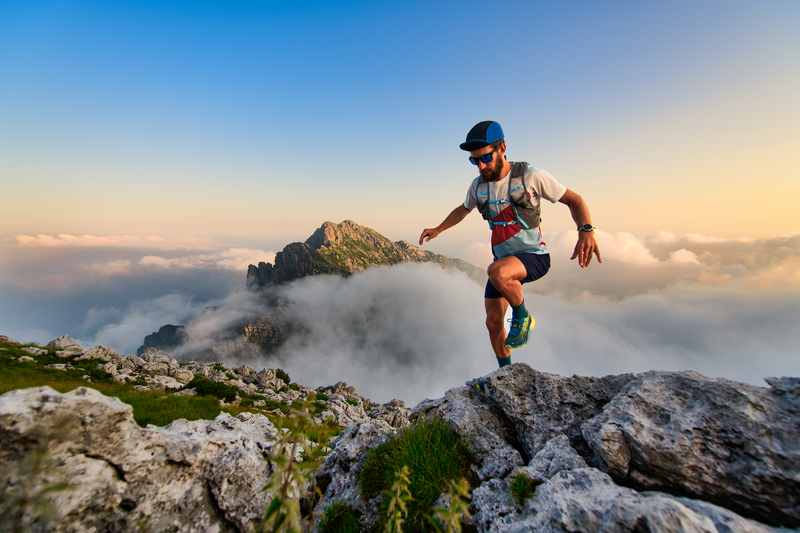
95% of researchers rate our articles as excellent or good
Learn more about the work of our research integrity team to safeguard the quality of each article we publish.
Find out more
REVIEW article
Front. Energy Res. , 17 July 2020
Sec. Bioenergy and Biofuels
Volume 8 - 2020 | https://doi.org/10.3389/fenrg.2020.00144
This article is part of the Research Topic Nanocatalysts in Biofuel Process Optimization View all 13 articles
For the last two decades, the biodiesel attracted increasing attention as a promising biofuel to replace fossil diesel. However, the non-recyclability of homogeneous alkali catalysts and waste generation due to subsequent water washing remained as one of the major drawbacks of the biodiesel production process in the industry. Ionic liquids are one of the best alternatives to replace alkali catalysts owing to their unique properties such as non-volatility, excellent solubility for various organic and inorganic materials, structure tunability, environment-friendliness, and wide liquid temperature. However, high viscosity and difficult recovery have limited their application. Recently, heterogenization of ionic liquids on solid supports has been proposed to circumvent these issues. Among these solids, nanoporous materials have shown great potential in providing stable supports with high porosity and specific surface area. This paper reviews the recent developments in designing ionic liquids deposited on nanoporous materials as catalysts for biodiesel production. The emphasis was on the application of this type of catalysts for the optimization of reaction conditions. Moreover, challenges and opportunities for improving the overall production process in the presence of these catalysts were discussed. Despite that high biodiesel yields were obtained over many of nanoporous material-supported ionic liquids, their significantly higher cost compared to the conventional catalysts remained a major challenge. This issue can be overcome by employing less expensive cations and anions, increasing the loading amount of ionic liquids, and improving catalyst reusability in future studies.
Nowadays, due to the non-renewability of fossil fuels and their negative environmental impacts, the necessity of finding alternative energy sources is well-established. With this respect, renewable energy sources have been considered as one of the most promising options for the last few decades, as they are cleaner and more environment-friendly and can contribute to the sustainable development of societies (Dincer, 2000; Panwar et al., 2011).
As the most common renewable energy resource, biomass has a significant contribution to the world economy and provides a range of biofuels and chemicals (Chum and Overend, 2001; McKendry, 2002; Dodds and Gross, 2007). In 2017, nearly 11% of the world's energy demand was supplied by biomass. However, contributing to 65% of the world's total final consumption, oil has remained as the main energy source in the transportation sector (International Energy Agency, 2019). This necessitates more production and incorporation of biofuels, especially in the transportation fleet, to reduce dependence on petroleum and its negative impacts on the environment.
Biodiesel is one of the popular forms of biofuel which is obtained from vegetable oils and animal fats and is considered as an attractive alternative to fossil diesel (Van Gerpen, 2005). It is renewable, non-toxic, biodegradable, sulfur, and benzene-free, can be used in common diesel engines with no modification, and its blending with fossil diesel at any given ratio is possible (Demirbas, 2006; Nabi et al., 2006; Khan et al., 2009; Kumar et al., 2010; Mekhilef et al., 2011). Owing to these benefits, 36 billion liters of biodiesel were produced around the globe in 2017. This figure is expected to rise by 9% through 2027 (OECD/FA, 2018).
Despite paramount advantages, biodiesel has some technical, economic, and environmental drawbacks. Compared to fossil diesel, biodiesel presents weaker oxidative stability and cold flow properties, higher viscosity and density, and higher NOx emissions (Monyem and Van Gerpen, 2001; Agarwal et al., 2006; Canakci, 2007; Tesfa et al., 2010). However, the most challenging disadvantage of biodiesel is its higher production cost in comparison with fossil diesel, which is mainly due to the high cost of feedstock and inefficient production processes (Hasheminejad et al., 2011; Yaakob et al., 2014; Ullah et al., 2015).
The most common and economically feasible method of biodiesel production at an industrial scale is the alkali-catalyzed transesterification of fresh vegetable oils (Gebremariam and Marchetti, 2018). However, over 80% of biodiesel production cost stems from employing fresh edible oils as feedstock (Mansir et al., 2018). Therefore, the best way to reduce the production cost of biodiesel is the use of alternative, cheaper sources of lipids such as waste cooking oil, non-edible Jatropha, algae, municipal sewage sludge, and recycled grease trap waste (Nagarajan et al., 2013; Yusuf and Kamarudin, 2013; Olkiewicz et al., 2016; Abdurakhman et al., 2018; Tran et al., 2018). Nevertheless, the high free fatty acid (FFA) content of these feedstocks results in saponification and emulsification in the presence of homogeneous base catalysts, which reduces biodiesel yield and hinders its separation from glycerol (Ghiaci et al., 2011; Alegria et al., 2014). Moreover, these catalysts are corrosive, difficult to be recovered and reused and are removed from the reaction mixture by water washing, which produces considerable amounts of wastewater (Takase et al., 2014; Lee et al., 2015; Sirisomboonchai et al., 2015).
One way to avoid the saponification problem is the use of homogeneous acids such as sulfuric acid and hydrochloric acid. These acids are tolerant of FFAs and esterify them into biodiesel, but they require higher temperature and pressure, a higher amount of alcohol, and longer reaction time to yield high biodiesel conversion (Su, 2013). Furthermore, corrosiveness, undesired byproducts, negative environmental impacts, the requirement of additional neutralization and separation processes, and difficult recovery have restrained the application of homogeneous acid catalysts at industrial scale (Han et al., 2013).
Ionic liquids (ILs) have recently emerged as one of the most promising options to replace conventional homogeneous catalysts. ILs are organic salts composed of cations and anions with melting points below 100°C (Liu C. Z. et al., 2012; Fang et al., 2014). They are nearly non-volatile, soluble in organic and inorganic materials, remarkably less toxic compared to the traditional organic solvents, possess wide liquids temperature and significant thermal and chemical stability (Fauzi and Amin, 2012; Muhammad et al., 2015). These unique materials are also recyclable and environmentally-friendly (Fan et al., 2013; Montalban et al., 2018; Gholami et al., 2019). More importantly, the properties of ILs can be altered and designed for a specific application by changing the type of cation or anion in their structure (Pandey, 2006; Luo et al., 2013). Owing to these interesting properties, ILs have been employed not only in catalysis but also as base materials and solvents in electrochemistry (Osada et al., 2016; Watanabe et al., 2017), extraction, and separation (Ventura et al., 2017; Berthod et al., 2018), biology and biotechnology (Egorova et al., 2017; Claus et al., 2018) and tribology (Amiril et al., 2017).
With regards to biodiesel production, ILs have been used as catalysts for the transesterification reaction, as cosolvents for stabilizing enzymes in biocatalyzed transesterification, and as solvents for extracting lipids from biomass, FFAs from oily feedstocks, and unsaturated esters and triacylglycerols from biodiesel (Troter et al., 2016). Employing ILs as novel catalysts for transesterification of triglycerides into biodiesel could lead to high yields with the less complex production process and reduces equipment corrosion, saponification, and waste generation (Ullah et al., 2018). Nevertheless, several drawbacks have hindered the widespread application of ionic liquids in the biodiesel industry. These include high cost, high viscosity, tedious recovery demanding expensive and energy-consuming processes, and large concentration in catalysis (Vallette et al., 2006; Bourbigou et al., 2010; Troter et al., 2016). The high cost of ILs can be outweighed by their overall benefits in many cases (Troter et al., 2016), but their high viscosity and inconvenient separation procedure remain a serious challenge for employing ILs in industrial plants.
To overcome the aforementioned problems, ILs can be heterogenized using solid materials. These supported ILs (SILs) provide the reaction catalysis with new opportunities, as they inherit the characteristics of both homogeneous and heterogeneous catalysts. Using SILs combines the possibility of facile separation and recovery of heterogeneous catalysts with the homogeneous media for reactants, the latter is provided by the IL layer on the solid surface. Moreover, fixed-bed reactors can be used for continuous production (Riisager et al., 2006a,b). Hence, many studies have investigated the utilization of SILs as catalysts for transesterification, in which a variety of solid carriers such as silica (Zhen et al., 2014), resin (Abreu et al., 2005), and polymers (Liang, 2013a,b) have been employed. However, these catalysts exhibit weak catalytic activities due to high mass-transfer resistance and low amount of ILs grafted on the surface of the carrier. The main reason for these drawbacks is attributed to the small specific surface area, low pore volume, wide-range pore distribution, and irregular pore shape of the conventional solid supports (Zhen et al., 2012; Zhang et al., 2013). Therefore, the search for more suitable solid supports for immobilization of ILs continues.
Nanoporous materials are of great potential in tackling the aforesaid issues associated with ILs heterogenization. They possess high surface area, uniform pore distribution, and a large number of active sites per unit surface area. In virtue of these characteristics, these solids have been used not only as novel supports for ILs (Selvam et al., 2012), but also as green catalysts for biofuel production (Fu et al., 2018; Sharma et al., 2018).
Regarding to the role of ILs, nanoporous materials or their combination in various processes, many valuable reviews can be found in the literature. However, no review has solely focused on the function of nanoporous materials as supports for ILs in biodiesel production. Consequently, there is a lack of information on the present status of this research area, such as different nanoporous supports, synthesis routes, and optimum reaction conditions. In this paper, we comprehensively discussed the recent developments in synthesizing ILs-nanoporous materials hybrid catalysts for biodiesel production. We mainly focused on the application of different types of these catalysts for the optimization of transesterification reaction conditions. The results from each category were compared together to highlight the most promising classes for future studies. Moreover, to put further light on the future pathways, challenges, and opportunities for improving the overall production process in terms of technical and economic aspects were discussed.
Nanoporous materials are a class of substances that contain nanoscale pores (Fu et al., 2018). According to the International Union of Pure and Applied Chemistry, these materials are divided into three groups based on their pore diameter: microporous (pore diameter under 2 nm), mesoporous (pore diameter between 2 and 50 nm) and macroporous (pore diameter above 50 nm) (Rouquerol et al., 1994). They have been widely used in industrial areas such as petroleum refining, detergents, medicinal applications, and separation since the dimensions of the pores are controllable (Valtchev et al., 2009).
To date a variety of nanoporous materials have been used to heterogenize ILs for transesterification catalysis. Figure 1 depicts the classification of these materials. In the majority of the researches that have been performed to date, silicon-based supports and polymers have been studies, followed by metal-organic frameworks (MOFs) and nanoporous carbon. Furthermore, in terms of pore size, mesoporous materials have attracted the most attention. On the other hand, only a few studies investigated the effect of micro and macroporous materials-supported ILs as catalysts for biodiesel production. This might be related to the interesting properties of mesoporous solids which will be discussed in the following sections.
