.16029
Zhang, G., Yang, Y., Xu, G., Zhang, K., and Zhang, D. (2015). CO2 capture by chemical absorption in coal-fired power plants: energy-saving mechanism, proposed methods, and performance analysis. Int. J. Greenh. Gas Control 39, 449–462. doi: 10.1016/j.ijggc.2015.06.006
Zhang, J., Xiao, P., Li, G., and Webley, P. A. (2009). Effect of flue gas impurities on CO2 capture performance from flue gas at coal-fired power stations by vacuum swing adsorption. Energy Procedia 1, 1115–1122. doi: 10.1016/j.egypro.2009.01.147
Zhang, T., Yu, Y., and Zhang, Z. (2018). Effects of non-aqueous solvents on CO2 absorption in monoethanolamine: Ab initio calculations. Mol. Simul. 7022, 1–11. doi: 10.1080/08927022.2018.1455004
Zhang, Y. Y., Ji, X. Y., and Lu, X. H. (2014). Energy consumption analysis for CO2 separation from gas mixtures. Appl. Energy 61, 2695–2698. doi: 10.1016/j.apenergy.2014.05.057
Keywords: CO2 capture, absorption, water-free amines, adsorption, metal–organic frameworks, soft-SAFT, molecular simulations
Citation: Bahamon D, Alkhatib III, Alkhatib N, Builes S, Sinnokrot M and Vega LF (2020) A Comparative Assessment of Emerging Solvents and Adsorbents for Mitigating CO2 Emissions From the Industrial Sector by Using Molecular Modeling Tools. Front. Energy Res. 8:165. doi: 10.3389/fenrg.2020.00165
Received: 31 March 2020; Accepted: 29 June 2020;
Published: 28 July 2020.
Edited by:
Carlo Giorgio Visconti, Politecnico di Milano, ItalyReviewed by:
Nicolaas Vermeulen, Northwestern University, PhilippinesWei Liu, Molecule Works Inc., United States
Copyright © 2020 Bahamon, Alkhatib, Alkhatib, Builes, Sinnokrot and Vega. This is an open-access article distributed under the terms of the Creative Commons Attribution License (CC BY). The use, distribution or reproduction in other forums is permitted, provided the original author(s) and the copyright owner(s) are credited and that the original publication in this journal is cited, in accordance with accepted academic practice. No use, distribution or reproduction is permitted which does not comply with these terms.
*Correspondence: Lourdes F. Vega, bG91cmRlcy52ZWdhQGt1LmFjLmFl; bG91cmRlcy52ZWdhQGdtYWlsLmNvbQ==