Figure 1. Classification of nanoporous materials used as supports for ILs in (trans)esterification reaction (SBA, Santa Barbara Amorphous; PILs, Polymeric ionic liquids; MIL, Materiaux de l′Institute Lavoisier; HKUST, Hong Kong University of Science and Technology; UiO, Universitetet i Oslo).
Zeolites are the most common type of microporous silica-based materials. However, they are not very good candidates for being used as supports for ILs in biodiesel production. This can be ascribed to their small pore sizes which restrict the diffusion of large molecules like triglyceride to the active sites (Valtchev et al., 2009). As a result, instead of zeolites, Cao et al. (2016) focused on the immobilization of IL on microporous silica-gel. They prepared an acidic IL with 1-allyl-1H-imidazole, 1,3-propyl sulfonic acid lactone and H2SO4. This IL was then immobilized on thiol-group functionalized microporous silica-gel to fabricate a solid catalyst for biodiesel production from waste cooking oil (3-Mercaptopropyl) trimethoxysilane was employed to functionalize silica-gel with thiol groups. The resultant catalyst showed a high specific surface area (278.6 m2/g) and was not decomposed below 250°C. By using the catalyst dosage of 5 wt.% and methanol to waste cooking oil molar ratio of 25–1, the maximum yield of 87.58% was obtained over the catalyst at 60°C and reflux of methanol for 20 h. The reaction yield decreased to around 70% after the catalyst was reused for 5 times. This resulted from the leaching of IL from the surface of the silica-gel support.
The limited pore size of zeolites and other microporous silica materials led to the invention of mesoporous silica materials, which have unique properties such as well-defined and uniform pore size, significantly high surface area, large pore volume, and facile surface functionalization (Al Othman, 2012; Da'na, 2017). Owing to these properties, mesoporous silica-based materials have had the most contribution to the heterogenization of ILs for biodiesel production.
SBA-15 is a type of mesoporous silica-based material containing uniform hexagonal pores which have a tunable diameter of 5–15 nm. In addition to the general benefits of mesoporous solids, it is cheap, chemically inert, and thermodynamically stable. Moreover, it possesses narrow pore size distribution and various functional chemical groups could be grafted on its surface due to the presence of abundant active sites (Yuan et al., 2020).
Mesoporous materials based on silicon have one weakness in common: They possess a limited number of functional groups. This reduces the loading rate of IL as well as catalytic activity (Cheng et al., 2013; Liang, 2014). The functionalization of these materials by acidic groups turns them into an attractive heterogeneous catalyst for the esterification of carboxylic acids. However, one problem associated with the utilization of mesoporous silica in this reaction is its hydrophilic nature. This results in the deactivation of active sites in the presence of water, which is a by-product of the esterification reaction. To address this issue, Karimi and Vafaeezadeh (2012) confined the IL 1-methyl-3-octylimidazolium hydrogen sulfate ([MOIm]HSO4) inside the mesochannels of SBA-15-functionalized propylsulfonic acid by impregnation method. To this end, the solid support was impregnated with [MOIm]HSO4 in an acetone solution. The supported IL was obtained after stirring at room temperature for 3 h and evaporation of acetone under vacuum. The resultant catalyst was able to effectively catalyze the esterification of different carboxylic acids with ethanol at room temperature, in which at least 87% yield was obtained within 40 h. The high yield was attributed to the synergetic effect between the grafted sulfonic acid groups and the anions of IL. The incorporation of the hydrophobic IL bearing N-octyl group also improved the mass transfer rate and expelled the produced water out of the catalyst surface during the reaction.
Incorporation of metals into SBA-15 is another way to adjust its catalytic performance (Sasidharan et al., 2009). Zhang et al. (2012) synthesized an iron incorporated SBA-15 (Fe-SBA-15) and used it as a support for Bronsted acidic IL 1-(propyl-3-sulfonate)-3-(3-trimethoxysilylpropyl) imidazolium hydrogen sulfate ([SO3H-PIm-CPMS] [HSO4]). The IL was immobilized on the support by the impregnation method. First, Fe-SBA-15 was activated at 150°C for 10 h. Then both support and IL were added to toluene and the mixture was heated to reflux for 20 h under the N2 atmosphere. The final product was washed with diethyl ether and dried under vacuum. This catalyst was employed in esterification of oleic acid with methanol, where under the conditions of 5 wt.% catalyst dosage, methanol to oleic acid molar ratio of 6–1, a reaction time of 3 h and temperature of 90°C, the conversion of oleic acid reached to 87.7%. Compared to the case when IL was supported on SBA-15, the IL/Fe-SBA-15 showed a higher catalytic activity despite having less Bronsted acidic sites. This was attributed to the cooperative effect of Lewis and Bronsted acidic sites.
In another study conducted by Wang et al. (2018), three acidic ILs 1-(3-sulfonate)-propyl-3-allylimidazolium hydrogen sulfate, trifluoromethanesulfonate, and dihydrogen phosphotungstate were covalently immobilized on SBA-15 through thiol-ene reaction. To this end, SBA-15 was modified with KH-590 to obtain a thiol-group functionalized SBA-15 (SBA-15-SH). In the next step, IL was dissolved in methanol at around 40°C, and then SBA-15-SH was dispersed in the solution. To initiate the thiol-ene reaction, azobisisobutyronitrile was added and the mixture was heated at 60°C for 12 h. The produced solid was separated by filtration, washed with methanol and dried at 60°C under vacuum for 3 h. The supported 1-(3-sulfonate)-propyl-3-allylimidazolium dihydrogen phosphotungstate showed the best catalytic performance in the esterification of palmitic acid with methanol due to high acidity of phosphotungstic acid and higher loading rate compared to the other two catalysts. Another factor responsible for the higher yield for phosphotungstate based catalyst was its larger molecule, which resulted in remarkable stearic hinderance and more exposure of active sites to the reactants. The catalyst could be reused 5 times without a considerable decrease in ester yield, which demonstrates the high potential of thiol-ene reaction to produce supported ILs with high stability.
Generally, the conversion of triglycerides to methyl/ethyl esters can be achieved under milder reaction conditions when basic ILs are used (Gholami et al., 2019). Accordingly, the potential of SBA-15-supported basic IL 4-butyl-1,2,4-triazolium hydroxide in transesterification of soybean oil with methanol was investigated by Xie et al. (2015a). The catalyst was synthesized by grafting of 4-butyl-1-triethoxysilylpropyl-triazolium chloride onto the surface of SBA-15 through the formation of Si-O bonds. To this end, after dissolving the IL in anhydrous toluene and adding the SBA-15, the mixture was refluxed in the N2 atmosphere for 24 h. The mixture was allowed to cool down to room temperature, and then a solid was obtained by filtration, washing with acetone and drying under vacuum at 40°C for 3 h. The obtained solid was then extracted with diethyl ether and dichloromethane mixture for 6 h and was dissolved in a tetramethylammonium hydroxide solution in methanol. The solution was stirred at room temperature for 4 h, through which the chloride anions were exchanged to hydroxides. Finally, the catalyst was obtained after filtration, washing with ethanol and drying at 60°C under vacuum for 12 h. Employing this heterogeneous catalyst resulted in the conversion of 95.4% at optimum reaction conditions of 8 h, 65°C, methanol to oil molar ratio of 20–1 and catalyst concentration of 7 wt.%. After 4 catalytic cycles, the conversion remained over 84%.
The catalytic activity of basic ILs strongly depends on basicity. However, it should be noted that excessive loading of IL on the support results in decreased catalytic activity, as it reduces the specific surface area. Therefore, the amount of grafted IL on the support should be optimized. As an example, in another work by Xie et al. (2015b), the basic IL 1,3-dicyclohexyl-2-octylguanidine (DCOG) was anchored onto the SBA-15 by grafting method. In the first step, DCOG and 1,3-glycidyloxypropyl-trimethoxysilane were added to dry N,N-dimethylformamide and the mixture was stirred at room temperature for 48 h under N2 atmosphere to yield DCOG-organosilane. Afterwards, SBA-15 was dispersed in dry toluene and refluxed for 2 h with stirring under N2 atmosphere. The DCOG-organosilane containing solution was then added to SBA-15 and toluene solution at room temperature. The resultant mixture was refluxed with stirring in the N2 atmosphere for 24 h. The mixture was cooled down to room temperature and the solid product was filtered, washed with toluene and methanol, extracted with a diethyl ether and dichloromethane mixture at 70°C and dried under vacuum at 60°C for 12 h. By using 8 wt.% of this catalyst, the conversion of soybean oil reached 92.6% after 15 h. The catalytic activity increased when the loading amount of IL was lower than 2.5 g/g SBA-15, while a further increase of this figure did not show a significant effect on the catalytic performance.
The inclusion of organic functional into the structure of inorganic mesoporous silica materials leads to the formation of mesoporous organosilicas. These organic-inorganic hybrid mesoporous materials compensate the drawbacks of both siliceous mesoporous supports, i.e., limited number of functional groups, and organic carriers, i.e., low mechanical strength, weak thermal stability, and poor structure of the pore.
One problem associated with the use of SBA-15-supported ILs in the esterification reaction is the leaching of ILs from the surface of the support, which limits catalyst recoverability and reusability. Elhamifar et al. (2014) demonstrated that this issue can be overcome by covalent bonding of IL on mesoporous silica. Using this method, they supported 1-methyl-3-octylimidazolium hydrogen sulfate ([OMIm]HSO4) IL on sulfonic acid functionalized periodic mesoporous organosilica (PMO-IL-SO3H). After the preparation of PMO-IL, it was modified through grafting of (3-mercaptopropyl) trimethoxysilane. To this end, PMO-IL was added to dry toluene at room temperature under stirring for 5 min (3-mercaptopropyl) trimethoxysilane was then added to the solution and the mixture was refluxed under the argon atmosphere for 24 h. The resultant solid, PMO-IL-SH, was separated by filtration, washed with dry toluene and dried at 70°C for one night. In the second step, SH groups were oxidized to SO3H using H2O2. In this process, PMO-IL-SH was added to hydrogen peroxide and stirred at room temperature for 24 h. Afterwards, a dilute solution of sulfuric acid was added to the mixture under stirring for 30 min. PMO-IL-SO3H was obtained after filtering, washing with deionized water and ethanol and drying at 60°C for 12 h. The obtained catalyst was used in the esterification of various carboxylic acids with different alcohols, where the yield remained over 82%. The high efficiency was ascribed to the presence of imidazolium anion and sulfonic acid groups in the mesochannels of the carrier and their synergetic cooperation. They also stated that the nanostructure of PMO-IL with the imidazolium framework was responsible for the good stability of sulfonic acid sites.
Fan et al. (2018) prepared the acidic organosilica material /ZrO2-SiO2(Et) by a one-step co-condensation method and the subsequent hydrothermal treatment. Acidic IL sulfonic acid functionalized imidazolium hydrogen sulfate ([Ps-im] HSO4) was then immobilized on the acidic organosilica carrier by the chemical grafting. Figure 2 illustrates the four steps involved in the production process of the catalyst. First (3-chloropropy) triethoxysilane and /ZrO2-SiO2(Et) were dispersed in dry toluene. After stirring at 90°C for 24 h, the mixture was cooled, and /ZrO2-SiO2(Et)-(CH2)3-Cl was obtained by filtering, washing with toluene, dichloromethane and diethyl ether and drying at 100°C for 12 h. In the second step, this product along with imidazole was added to dry toluene, and /ZrO2-SiO2(Et)-im was produced using the same process as the previous step. In the next step, this product was dispersed in dry toluene and 1,3-propane sultone was added to the mixture. After undergoing the same process as the previous steps, /ZrO2-SiO2(Et)-Ps-im was obtained. Finally, this solid was suspended in dry dichloromethane and concentrated sulfuric acid (98%) was added dropwise at 0°C under vigorous stirring. The mixture was then stirred at room temperature for 24 h. After washing the resultant material with dichloromethane and diethyl ether and drying it at 100°C for 12 h, the catalyst [/ZrO2-SiO2(Et)-Ps-im]HSO4 was obtained. This catalyst was used to produce biodiesel from soybean oil, where under optimum reaction conditions of 5 wt.% catalyst concentration, the temperature of 150°C, methanol to oil molar ratio of 18–1, a 99% yield was obtained after 3 h. The hybrid support possessed stronger Bronsted and Lewis acid sites compared to /ZrO2, which was attributed to the improved hydrophobicity and porosity of the surface resulted from the incorporation of ethane-bridge organosilica moieties. Despite having lower acidity, the catalyst gave a higher yield as compared with the pure ([Ps-im] HSO4) owing to the synergetic impact of Bronsted and Lewis acid sites. The catalyst maintained its activity after 5 consecutive runs and exhibited excellent resistance to oleic acid and water.
Figure 2. The synthesis process of [/ZrO2-SiO2(Et)-Ps-im]HSO4 [Redrawn from Fan et al. (2018)].
Magnetic mesoporous silica is one of the most important classes of mesoporous materials that have been used as carriers for ILs. Magnetic mesoporous materials can combine the benefits of mesoporous and magnetic materials, and facilitate the separation of the catalyst from reaction mixture through a magnetic field. By immobilizing the acidic IL 1-Allyl-3-(butyl-4-sulfonyl) imidazolium trifluoromethanesulfonate ([BsAIm][OTf]) on magnetic mesoporous silica, Zhen et al. (2012) prepared a magnetic mesoporous silica-supported IL for esterification of oleic acid with methanol. Figure 3 illustrates the process for the synthesis of this catalyst. The magnetic carrier was synthesized by embedding the cobalt ferrite nanoparticles in silica using the sol-gel method and then modified with (3-mercaptopropyl) trimethoxysilane to add sulfhydryl groups to its structure. The IL was then supported on the modified magnetic mesoporous silica through a radical reaction between the allyl and sulfhydryl groups. After 12 h, the conversion of oleic acid reached 87% using a 10 wt.% catalyst dosage. They found that an increase in sulfhydryl loading of modified mesoporous silica resulted in a decrease in pore diameter, the amount of IL supported on the carrier, and conversion. Moreover, the catalyst did not show high stability and reusability due to the hydrolysis or alcoholysis of the Si-O bonds on the support under harsh reaction conditions 110°C.
Figure 3. Schematic of the synthesis process of [BsAIm][OTf]@SiO2/CoFe2O4 [Redrawn with modification from Zhen et al. (2012)].
The catalytic performance of mesoporous silica-based ILs could be even better than that of the ILs supported on nanoparticles. Zhang et al. (2013) prepared 1-Allyl-dodecyl imidazolium hydroxide ([ADIm][OH]) basic IL immobilized on magnetic mesoporous SiO2/CoFe2O4 nanoparticles (SCF) and magnetic CoFe2O4 nanoparticles (CF) and compared their catalytic activity in the transesterification of glycerol trioleate. For the synthesis of [ADIM][OH]/SCF, the carrier was first modified with 3-mercaptopropyltrimethoxysilane through thermal treatment at 110°C for 24 h. The modified SCF particles were washed with acetone and dried. In the next step, IL was supported on the carrier through the free radical reaction between allyl groups of IL and sulfhydryl groups of the carrier. To this end, [ADIm][Br] was first produced from the reaction between 1-allylimidazole and n-dodecyl bromide at 60°C for 24 h. After washing with diethyl ether and drying in vacuum, [ADIm][Br] and modified SCF were added to acetonitrile and the reaction was initiated by azobisisobutyronitrile at 100°C for 24 h. The produced solid [ADIm][Br]/SCF was washed with methanol and then was added into a flask along with NaOH and dichloromethane. The mixture was reacted at room temperature for 24 h and [ADIm][OH]/SCF was obtained after washing with water and ethanol and drying at 60°C. Both catalysts showed better catalytic performances than NaOH, with IL/SCF led to a slightly higher yield than CF. The mesoporous structure of SCF made it difficult for the large molecule of triglyceride to diffuse into the pores and reach the active sites of IL, so the reaction rate and yield were low at the beginning of the reaction. However, the long and narrow pores also prevented the triglyceride and intermediate diglyceride to escape from the surface of the carrier, thus providing longer contact opportunities for reactants and intermediate with catalytic sites. On the other hand, there were no pores on the surface of CF, and as a result, all the catalytic active sites in the bulk of IL/CF were easily accessible for reactants, increasing the reaction rate compared to IL/SCF at initial stages of transesterification. Nevertheless, because of the absence of pores and pore diffusion effects, triglyceride molecules were able to leave the catalytic sites freely, reducing the reaction yield at longer reaction times compared to the IL/SCF. The existence of mesopores and pore diffusion effect also provided the active sites with more protection and reduced their loss to some extent, due to which IL/SCF exhibited a higher yield in comparison to IL/CF after three cycles.
Wan et al. (2015a) employed Fe3O4 nanoparticles to fabricate a magnetic mesoporous silica material and used it as support for acidic IL 3-sulfopropyl-1-(3-propyltrimethoxysilane) imidazolium hydrogen sulfate ([SO3H-PIM-TMSP] HSO4). The Fe3O4 nanoparticles were produced by the solvothermal method. Then, they were coated with silica layer through hydrolysis and condensation of tetraethyl orthosilicate in the mixture of water, ethanol and ammonia, producing Fe3O4@SiO2 particles. Afterwards, these particles were coated with a composite layer consist of cetyltrimethylammonium bromide (CTAB) and silica by using tetraethyl orthosilicate and CTAB. After removing CTAB, magnetic mesoporous Fe3O4@SiO2@mSiO2 particles were obtained. In the next step, the IL was supported on the mesoporous silica by covalent immobilization, with Fe3O4 and mesoporous silica-based IL as core and shell, respectively. Typically, a mixture of IL and mesoporous carrier in dry toluene was prepared. The mixture was refluxed for 24 h under N2 atmosphere. Then, the produced material was washed with diethyl ether and dried under vacuum at 323 K for 8 h, yielding IL/Fe3O4@SiO2@mSiO2. In this catalyst, the function of non-porous inner silica layer was to protect the Fe3O4 nanoparticles, while the outer mesoporous layer acted as support. At optimum reaction conditions of 10.6 wt.% catalyst dosage, oleic acid to ethanol molar ratio of 6–1, a reaction time of 4 h and a temperature of 110°C, the catalyst yielded 93.5% conversion in the esterification of oleic acid with ethanol.
Based on the above discussion, there are two main methods for supporting ILs on silicon-based materials: Impregnation and grafting. Impregnation consists of physical adsorption of the IL, whereas in grafting the IL is immobilized on the surface through a chemical bond. Impregnation is simpler and less expensive than chemical grafting, but the resultant catalyst does not usually show good stability because the active sites are fallen off the surface of the support. In contrast, chemical grafting results in catalysts with higher stabilities, but it is more complicated and time-consuming, as well as consumes extra chemicals and reagents which increases the fabrication cost.
In chemical grafting, the IL is immobilized on the carrier surface through Si-O-Si covalent bonds. These bonds are formed by a chemical reaction between the silane groups and silanol groups on the carrier surface. There are two ways to incorporate silane groups in the structure of final catalyst. In the first method, which we call carrier modification, the as-synthesized carrier is functionalized with silane groups. Then, IL is connected to the silanol group through another group such as propyl or thiol. In the second method, called direct immobilization, the silane groups are first incorporated in IL structure, and then the IL is directly immobilized on the support.
Table 1 summarizes the optimized reaction conditions over ILs/silicon materials hybrid catalysts. As can be seen, all studies synthesized catalysts with large specific surface areas and obtained high biodiesel yields. Except for researches conducted by Karimi and Vafaeezadeh (2012) and Zhang et al. (2012), chemical grafting was used in all other studies for catalyst synthesis. Although Karimi and Vafaeezadeh (2012) did not report the biodiesel yield after several catalytic cycles, the results reported by Zhang et al. (2012) show that impregnation may also result in a catalyst with good stability. As impregnation is simpler and less-expensive than grafting, further studies on using this synthesis method with critical attention on recyclability are worth conducting in the future.
The high specific surface area of microporous silica-gel led to a comparable biodiesel yield with mesoporous silica supports. However, a higher amount of alcohol and longer reaction time was required which can be attributed to the smaller pore diameter of silica-gel and limited diffusion of the reactants as a result. These problems may be overcome by employing basic ILs which accompany lower alcohol consumption and shorter reaction time compared to acidic ILs. The effect of direct immobilization of IL on the recyclability of the final catalyst can also be investigated in future research.
Most studies in this category have been focused on SBA-15. By comparing the results, one could conclude that the modification of carrier with thiol groups (Wang et al., 2018) has the same effect on reaction yield and catalyst stability as the impregnation method (Zhang et al., 2012). On the other hand, by supporting basic ILs on SBA-15 using direct immobilization, Xie et al. (2015a,b) synthesized heterogeneous catalysts with higher stabilities. When the carrier is modified through thiol-ene reaction, the silane group on the surface is first attached to one atom of S through a propyl group. Then, this atom is connected to the cation of IL via another propyl group. In direct immobilization, on the other hand, the IL is directly attached to the silane group through a propyl group.
Among all the research on IL/silicon-based supports, Fan et al. (2018) obtained the best results. They reported almost 99% efficiency in transesterification of soybean oil with relatively low catalyst concentration and more importantly, the reaction yield remained over 95% after 5 catalytic cycles. Moreover, when the water and oleic acid contents of the feedstock were 5 wt.% and 9 wt.%, respectively, the yield of biodiesel was higher than 98%. These results indicate that mesoporous organosilica materials have great potential in (trans)esterification. Despite that Fan et al. (2018) first modified the organosilica by silane groups, they used a step-by-step procedure to immobilize the IL on the carrier so that the cation of IL was finally attached to the organosilica support by propyl-Si-O-Si bonds, the same method employed by Xie et al. (2015a,b).
Finally, in all three studies on magnetic mesoporous silica supports, high yields were reported. However, the catalysts fabricated by Zhen et al. (2012) and Zhang et al. (2013) did not show good recyclability due to the leaching of active sites, which was resulted from the breaking of Si-O bonds under harsh reaction conditions. In both studies, the support was first functionalized with thiol groups and then IL was attached to the support via free radical addition reaction between allyl groups of the IL and thiol groups on the surface of the carrier. On the contrary, Wan et al. (2015a) first functionalized the IL with silane groups, and then directly immobilized it on the magnetic silica support. The reaction yield remained over 87% after the catalyst was reused for 6 times.
In summary, direct immobilization of IL on the surface of mesoporous silica carrier through silane groups seems to reduce the leaching of IL and improve the catalyst recyclability compared to the case where thiol groups are used.
Organic polymers containing nanopores possess controlled wettability, flexible chemical tenability, and remarkable chemical stability in addition to the high surface area. Generally, these materials can be easily fabricated through hard-templating, soft-templating and template-free methods, and are functionalized by several strategies such as post-modification, co-polymerization of skeleton molecules with functional groups and self-polymerization of functional organic groups (Sun et al., 2015).
The functionalization of nanoporous polymers with ILs leads to the fabrication of so-called polymeric or polymerized ionic liquids or poly(ionic liquids)s (PILs). These polyelectrolytes consist of a polymeric backbone and an IL species in each monomer repeating units. PILs have been widely used as catalyst, catalyst support and pre-catalyst as they are mechanically stable and their catalytic influence can be controlled by the possibility of choosing a variety of cations and anions and tuning the macromolecular structure (Qian et al., 2017). There are two principal strategies to fabricate PILs, i.e. direct polymerization of IL monomers and post-modification of an already existing polymer. The structure, merits, and shortcomings of a PIL strongly depend on the synthesis technique and polymerization methodology (Yuan and Antonietti, 2011).
In the post-modification route, an existing precursor polymer is functionalized via chemical reactions. PILs obtained from this strategy possess the same number of monomeric units, architecture, and monomer composition as the primary polymer chains. Consequently, the desirable architecture and composition of PILs can be achieved by choosing a precursor with suitable structure, mass, and architecture (Yuan and Antonietti, 2011). The modification of nanoporous polymers for the preparation of PIL catalysts and their use in the biodiesel production process was first reported by Liu F. et al. (2012). They synthesized several mesoporous superhydrophobic polydivinylbenzenes by solvothermal co-polymerization of divinylbenzene with a series of vinyl-based monomers, followed by quaternary ammonization with CH3I and ion exchange with HSO3CF3. Compared to the same homogeneous ILs and ILs supported on SBA-15 and Amberlyst 15, the obtained IL-functionalized mesoporous polymers gave higher palmitate yield in transesterification of tripalmitin. This was attributed to the excellent adsorption of the reactants on the catalyst as a result of its significant wettability for the reactants. The catalyst was also well-recyclable because of the stable polymer structure. However, very high alcohol to tripalmitin molar ratio (90–1) and long reaction time (16 h) were required to achieve high yields.
Templating is an effective method for the synthesis of nanoporous polymers. Noshadi et al. (2014) successfully synthesized acidic PILs with an average pore size of 11.1 nm using resol and Pluronic F127, as precursor and template, respectively. The crosslinker hexamethylenetetramine (HMTA) was used to link the precursor to the template. After template removal through calcination, an ordered mesoporous resin (OMR-[HMTA]) was obtained using the crosslinker HMTA. Following modification of the mesoporous polymer through quaternary ammonization with 1,4-butanesultone and anion exchange with H2SO4, the polymeric ionic liquid OMR-[C4HMTA][SO4H] was obtained. Due to high acidity, high specific surface area, and a stable hydrophobic polymeric structure, this catalyst outperformed HCl and Amberlyst 15 in the esterification of a brown grease feed with high FFA content. The conversion of FFA to biodiesel reached 99.5% only with a methanol-to-grease molar ratio of 9:1 at 65°C within 1.5 h. The yield of esterification decreased from 99.5 to 96% after 5 times of the PIL catalyst recovery indicating its reasonable chemical stability. The brown grease with a FFA content of 90% was transesterified with a yield of 75% in the presence of this catalyst. The corresponding value for Amberlyst 15 was 65%. However, due to the need for high volumes of alcohol (the methanol-to-feed molar ratio of 40–1) and moderate yield, this catalyst is not suitable for the transesterification reaction presumably due to its small pores that do not provide adequate space for the diffusion of larger triglyceride molecules.
Adding sulfonic groups to a polymer functionalized with an acidic ionic liquid (IL) may significantly improve both acidity and catalytic activity. After synthesizing a mesoporous polymer through copolymerization of divinylbenzene with 1-vinylimidazole, Pan et al. (2016) functionalized the resulting polymer with an IL through quaternary ammonization with 1, 3-propanesultone and anion exchange with H2SO4. The resulting acidic PIL was sulfonated with chlorosulfonic acid to add a sulfonic acid group to the monomer. Within 4 h, the conversion of oleic acid reached 98% in the presence of a 5 wt.% catalyst with a methanol-to-oil molar ratio of 30–1 at 100°C. Moreover, simultaneous esterification and transesterification of Jatropha oil with a high acid value of 15 mg KOH/g were carried out in the presence of the catalyst. A conversion of 94% was obtained within 8 h at 160°C with a methanol-to-oil ratio of 50:1 (mol/mol) and 6 wt.% catalyst. Despite the lower acidity of this catalyst than Amberlyst 15, its BET surface area was 5 times higher leading to a significant increase in the conversion.
One limitation of some PILs used in biodiesel production is their nano-scale particles causing loss of catalyst during separation from reaction products. Increasing the particle size to micro-scale facilitates catalyst separation and reduces relevant costs. Using the procedure shown in Figure 4, Feng et al. (2017) synthesized acidic PIL microspheres with an approximate diameter of 500 μm through copolymerization of 1-vinylimidazole, divinylbenzene and styrene and then quaternary ammonization with 1,3-propanesultone and anion exchange with H2SO4. The resulting catalyst, P(VB-VS)HSO4, had an oleophilic mesoporous polymer network with a high specific surface area and abundant mesopores leading to the increased contact area of reactants with active sites and thereby enhanced mass transfer. Due to the good chemical and thermal stability of the catalyst, the conversion of transesterification of soapberry oil remained over 90% after 6 times of catalyst recovery. Interestingly, catalyst loss was nearly zero during recovery and reuse due to micron-sized catalyst particles. Moreover, the resulting PIL led to a high biodiesel production yield from various feeds with high acid values.
Figure 4. Fabrication procedure of P(VB-VS)HSO4 [Redrawn with modification from Feng et al. (2017)].
It has been reported that post-modification of the mesoporous melamine-formaldehyde as a class of covalent organic polymers (COPs) with ILs creates an ideal PIL for catalyzing esterification reactions. The repeating units in the mesoporous melamine-formaldehyde are connected through irreversible covalent bonds leading to high chemical and hydrothermal stability. Since this polymer is synthesized from low-cost available monomers, i.e., melamine and para-formaldehyde, its production cost is lower than divinylbenzene-based polymers. As another advantage, many aminal groups and triazine rings in the polymer network provide abundant sites for functionalization. Pan et al. (2019) synthesized this polymer by templating using Pluronic F127 and sodium dodecyl sulfate as templates. The polymer was then modified with 1,3- propanesultone and anion exchange with H3PW12O40 to obtain the MMFP-IL PIL. High acidity and surface area of this catalyst led to a yield of 95% in converting oleic acid to biodiesel. Strong covalent bonds between the polymer and IL as well as the stable structure of the catalyst led to its acceptable activity after 4 times of reuse.
There has been recently a great interest in the use of cheap monomers to reduce PIL production costs. For instance, Pei et al. (2019) used petroleum pitch as a monomer to produce a mesoporous polymer through hypercrosslinking. The resulting polymer was first functionalized by allyl chloride and then imidazole and ultimately modified through reaction with 1,3-propanesultone and anion exchange with 1,3-p-toluenesulfonic acid to achieve the PIL [HCPpitch–Im–Pros][Tos]. Oleic acid was esterified on this catalyst up to a yield of 93%, and the catalyst was used 5 times with an insignificant catalyst loss. Since the chlorine ions are replaced with imidazole groups in this method, the number of functional groups in the polymer increases with further chlorine grafting on the polymer leading to an increase in catalytic activity.
Direct copolymerization of divinylbenzene with IL monomers is a less expensive process for the synthesis of PILs which eliminates the use of expensive coupling reagents. Polydivinylbenzene can enhance the mass transfer rate and prevent the acid sites from falling off the surface owing to its high hydrophobic BET surface area. Liang (Liang, 2013a) fabricated an acidic PIL using the procedure illustrated in Figure 5. The Bronsted acidic IL monomer [SO3H(CH2)3 VIm]HSO4 was synthesized via the reaction between 4-vinylpyridine and 1,3-propane sulfonate, followed by acid treatment with H2SO4. The obtained monomer then was successfully copolymerized with divinylbenzene and was used in transesterification of waste cooking oil with methanol. After 12 h, a 99.1% biodiesel yield was achieved with 50 mg catalyst amount at 70°C and methanol to oil molar ratio of 15–1. After being used for 6 times, the reaction yield was still 99%, demonstrating its high stability.
Figure 5. The synthesis procedure of mesoporous acidic PIL [Redrawn with modification from Liang (2013a)].
Some PILs have a small specific surface area leading to inadequate access of reactants to catalytic sites. Hard templating of nanoparticles can be used to overcome this problem, which is a facile and effective method for producing porous materials with a suitable pore structure. However, a suitable template is required for this purpose to generate a uniform pore structure and its facile removal from the polymer at the end of the process. Wu et al. (2016a) used Fe3O4 nanoparticles as a template for the synthesis of a PIL catalyst. To this end, Fe3O4 nanoparticles were first modified with 3-methacryloxypropyltrimethoxy-silane and then polymerized with the IL monomer 1-vinyl-3-(3-sulfopropyl)imidazolium hydrogen sulfate. An acidic macroporous catalyst was obtained by removing Fe3O4 nanoparticles by water/ethanol/hydrochloric acid solution and ultrasonication. Conversion of over 90% was obtained with this PIL to convert oleic acid to methyl oleate. The resulting PIL contained a large number of sulfonic acid and hydrogen sulfate groups. The PIL was reused up to 6 times without leaching its acidic sites.
In another study, Wu et al. (2016c) synthesized a magnetic PIL functionalized with phosphotungstic acid using Fe3O4 nanoparticles and copolymerization of the acidic IL monomer 1-vinyl-3-(3-sulfopropyl) imidazolium hydrogen sulfate with the crosslinker divinylbenzene. To functionalize Fe3O4 nanoparticles with vinyl groups, 3-(trimethoxysilyl)propyl methacrylate was used. The highly acidic catalyst used for esterification of oleic acid at a methanol-to-oil molar ratio of 12:1 at 90°C led to a conversion of 93.4% after 5 h in the presence of 13 wt.% catalyst. Due to good magnetic properties, the catalyst was easily separated from products and reused for 6 times.
In addition to hydrophobicity, tunable wetting of catalyst for both reactants and products is of great importance, as it improves the performance and recoverability of the catalyst. A basic PIL was synthesized through radical polymerization of 1-octyl-3-vinylimidazolium bromide with the crosslinker 1,4-butanediyl-3-bis-1-vinylimidazole and then anion exchange with KOH (Jiang et al., 2017). The resulting polymer contained mesopores with an average diameter of 35 nm and basicity of 3.67 mmol/g. The rough surface of this polymer with a unique porous structure, an organic hydrophobic framework, and long chains of IL monomers led to a superhydrophobic polymer. Moreover, the high affinity of this catalyst for methanol and soybean oil led to the high yield of transesterification reaction. On the other hand, the basic PIL was incompatible with glycerol leading to desorption of the byproduct, glycerol, from the catalyst surface. The catalyst resisted against a water content of up to 1.5 wt.% (relative to oil) and the triglyceride conversion reduced from 96.3 to 85% after 5 times of catalyst reuse. The conversion was lower than the heterogeneous CaO catalyst due to the lower basicity of the PIL. However, due to the lack of metal ions in the PIL structure, it does not cause environmental pollution unlike CaO.
The free radical polymerization between an IL monomer and divinylbenzene in the presence of the radical initiator azobisisobutyronitrile (AIBN) is a common method for the synthesis of PILs. Bian et al. (2019) synthesized an acidic PIL by this method and used it for esterification of oleic acid. The IL monomer was synthesized through the reaction of methyldiallylamine with 1,3-propanesulton and then ion exchange with trifluoromethanesulfonic acid. Despite the lower acidity of the resulting catalyst than Amberlyst 15, it led to a higher yield for methyl oleate production due to the higher surface area of the PIL (301.1 m2/g) than Amberlyst 15 (47.2 m2/g). The resulting PIL also had a reasonable recovery and reuse capacity.
The results of research on biodiesel production over PILs are summarized in Table 2. As can be seen, except for the conversion of brown grease (trans)esterification was successfully catalyzed by all the synthesized PILs with yields higher than 90%, and the minimum yield after several catalytic cycles was 74%.
The post-modification method was used in the first six studies (Liu F. et al., 2012; Noshadi et al., 2014; Pan et al., 2016, 2019; Feng et al., 2017; Pei et al., 2019), in which the IL is supported on an as-synthesized polymer. This method is based on three consecutive steps including polymer synthesis, modification through quaternary ammonization, and ion exchange. Among the aforementioned studies, Liu F. et al. (2012) reported the highest reaction yield and best recyclability, which can be ascribed to the use of super acid SO3CF3 anion. However, this result was accompanied by the highest alcohol consumption and the longest reaction time in this category, which is probably due to the lack of catalytic sites on the imidazolium cation. This speculation is confirmed by investigating the results of the next studies, in which more moderate reaction conditions were obtained by attaching a sulfonic acid group to the cations of PILs. When more acid sites are available for reactants, the reaction rate is increased and less alcohol is consumed.
In the next five studies (Liang, 2013a; Wu et al., 2016a,b,c; Jiang et al., 2017; Bian et al., 2019), direct polymerization was employed in which the IL monomer is first prepared and then the resultant PIL is obtained from copolymerization of IL monomer and divinylbenzene. Among this group, Liang (2013a) reported the best results in the transesterification of waste cooking oil. A yield of higher than 99% with only 1 wt.% catalyst dosage was obtained and the yield remained at 99% after 6 catalytic cycles. This significant activity and reusability of the catalyst can be due to the very high specific surface area (>500 m2/g) and the simultaneous presence of SO3H and HSO4 groups in the cation and anion, respectively.
The only basic PIL in this group was fabricated by Jiang et al. (2017) and showed high yield and good stability in the transesterification of soybean oil. However, given to its basic nature, lower alcohol consumption and shorter reaction time are expected compared to the acidic PILs, which is not observed. This may be due to the presence of octyl groups on the imidazolium cation of PIL and its complex cross-linker. Although incorporating these groups enhances hydrophobicity and expels glycerol from the surface of the catalyst, it limits the diffusion of reactants toward the active sites.
Among all studies focused on PILs, Bian et al. (2019) synthesized the only PIL with a non-cyclic diallylamine cation and obtained good results both in terms of yield and mild reaction conditions in the esterification of oleic acid.
From what mentioned earlier, it can be concluded that both post-modification and direct polymerization could result in highly active PIL catalysts for (trans)esterification. Nevertheless, given the low price of divinylbenzene, using a template-free method could reduce the fabrication cost of final polymer compared to the templating technique. Furthermore, direct polymerization is less complicated than the post-modification method, so the synthesis cost of PILs could be reduced by direct polymerization of IL monomer with the cheap divinylbenzene as co-polymer.
In addition to the direct use of PILs as esterification and transesterification catalysts, the immobilization of these materials on nanoporous supports has also been studied. For example, palygorskite is a mineral with a nanofiber-like structure containing aluminum and hydrated magnesium. These nanofibers have a diameter of 100–500 nm with a length of 1–2 μm. High specific surface area, high thermal and mechanical stability, and abundant silanol units on the surface have turned this substance good support. Moreover, this substance is abundant in nature and sufficient hydroxyl groups can be created by acid activation to increase its grafting density. Zhang W. et al. (2017) synthesized a mesoporous acidic PIL with an average pore diameter of 19 nm. The vinyl groups were grafted on the surface of palygorskite through reaction with γ-methacryloxypropyl trimethoxy silane and then grafting polymerization of the acidic IL monomers 1-butysulfonate-3-vinylimidazole hydrogensulfate in the presence of AIBN was performed. In the synthesis of this PIL, the weight ratio of the IL to palygorskite, temperature, reaction time and AIBN level affect the amount of ionic liquid immobilized on the support and thus should be optimized. A yield of 69% was reported for methyl ester in the presence of the immobilized PIL, which reduced to 22% after 6 times of catalyst recovery. This significant reduction in reaction yield was attributed to the dissolution of IL in methanol and its reduced loading on the support, adhesion of esters on the support and reduced specific surface area and active sites and catalyst loss in the recovery process.
Later, the palygorskite-supported PIL was used for the preparation of a hybrid organic-inorganic pervaporation catalytic membrane. In addition to the catalytic effect, these membranes increase the efficiency of equilibrium reactions by removing one of the products from the reaction medium. The membrane was prepared by mixing poly(vinyl alcohol) with the PIL supported on palygorskite and succinic acid was used to link Poly(vinyl alcohol) through crosslink reaction. Adding a solid catalyst to poly(vinyl alcohol) improved thermal and mechanical stability and hydrophobicity of the PIL. Although the membrane successfully removed water produced from esterification of oleic acid, the maximum yield was about 5% due to very low PIL levels supported on palygorskite in the membrane structure and its very low effective contact area (24.6 cm2) (Li et al., 2020).
Another class of supports used for immobilizing PILs is nanoporous magnetic silica that facilitates PIL separation from the reaction mixture by an external magnetic field. Zhang H. et al. (2017) immobilized a basic PIL on the magnetic mesoporous support, Fe3O4@SiO2@SBA-15 (FnmS), by radical polymerization. Figure 6 shows the fabrication route to this catalyst. To prepare the support, Fe3O4 nanoparticles were first prepared by the solvothermal method and then coated with SiO2 by the sol-gel method to obtain Fe3O4@SiO2 microspheres. Thereafter, the external layer of the mesoporous silica was formed on the support by adding tetraethyl orthosilicate, P123, and HCl. The resulting magnetic mesoporous support was functionalized with sulfhydryl groups after reaction with (3-mercaptopropyl)trimethoxysilane. The IL monomer was produced from the reaction of 1-vinylimidazole with ethyl bromide and then participated in precipitation polymerization with a crosslinker and 2,2′-azobis(2-methylpropionitrile). Eventually, the hydroxide ions replaced bromide ions through ion exchange with tetramethylammonium hydroxide to obtain the basic Fnms-PIL catalyst. In addition to a high surface area, the density of basic sites was high and grafting of organic functional groups did not negatively affect the mesoporous structure. The yield of transesterification reaction of the non-edible vegetable oil in the presence of this catalyst under mild reaction conditions including a methanol-to-oil molar ratio of 9:1, a catalyst concentration of 4 wt.% and the reaction time of 5 h at 85°C was 92.8%, which remained about 90% after 5 times of catalyst reuse.
Figure 6. The synthesis process of Fe3O4@SiO2@SBA-15-BPIL [Redrawn from Zhang H. et al. (2017)].
As mentioned above, water as an esterification product negatively affects the catalytic activity and causes leaching of active sites. In another study, Zhang et al. (2018) synthesized several acidic FnmS-PIL catalysts and found an increase in the catalytic activity and resistance to water with increasing the length of alkyl groups attached to FnmS and anion acidity. An increase in the length of alkyl groups used for functionalizing the support improved its hydrophobicity. Among various catalysts containing the anions CF3, , Cl−, 1/3 PW12, the catalyst containing CF3 showed the highest yield due to the higher acidity of this anion. The catalyst showed a good performance in converting oleic acid and Euphorbia lathyris L with a high acid number. A yield of over 90% was obtained when the water content in the feed increased up to 6 wt.%.
Fe3O4 nanoparticles are coated by a silica layer primarily for two reasons. First, magnetic nanoparticles are aggregated and form large clusters due to the bipolar-bipolar magnetic property. This in turn causes the non-uniform distribution of the catalyst in the reaction mixture and thereby reduced catalytic activity. Second, the silica layer prevents corrosion of iron oxide nanoparticles under harsh conditions. Recently, an acidic catalyst has been synthesized through radical copolymerization of the IL monomer 1-vinyl-3-(3-sulfopropyl)imidazolium hydrogen sulfate and Fe3O4/SiO2 nanocomposites functionalized with vinyl groups (Xie and Wang, 2020). The vinyl groups were grafted on the Fe3O4/SiO2 support through reaction with 1-vinyltriethoxysilane. The resulting hybrid organic-inorganic catalyst successfully converted 93.3% of oil to biodiesel through esterification and transesterification of oil with high FFA content, whereas FFA was completely converted into biodiesel. The conversion of the oil to biodiesel was lower in the presence of the unsupported homopolymer. Given the same number of acidic sits, this was related to the lower surface area in this case. Moreover, due to strong covalent bonds between the grafted IL and the support, the active acidic sites were preserved on the support surface so that conversion of over 80% was obtained after 5 times of catalyst reuse.
To summarize the above discussion, the supported PILs are synthesized by copolymerization of the IL monomer and carrier, and Si-O-Si bonds are used to attach the cation of IL to the surface of mesoporous silica. To connect the cations to Si atoms, various chemical groups such as vinyl, propyl, and thiol have been employed.
Table 3 compiles the results of previous research on biodiesel production over mesoporous silica-supported PILs. Overall, excluding the results found by Zhang W. et al. (2017) and Li et al. (2020), the other studies reported satisfactory findings.
In both studies conducted by Zhang W. et al. (2017) and Li et al. (2020), the catalyst was fabricated by copolymerization reaction between the IL monomer 1-butysulfonate-3-vinylimidazole hydrogensulfate and palygorskite as the support. In each unit of the resultant catalyst, the imidazolium cation is attached to one Si atom through a long chain containing propyl and vinyl groups. In the presence of PAL-PILs catalyst, the reaction yield fell dramatically after 6 cycles, which was due to the leaching of the PILs and active sites and significant reduction in specific surface area (from 43 to 11 m2/g) as a result of the adhesion of esters on the palygorskite. Therefore, the stability of active sites should be improved, for which the IL can be attached to palygorskite through silane or thiol groups.
On the other hand, the very low yield was obtained by Li et al. (2020) when CPVA/PAL-PILs were employed. This resulted from the limited number of active sites and negligible specific surface area of the catalyst. In PAL-PILs, the amount of PIL loaded on the support was 41%. But the main issue is associated with the loading amount of PAL-PILs on the CPVA which was very low (around 2%), which dramatically reduces the number of accessible active sites. Moreover, polyvinyl alcohol may cover these active sites and limit the diffusion of the reactants. Therefore, increasing the specific surface area and PIL loading will be the main challenge in future studies.
The sole basic PIL in this group was synthesized and supported on magnetic mesoporous silica by Zhang H. et al. (2017). They used thiol groups to attach the imidazolium cation of PIL to the carrier which led to a high biodiesel yield and good stability. The same method was employed for immobilizing an imidazolium-based acidic PIL on magnetic mesoporous silica and satisfactory results were obtained (Zhang et al., 2018). These results demonstrate the good performance of the thiol-ene reaction for supporting PILs on silica materials.
Finally, vinyl groups were employed by Xie and Wang (2020) for the immobilization of an acidic PIL on a magnetic mesoporous silica carrier. The strong covalent bonds were responsible for the reasonable recyclability and high biodiesel yield in the transesterification of soybean oil. Therefore, incorporation of vinyl groups can result in high stability catalysts.
Metal-organic frameworks (MOFs) are crystalline nanoporous materials that are obtained by combining inorganic nodes (including metal ions or clusters of metal ions) and organic ligands. MOFs have numerous advantages such as high thermal stability, ordered structures, very low density, a large internal surface area up to over 6,000 m2/g, and facile preparation through self-assembly (Gangu et al., 2016). Depending on the type of metal ions and organic linkers and direction of linkages between ions, many MOFs with a variety of framework geometries can be designed. These structures contain voids or pores that their size, shape, and functionalization can be well-controlled unlike zeolites. Therefore, these pores can be used to confine the desired molecules (Safaei et al., 2019).
MOFs have recently played a key role in heterogenizing ILs to be used in various areas including gas adsorption, catalysis, and fabrication of nanoporous carbon. In addition to a large number of nanopores in their crystalline structure, their properties such as pore size, surface area, framework topology, and polarity of the inner surface are tunable (Fujie and Kitagawa, 2016). MOFs are very similar to ILs in this regard. As properties of ILs are determined by cations and anions used in their structures, properties of MOFs can be designed by using various metal ions and organic ligands.
In general, there are two methods for incorporation of ILs in the structure of MOFs: ionothermal synthesis and post-synthetic modification. As a solvothermal method, an IL is used as a solvent in the ionothermal method, and IL cations are attached to negatively charged MOF frameworks. Given the strong interaction of cations and the MOF framework, the useful properties are limited in comparison with the bulk IL. Furthermore, functional groups used in this method should be compatible with the host MOF, thus a limited number of ILs and MOFs can be used. In contrast, ILs are used in the as-synthesized MOFs in the post-synthesis method. As a result, the need for compatibility of ILs and MOFs is eliminated to a large extent and a wide range of both materials can be used (Cota and Martinez, 2017). Accordingly, the post-synthesis method has been used in all studies on the application of hybrid IL-MOF catalysts for biodiesel production.
For the first time, Wan et al. (2015b) confined ILs in the polyoxometalate-based MIL-100(Fe) (POM-MIL-100) to be used as a heterogeneous catalyst for biodiesel production. The MOF was synthesized by the hydrothermal method by combining FeCl3.6H2O, phosphotunhstic acid (H3PW12O40), and benzene-1,3,5-tricarboxylic acid (H3BTC) leading to confining of H3PW12O40 in the MIL-100(Fe) framework. The IL 1-(propyl-3-sulfonate) imidazolium hydrogen sulfate [SO3H-(CH2)3-HIM][HSO4] was converted into the IL [SO3H-(CH2)3-HIM]3PW12O40 through anion exchange with H3PW12O40 and encapsulated in the MOF framework. To this end, POM-MIL-100 was added to the IL-methanol solution and the resulting mixture was vigorously stirred for 10 min and then treated with ultrasound waves for 30 min. The mixture was stirred for another 12 h at room temperature. Eventually, the resulting solid was centrifuged and washed with diethyl ether and dried in a vacuum oven at 60°C. The catalyst (POM-IL@MIL-100) led to a conversion of 94.6% in converting oleic acid with ethanol under optimal conditions. It was more active than MIL-100 and POM-MIL-100, which can be related to the high acidity (1.74 mmol/g) of the catalyst caused by the synergistic effect of Brønsted acid sites of the IL and Lewis acid sites provided by FeIII ions. The catalyst was recovered 6 times and reused without any significant reduction in the conversion.
In 2016, Han et al. (2016) developed a novel method for confining the dicatonic IL [SO3H-(CH2)3-IM]2C4[HSO4]2 in the MIL-100(Fe) framework. In this method known as the impregnation-reaction-encapsulation process, small molecules of the IL diffused into nanopores of the MOF and began to grow in the nanopores up to a point that was no longer able to escape from the MOF cages due to their large size. To this end, [SO3H-(CH2)3-HIM][HSO4] was added to a solution of methanol and MIL-100(Fe). After stirring for 30 min, 1,4-dibromobutane was dropwise added to the mixture at 0°C and stirred for 12 h at 25°C and for another 12 h at 60°C. The resulting solid was centrifuged and after washing with methanol, was performed with methanol at 40°C for another 12 h. Eventually, the product was dried under vacuum at 80°C for 12 h. 15 wt.% of the catalyst (MIL-100(Fe)@DAILs) converted 93.5% of oleic acid into methyl oleate. After 5 times of reuse, the conversion reduced to 86%. The results of FTIR spectra revealed that the size of dicationic IL molecules (length: 1.93 nm, width: 0.9) caused their confinement in the mesocages of the MOF (diameter: 2.9) so that they were not able to escape through 0.86 nm apertures. In contrast, the catalyst obtained from direct impregnation of the IL [SO3H-(CH2)3-HIM][HSO4] (length: 1.05 nm, width: 0.6 nm) led to a conversion of 22.3% after 5 times of reuse indicating leaching of large amounts of the IL from the MOF mesocages.
In recent years, magnetic MOFs have been used as the support of ILs in biodiesel production. Wu et al. (2016b) prepared an acidic heterogeneous catalyst for ethyl oleate production through confining the Brønsted acidic IL 1,4-butanediyl-3,3'-bis-(3-sulfopropyl) imidazolium dihydrogensulfate in the magnetic MOF Fe3O4@NH2-MIL-88B(Fe). The magnetic support functionalized with amino groups was obtained through thermal treatment of 2-aminoterephthalic acid, FeCl3.6H2O, N,N-dimethyl formamide, and Fe3O4 nanoparticles. The support was then dispersed with the IL in ethanol and mixed for 24 h at 110°C to obtain the catalyst DAIL-Fe3O4@NH2-MIL-88B(Fe) through the interaction of amino and sulfonic acid groups. A conversion of 93.2% was obtained for the reaction of oleic acid with ethanol, which was higher than the unsupported ionic liquid catalyst. A lower conversion was obtained when the monocationic IL [SO3H-(CH2)3-IM][HSO4] was immobilized on the support due to the lower number of SO3H and HSO4 groups and thereby a lower acidity.
Besides direct use of ILs, tandem post-synthetic modification can be also used for the incorporation of ILs in the MOF structure. One approach is to place functional groups on the IL and then immobilization of the functionalized IL on MOFs. Using this method, Han et al. (2018) first functionalized the ILs 2-mercaptobenzimidazole (MBI) with electron-enriched thiol groups. The resulting IL was then immobilized on the surface of MIL-101(Cr) through S-Cr coordination bonds. To synthesize the catalyst, MBI was first dissolved in ethanol and then MIL-101(Cr) was added. After 24 h mixing at room temperature, centrifugation, washing the solid with ethanol, and drying under vacuum at 60°C, MIL-101(Cr)@MBI was obtained. Thereafter, MIL-101(Cr)@SO3-(CH2)3-HMBI was obtained by dispersing MIL-101(Cr)@MBI in ethyl acetate and reacting with 1,3-propanesulfonate for 12 h at 60°C. Eventually, the product was dissolved in ethanol and reacted with H2SO4 for 24 h at 60°C to obtain MIL-101(Cr)@[SO3H-(CH2)3-HMBI][HSO4]. Figure 7 shows the procedure for the synthesis of this catalyst. The results showed that immobilization of the acidic IL on MIL-101(Cr) through S-Cr coordination bonds not only increased the oleic acid conversion but also improved catalyst reusability as compared with the neat MOF.
Figure 7. Synthesis process for MIL-101(Cr)@[SO3H-(CH2)3-HMBI][HSO4] [Redrawn with modification from Han et al. (2018)].
In a recent study by Xie and Wan (2019), the IL [SO3H-(CH2)3-HIM][HSO4] was encapsulated in the POM-UiO-66-2COOH framework. The MOF was synthesized through the in-situ reaction of ZrCl4, H3PW12O40, and 1,2,4,5-benzene-tetracarboxylic acid. Subsequent anion exchange between the acidic IL and the heteropoly anion led to the synthesis of ILs/POM/UiO-66-2COOH. A conversion of 95.8% was obtained for transesterification of soybean oil with methanol using this catalyst under optimal conditions. Strong interactions between sulfonic acid groups and heteropoly tungstate molecules effectively prevented the loss of active species from the MOF support and led to reasonable stability of the catalyst during multiple recoveries and reuse cycles. The catalyst showed higher activity in FFA esterification than the transesterification of soybean oil.
Ye et al. (2019) proposed a new method for confining the acidic IL 1,3-biscarboxymethyl-imidazolium hydrosulfate in the UiO-66 framework. In the so-called approximate ligand substitution method, parts of ligands were eliminated through MOF etching by propionic acid to produce the hierarchical porous UiO-66 with a large number of defects and Lewis acid sites. Then, the IL precursor was supported on H-UiO-66 through bidentate coordination bonds between a COO−1 group and two unsaturated Zr ions. The final catalyst was obtained by the addition reaction of the modified MOF with H2SO4. This catalyst showed higher activity in the esterification of oleic acid (93.8%) than the bulk IL (89.2%), UiO-66 (25.6%), and H-UiO-66 (78.6%). UiO-66 also showed a catalytic activity due to defects and unsaturated Zr atoms in its natural structure leading to the opening of Lewis acid sites. However, these Lewis acids are not well accessible due to the lower diameter of pore aperture than the oleic acid molecule. By opening this MOF structure and with an increase in the size of pores, the catalytic activity of H-UiO-66 becomes much greater than MOF. By immobilizing the IL in the MOF structure, Brønsted acid sites are added to the Lewis acid sites leading to an increase in the acidity and thereby reaction yield.
Using the tandem post-synthetic modification method, ILs with ions larger than the aperture diameter of the pores can be incorporated into MOF pores (Fujie and Kitagawa, 2016). In another approach of this method, MOFs can be functionalized through unsaturated metal centers (UMCs). In this method, active species are grafted through coordination covalent bonds with UMCs. Chen et al. (2016) used this method for the functionalization of HKUST-1 through coordination bonding of Cu2+ metal centers with thiol functional groups (S-Cu coordination bonds). For the synthesis of thiol-functionalized HKUST-1, ethanedithiol was dissolved in anhydrous toluene and HKUST-1 was gradually added to the resulting solution. The mixture was stirred for 24 h at room temperature and the resulting suspension was centrifuged. The resulting solid was washed with ethanol and then dried under vacuum at room temperature. Thereafter, this substance was dispersed in the IL [HVIm-(CH2)3SO3H]HSO4 in anhydrous ethanol. After rising the temperature to 80°C, AIBN was added under nitrogen and the reaction continued for 30 h. The product was separated by filtration and then was washed several times with ethanol to remove excess IL. Finally, the catalyst IL@HKUST-1 was obtained by drying under vacuum at 60°C for 12 h. The catalyst was synthesized through covalent bonding of the vinyl groups of the IL with thiol groups in the HKUST-1 structure to achieve a conversion of 92.1% in the esterification of oleic acid to methanol. The conversion reduced to 86.3% after 5 catalytic cycles due to the decomposition of the HKUST-1 structure and thereby loss of active IL.
In another study, Xie and Wan (2018) immobilized amino-functionalized ILs on the magnetic HKUST-1. The layer-by-layer assembly was used to prepare Fe3O4@HKUST-1 composites. The Fe3O4 nanoparticles were first modified with mercaptoacetic acid and then added to the Cu(CH3COO)2.H2O solution in ethanol. The resulting mixture was stirred for 30 min at 70°C and the obtained solid was dispersed in H3BTC solution in ethanol. After 1 h stirring of the mixture at 70°C and separation of the solid with a magnet and washing with ethanol, the production cycle was terminated. After 20 same cycles, Fe3O4@HKUST-1 with a core-shell structure was obtained. The composite was then added to the amino-functionalized IL solution in ethanol and the mixture was stirred for 24 h at 60°C. Eventually, the basic magnetic catalyst Fe3O4@HKUST-1-ABILs was obtained after magnetic separation, washing with ethanol, and drying. In this process, the IL was added to the MOF structure through N-Cu coordination bonds. The basic catalyst led to a conversion of 92.3% in transesterification of soybean oil at an ethanol-to-oil molar ratio of 30:1 and a catalyst dosage of 1.2% within 3 h. The catalyst was used 5 times without a significant reduction in the conversion.
Table 4 presents the results of previous studies on (trans)esterification of various feedstock over MOF-supported ILs. As can be seen, yields of higher than 90% were obtained in all studies.
Regarding the research on MIL supports, ILs were either encapsulated inside the MIL framework (Wan et al., 2015b; Han et al., 2016) or immobilized on the surface of the framework (Wu et al., 2016a,b; Han et al., 2018). The results suggest that encapsulation leads to catalysts with better recyclability (higher yield after multiple reuses) compared to surface immobilization. On the other hand, a higher amount of catalyst is required for high biodiesel yields when encapsulation is employed. This can be attributed to the fact that when IL is confined inside the framework, the leaching of active species is reduced but the active sites are less exposed to the reactants. On the contrary, in surface immobilization, the catalytic sites have more exposure to the reactants and lower catalyst dosage is required for a certain yield. However, as the IL is attached to the surface of the framework, its leaching will rise. Immobilization of IL on the surface of the MIL framework through SO3H-NH2 bonds (Wu et al., 2016a,b) has shown the same effect on recyclability as the direct attachment of the IL via S-Cr bonds (Han et al., 2018).
Ye et al. (2019), which employed the etching method for confining IL (CH2COOH)2IM][HSO4] inside UiO-66 framework, obtained a higher yield and better performance after several catalytic cycles by using a lower catalyst dosage. The MOF framework is partly broken by etching and more catalytic sites present in the IL are exposed to the reactants. This reduces the required catalyst concentration. On the other hand, leaching is decreased because the IL is attached to the framework through strong double Zr-O coordination bonds, which improves the reaction yield after multiple reuses.
In both works connected with HKUST-1, the ILs were immobilized on the surface of frameworks. Chen et al. (2016) first functionalized the framework by thiol groups and then the IL was attached to the framework through vinyl-thiol interactions. Therefore, the IL was immobilized on the framework via S-Cu coordination bonds. On the other hand, Xie and Wan (Xie and Wan, 2018) first functionalized the IL with amino groups and then attached it to the surface of the framework through N-Cu coordination bonds. Both resultant catalysts showed good activities, especially Fe3O4@HKUST-1-ABILs which with only 1.2 wt.% dosage led to over 90% biodiesel yield in transesterification of soybean oil.
Given to what mentioned earlier, all three methods have resulted in catalysts with high activity and reasonable reusability. However, the etching method combines the advantages of encapsulation and surface immobilization in fabricating a MOF-based IL catalyst. Thus, further research on the synthesis of new catalysts using this approach with an emphasis on the transesterification of non-edible and high FFA content oils is recommended.
Graphene-based nanomaterials as novel carbon materials with a nanosheet structure have a high surface area and good mechanical flexibility and thermal stability (Novoselov et al., 2004; Wang et al., 2012; Zhou et al., 2014; Hu et al., 2015, 2017). This causes reduced mass transfer resistance, good exposure of active sites, and improved recyclability of these nanomaterials and turns them ideal supports for active species such as ILs (Liu et al., 2015).
Despite these advantages, the synthesis of graphene-based nanomaterials with high surface area and abundant nanopores through a facile low-cost method is a great challenge. Moreover, these nanomaterials are chemically neutral making their functionalization by acidic functional groups difficult (Tang et al., 2015). Doping can be used to overcome this drawback and to modify other properties of nanoporous carbon materials (Albero and Garcia, 2015). Liu et al. (2015) produced a graphene-like nanoporous carbon by carbonizing melamine and glucose and subsequent doping with N2. Melamine and glucose were first mixed and crushed and then carbonized in a tube furnace under N2 gas flow at 800°C. After cooling down the furnace, N-doped graphene-like nanoporous carbon (GNC) was obtained. Thereafter, GNC was added to a mixture of toluene and 1,3-propanesultone and the resulting mixture was refluxed at 110°C for 24 h to complete the quaternary ammonization reaction. After cooling down to the room temperature, the mixture was stirred for 24 h at room temperature for anion exchange with toluene and HSO3CF3. Finally, the solid was separated by filtration and washed with CH2Cl2 and dried for 12 h at 60°C to obtain the heterogeneous acidic catalyst GNC-[C3N][SO3CF3]. This catalyst was used for the transesterification of tripalmitin with methanol. After 14 h, a conversion of 88.5% was obtained at 65°C, which was higher than that in the presence of Amberlyst 15, SBA-15-SO3H and H3PW12O40. The catalyst also showed good activity in the transesterification of sunflower oil, which was comparable with sulfuric acid. In addition to high acidity, 2D nanosheets provide the very good accessibility of reactants to active catalyst sites. Moreover, the good stability of the catalyst provides the possibility for 5 times of reuse in the transesterification reaction.
Despite good results, the use of nanoporous carbon materials functionalized with ILs as a catalyst for biodiesel synthesis is limited to this study. Thus, there is an obvious need for further research in this area.
In general, a suitable heterogeneous catalyst for (trans)esterification of oil should have a porous structure with abundant pores, high BET surface area, high basicity or acidity, high density of active species, and good dispersion of active sites. These properties increase the contact area of active sites with reactants leading to enhanced diffusion and mass transfer and thereby catalytic activity (Pirez et al., 2012; Wang et al., 2015). According to the above discussions, by combining unique properties of ILs and nanoporous materials, a catalyst with high activity and reasonable stability can be synthesized. Since these properties can be tailored, hydrophobicity, adsorption of reactants, and desorption of products from the catalyst surface can be well-designed.
In most of the studies, a BET surface area of higher than 100 m2/g was reported. However, according to the results of Wu et al. (2016a), Xie and Wang (2020), and Xie and Wan (2018, 2019) high yields can be obtained with smaller surface areas. Xie and Wan (2019) reported a 95.8% biodiesel yield in transesterification of soybean oil over AILs/HPW/UiO-66-2COOH with a specific surface area of 8.63 m2/g. On the other hand, the very low yield was obtained with a BET surface area of 0.00246 m2/g (Li et al., 2020). Therefore, a minimum BET surface area of 8 m2/g is recommended to achieve high conversions.
The catalyst acidity or basicity plays a key role in the catalyst activity so that acidity or basicity of the surface is directly related to the catalytic activity. Acidic or basic sites are required for the activation of carbonyl groups in the triglyceride molecules and initiation of the transesterification reaction (Xie and Wan, 2019). The acid content of acidic ILs/nanoporous materials varied from 1.13 to 4.3 mmol/g in the literature. The corresponding range for basicity was 2.03–3.67 mmol/g. Based on these results, one can conclude that the minimum acidity and basicity to achieve high biodiesel yields are around 1 and 2 mmol/g, respectively. Furthermore, the resistance of acidic catalysts against heat as well as water and FFA content of the feedstock prevent loss of active sites and improve recoverability and reusability of the catalyst.
The average pore diameter is another important factor that specifically affects reaction time. According to Granados et al. (2007) and Coenen (1986), a minimum pore diameter of 3.5 nm is preferable for producing biodiesel. However, high conversion and yield after multiple reuses was reported by Pei et al. (2019), using an average pore diameter of 2.56 nm. Therefore, a pore diameter of >2.5 nm should be considered to achieve high yields under mild reaction conditions.
The most serious obstacle for employing IL/nanoporous material hybrids is the high synthesis cost. To compare the cost of supported ILs with traditional catalysts, a cost estimation based on the synthesis routes proposed by previous studies was performed. The cost for all chemicals and reagents were obtained from Sigma-Aldrich. For NaOH and H2SO4, the prices presented by industrial manufacturers on Alibaba website were used. Table 5 gives information on the estimated fabrication cost of some supported ILs. As can be seen from the table, with a synthesis cost of $1/g, the acidic PIL Poly[SO3H(CH2)3VIm]HSO4 is the cheapest of all, following by IL/silica-gel and SBA-15-pr-ILOH. On the other hand, magnetic mesoporous ILs Fe3O4@HKUST-1-ABILs and Fe3O4@SiO2@SBA-15-BPIL are the most expensive which is obviously due to the more reagents required and longer and more complicated fabrication process of these catalysts.
It should be noted that the final cost of catalyst in the biodiesel production process is mostly affected by catalyst dosage and the number of reuse times. Lower catalyst dosages and more catalytic cycles reduce the final cost of catalyst. For example, among other catalysts, synthesis of 1 g of Fe3O4@HKUST-1-ABILs costs the most. However, taking into account the catalyst's low consumption and good reusability, it is the second least consumed catalyst and costs lower than some of the other supported-ILs in the production of 1,000 g biodiesel. Even by considering these two key factors, the costs of heterogeneous IL catalysts per 1,000 g biodiesel is far higher than the conventional NaOH and H2SO4. The acidic PIL Poly[SO3H(CH2)3VIm]HSO4 costs the least among all other heterogeneous ILs with only $1.67/1,000 g biodiesel. This is roughly 44 times higher than the cost for H2SO4 which is a common homogeneous acid catalyst for (trans)esterification of high FFA oils. On the other hand, Fe3O4@HKUST-1-ABILs is the most cost-effective basic supported IL, which costs ~5,040 times higher than NaOH.
One should consider that these estimations are based on the synthesis processes in laboratories. These figures would significantly reduce if heterogeneous ILs were produced at the industrial scale. DeSantis et al. (2017) estimated a total production cost of $53.75/kg ($0.054/g) for HKUST-1 at a plant capacity of 50,000 ton/yr, which is 108 times as high as the cost for NaOH. Also, the supported IL costs could be further reduced by increasing the loading amount of IL and improving the catalyst reusability. In the case of Poly[SO3H(CH2)3VIm]HSO4, as an example, decreasing the catalyst dosage by half and increasing the number of reuse times to 100 would result in an approximately same cost/1,000 g biodiesel as H2SO4. Another option is the use of cheaper anions and cations for IL synthesis. For instance, choline-based ILs are less expensive compared to many ILs because choline chloride as the main raw material is relatively cheap and commercially available for purchase (Andreani and Rocha, 2012).
According to the results in the above, almost in all studies, the reaction conversion, number of catalytic cycles, and yield after multiple recovery and reuse are reasonable indicating the accurate design of the catalyst by selecting suitable ILs and nanoporous materials. These results also indicate the high potential of IL-nanoporous material (NPM) hybrids as good alternatives for traditional catalysts for biodiesel production. Nonetheless, by focusing on some limitations in future studies a big step can be taken toward the use of these catalysts in the biodiesel industry.
Oleic acid has been used in most studies as the feedstock. It should be noted that esterification of oleic acid is only a model reaction for biodiesel production, and esterification and transesterification of diverse feedstocks such as algae (Nagarajan et al., 2013), municipal sewage sludge (Olkiewicz et al., 2016), and recycled grease trap waste (Tran et al., 2018) may provide more realistic results on the catalytic activity of IL-NPM hybrid materials. This becomes more significant considering that optimal conditions reported for transesterification are harsher than esterification.
The other challenge is harsh reaction conditions, especially high alcohol-to-oil molar ratios (up to 90:1) and long reaction times (up to 40 h). This is originated from the heterogeneous nature of the catalyst leading to the formation of three phases in the reaction medium and reduced mass transfer due to the immiscibility of oil and alcohol. To achieve mild reaction conditions, intensification processes such as microwave, microchannel, hydrodynamic cavitation, and ultrasonic reactors can be used (Tabatabaei et al., 2019). Using various technologies, these reactors increase the mass transfer rate while reducing the reaction time. For example, hydrodynamic cavitation significantly increases the contact area of cavitation-induced alcohol and oil phases and facilitates transesterification reaction with a high yield at room temperature with lower energy consumption and also alcohol-to-oil molar ratio (Gholami et al., 2018).
In most studies, optimal conditions for biodiesel production have been obtained through the one variable at a time method. Since this methodology does not consider the effect of interactions of effective variables on the reaction yield, the resulting conditions and yield do not necessarily reflect optimal conditions (Montgomery, 2013). On the other hand, the pore size of nanoporous materials, especially MOFs, can be tailored. However, this advantage has not been still used for the optimization of transesterification conditions. The synthesis of catalysts with different pore sizes and investigation of the effect of this parameter on the yield and reusability may help to develop an optimal stable catalyst. Moreover, optimal conditions and more realistic maximum yields can be obtained by combining this innovation with the design of experiments (DOE) and response surface methodology (RSM).
Further studies on the kinetics of transesterification in the presence of IL-NPM catalysts, especially in intensification reactors, may lead to design a suitable alternative process for conventional industrial units. Since ILs and nanoporous materials can be produced from a wide range of eco-friendly materials, the above process may play a key role in the sustainable development and reduction of adverse environmental effects.
The literature on biodiesel production using nanoporous materials functionalized with ILs as catalyst was reviewed. A variety of hybrids of ILs and nanoporous materials including nanoporous silica, polymers, MOFs, and carbon materials have been used for catalyzing the (trans) esterification reaction. All these materials have led to satisfactory results in biodiesel production. Moreover, these hybrids were considered attractive alternatives for conventional homogenous catalysts due to ease of separation, good stability, and activity after multiple catalytic cycles. Nonetheless, high synthesis cost, harsh reaction conditions, especially high alcohol-to-oil molar ratios, and long reaction times due to the heterogeneous nature of the catalyst were considered great challenges. The use of intensification processes may be a suitable solution to this problem. Moreover, tunable properties of IL-nanoporous material hybrids such as pore size and surface area reflected the need for further studies on the effect of catalyst structure on the yield and optimal reaction conditions and structure optimization. In particular, it was recommended to investigate the use of MOFs for facilitating catalyst separation from products given the ease of synthesis, flexible design, and the possibility to combine their properties with magnetic nanoparticles.
AG: conceptualization, methodology, data curation, writing, and original draft preparation. FP: conceptualization, methodology, supervision, writing-reviewing, and editing. AM: writing- reviewing and editing.
The authors declare that the research was conducted in the absence of any commercial or financial relationships that could be construed as a potential conflict of interest.
Abdurakhman, Y. B., Putra, Z. A., Bilad, M. R., Nordin, N. A. H. M., and Wirzal, M. D. H. (2018). Techno-economic analysis of biodiesel production process from waste cooking oil using catalytic membrane reactor and realistic feed composition. Chem. Eng. Res. Des. 134, 564–574. doi: 10.1016/j.cherd.2018.04.044
Abreu, F. R., Alves, M. B., Macedo, C. C. S., Zara, L. F., and Suarez, P. A. Z. (2005). New multi-phase catalytic systems based on tin compounds active for vegetable oil transesterificaton reaction. J. Mol. Catal. A Chem. 227, 263–267. doi: 10.1016/j.molcata.2004.11.001
Agarwal, D., Sinha, S., and Agarwal, A. K. (2006). Experimental investigation of control of NOx emissions in biodiesel –fueled compression ignition engine. Renew. Energy 31, 2356–2369. doi: 10.1016/j.renene.2005.12.003
Al Othman, Z. A. (2012). A review: fundamental aspects of silicate mesoporous materials. Materials 5, 2874–2902. doi: 10.3390/ma5122874
Albero, J., and Garcia, H. (2015). Doped graphenes in catalysis. J. Mol. Catal. A Chem. 408, 296–309. doi: 10.1016/j.molcata.2015.06.011
Alegria, A., de Arriba, A. L. F., Moran, J. R., and Cuellar, J. (2014). Biodiesel production using 4-dodecylbenzenesulfonic acid as catalyst. Appl. Catal. B Environ. 160–161, 743–756. doi: 10.1016/j.apcatb.2014.06.033
Amiril, S. A. S., Rahim, E. A., and Syahrullail, S. (2017). A review on ionic liquids as sustainable lubricants in manufacturing and engineering: recent research, performance, and applications. J. Clean. Prod. 168, 1571–1589. doi: 10.1016/j.jclepro.2017.03.197
Andreani, L., and Rocha, J. D. (2012). Use of ionic liquids in biodiesel production: a review. Brazil. J. Chem. Eng. 29, 1–13. doi: 10.1590/S0104-66322012000100001
Berthod, A., Ruiz-Angel, M. J., and Carda-Broch, S. (2018). Recent advances on ionic liquid uses in separation techniques. J. Chromatogr. A. 1559, 2–16. doi: 10.1016/j.chroma.2017.09.044
Bian, Y., Zhang, J., Liu, C., and Zhao, D. (2019). Synthesis of cross-linked poly acidic ionic liquids and its application in biodiesel production. Catal. Lett. 150, 969–978. doi: 10.1007/s10562-019-02988-0
Bourbigou, H. O., Magna, L., and Morvan, D. (2010). Ionic liquids and catalysis: recent progress from knowledge to applications. Appl. Catal. A General 373, 1–56. doi: 10.1016/j.apcata.2009.10.008
Canakci, M. (2007). The potential of restaurant waste lipids as biodiesel feedstocks. Bioresour. Technol. 98, 183–190. doi: 10.1016/j.biortech.2005.11.022
Cao, Y., Zhou, H., and Li, J. (2016). Preparation of a supported acidic ionic liquid on silica-gel and its application to the synthesis of biodiesel from waste cooking oil. Renew. Sustain. Energy Rev. 58, 871–875. doi: 10.1016/j.rser.2015.12.237
Chen, C., Wu, Z., Que, Y., Li, B., Guo, Q., Li, Z., et al. (2016). Immobilization of a thiol-functionalized ionic liquid onto HKUST-1 through thiol compounds as the chemical bridge. RSC Adv. 6, 54119–54128. doi: 10.1039/C6RA03317B
Cheng, W., Chen, X., Sun, J., Wang, J., and Zhang, S. (2013). SBA-15 supported triazolium-based ionic liquids as highly efficient and recyclable catalysts for fixation of CO2 with epoxides. Catal. Today 200, 117–124. doi: 10.1016/j.cattod.2012.10.001
Chum, H. L., and Overend, R. P. (2001). Biomass and renewable fuels. Fuel Process. Technol. 71, 187–195. doi: 10.1016/S0378-3820(01)00146-1
Claus, J., Sommer, F. O., and Kragl, U. (2018). Ionic liquids in biotechnology and beyond. Solid State Ionics 314, 119–128. doi: 10.1016/j.ssi.2017.11.012
Coenen, J. W. E. (1986). Catalytic hydrogenation of fatty oils. Indus. Eng. Chem. Fundamentals 25, 43–52. doi: 10.1021/i100021a006
Cota, I., and Martinez, F. F. (2017). Recent advances in the synthesis and applications of metal organic frameworks doped with ionic liquids for CO2 adsorption. Coordinat. Chem. Rev. 351, 189–204. doi: 10.1016/j.ccr.2017.04.008
Da'na, E. (2017). Adsorption of heavy metals on functionalized-mesoporous silica: a review. Microporous Mesoporous Mater. 247, 145–157. doi: 10.1016/j.micromeso.2017.03.050
Demirbas, A. (2006). Biodiesel production via non-catalytic SCF method and biodiesel fuel characteristics. Energy Convers. Manage. 47, 2271–2282. doi: 10.1016/j.enconman.2005.11.019
DeSantis, D., Mason, J. A., James, B. D., Houchins, C., Long, J. R., and Veenstra, M. (2017). Techno-economic analysis of metal-organic frameworks for hydro-gen and natural gas storage. Energy Fuels 31, 2024–2032. doi: 10.1021/acs.energyfuels.6b02510
Dincer, I. (2000). Renewable energy and sustainable development: a crucial review. Renew. Sustain. Energy Rev. 4, 157–175. doi: 10.1016/S1364-0321(99)00011-8
Dodds, D. R., and Gross, R. A. (2007). Chemicals from biomass. Science 318, 1250–1251. doi: 10.1126/science.1146356
Egorova, K. S., Gordeev, E. G., and Ananikov, V. P. (2017). Biological activity of ionic liquids and their application in pharmaceutics and medicine. Chem. Rev. 117, 7132–7189. doi: 10.1021/acs.chemrev.6b00562
Elhamifar, D., Karimi, B., Moradi, A., and Rastegar, J. (2014). Synthesis of a novel sulfonic acid containing ionic-liquid-based periodic mesoporous organosilica and study of its catalytic performance in the esterification of carboxylic acids. ChemPlusChem 79, 1147–1152. doi: 10.1002/cplu.201402071
Fan, M., Huang, J., Yang, J., and Zhang, P. (2013). Biodiesel production by transesterification catalyzed by an efficient choline ionic liquid catalyst. Appl. Energy 108, 333–339. doi: 10.1016/j.apenergy.2013.03.063
Fan, M., Liu, H., and Zhang, P. (2018). Ionic liquid on the acidic organic-inorganic hybrid mesoporous material with good acid-water resistance for biodiesel production. Fuel 215, 541–550. doi: 10.1016/j.fuel.2017.11.085
Fang, Z., Smith, R. L., and Qi, X. (2014). Production of Biofuels and Chemicals With Ionic Liquids. Dordrecht: Springer.
Fauzi, A. H. M., and Amin, N. A. S. (2012). An overview of ionic liquids as solvents in biodiesel synthesis. Renew. Sustain. Energy Rev. 16, 5770–5786. doi: 10.1016/j.rser.2012.06.022
Feng, Y., Li, L., Wang, X., Yang, J., and Qiu, T. (2017). Stable poly (ionic liquid) with unique crosslinked microsphere structure as efficient catalyst for transesterification of soapberry oil to biodiesel. Energy Convers. Manage. 153, 649–658. doi: 10.1016/j.enconman.2017.10.018
Fu, J., Detsi, E., and De Hosson, J. T. M. (2018). Recent advances in nanoporous materials for renewable energy resources conversion into fuels. Surf. Coat. Technol. 347, 320–336. doi: 10.1016/j.surfcoat.2018.05.001
Fujie, K., and Kitagawa, H. (2016). Ionic liquid transported into metal–organic frameworks. Coordinat. Chem. Rev. 307, 382–390. doi: 10.1016/j.ccr.2015.09.003
Gangu, K. K., Maddila, S., Makkamala, S. B., and Jonnalagadda, S. B. (2016). A review on contemporary Metal–Organic Framework materials. Inorgan. Chim. Acta 446, 61–74. doi: 10.1016/j.ica.2016.02.062
Gebremariam, S. N., and Marchetti, J. M. (2018). Economics of biodiesel production: review. Energy Convers. Manage. 168, 74–84. doi: 10.1016/j.enconman.2018.05.002
Ghiaci, M., Aghabarari, B., Habibollahi, S., and Gil, A. (2011). Highly efficient Bronsted acidic ionic liquid-based catalysts for biodiesel synthesis from vegetable oils. Bioresource Technol. 102, 1200–1204. doi: 10.1016/j.biortech.2010.09.095
Gholami, A., Hajinezhad, A., Pourfayaz, F., and Ahmadi, M. H. (2018). The effect of hydrodynamic and ultrasonic cavitation on biodiesel production: an exergy analysis approach. Energy 160, 478–489. doi: 10.1016/j.energy.2018.07.008
Gholami, A., Pourfayaz, F., Hajinezhad, A., and Mohadesi, M. (2019). Biodiesel production from Norouzak (Salvia leriifolia) oil using choline hydroxide catalyst in a microchannel reactor. Renew. Energy 136, 993–1001. doi: 10.1016/j.renene.2019.01.057
Granados, M. L., Poves, M. D. Z., Alonso, D. M., Mariscal, R., Galisteo, F. C., Moreno-Tost, R., et al. (2007). Biodiesel from sunflower oil by using activated calcium oxide. Appl. Catal. B Environ. 73, 317–326. doi: 10.1016/j.apcatb.2006.12.017
Han, M., Gu, Z., Chen, C., Wu, Z., Que, Y., Wang, Q., et al. (2016). Efficient confinement of ionic liquids in MIL-100(Fe) frameworks by the “impregnation-reaction-encapsulation” strategy for biodiesel production. RSC Adv. 6, 37110–37117. doi: 10.1039/C6RA00579A
Han, M., Li, Y., Gu, Z., Shi, H., Chen, C., Wang, Q., et al. (2018). Immobilization of thiol-functionalized ionic liquids onto the surface of MIL-101(Cr) frameworks by SCr coordination bond for biodiesel production. Colloids Surf. A Physicochem. Eng. Aspects 553, 593–600. doi: 10.1016/j.colsurfa.2018.05.085
Han, X. X., He, Y. F., Hung, C. T., Liu, L. L., Huang, S. J., and Liu, S. B. (2013). Efficient and reusable polyoxometalate-based sulfonated ionic liquid catalysts for palmitic acid esterification to biodiesel. Chem. Eng. Sci. 104, 64–72. doi: 10.1016/j.ces.2013.08.059
Hasheminejad, M., Tabatabaei, M., Mansourpanah, Y., Khatami far, M., and Javani, A. (2011). Upstream and downstream strategies to economize biodiesel production. Bioresource Technol. 102, 461–468. doi: 10.1016/j.biortech.2010.09.094
Hu, H., Xin, J. H., Hu, H., Wang, X., and Kong, Y. (2015). Metal-free graphene-based catalyst—Insight into the catalytic activity: a short review. Appl. Catal. A General 492, 1–9. doi: 10.1016/j.apcata.2014.11.041
Hu, M., Yao, Z., and Wang, X. (2017). Graphene-based nanomaterials for catalysis. Indus. Eng. Chem. Res. 56, 3477–3502. doi: 10.1021/acs.iecr.6b05048
Jiang, B., Wang, Y., Zhang, L., Sun, Y., Yang, H., Wang, B., et al. (2017). Biodiesel production via transesterification of soybean oil catalyzed by superhydrophobic porous poly(ionic liquid) solid base. Energy Fuels 31, 5203–5214. doi: 10.1021/acs.energyfuels.7b00443
Karimi, B., and Vafaeezadeh, M. (2012). SBA-15-functionalized sulfonic acid confined acidic ionic liquid: a powerful and water-tolerant catalyst for solvent-free esterifications. Chem. Commun. 48, 3327–3329. doi: 10.1039/c2cc17702a
Khan, S. A., Rashmi, Hussain, M. Z., Prasad, S., and Banerjee, U. C. (2009). Prospects of biodiesel production from microalgae in India. Renew. Sustain. Energy Rev. 13, 2361–2372. doi: 10.1016/j.rser.2009.04.005
Kumar, D., Kumar, G., and Singh, P. C. P. (2010). Fast, easy ethanolysis of coconut oil for biodiesel production assisted by ultrasonication. Ultrasonics Sonochem. 17, 555–559. doi: 10.1016/j.ultsonch.2009.10.018
Lee, S. L., Wong, Y. C., Tan, Y. P., and Yew, S. Y. (2015). Transesterification of palm oil to biodiesel by using waste obtuse horn shell-derived CaO catalyst. Energy Convers. Manage. 93, 282–288. doi: 10.1016/j.enconman.2014.12.067
Li, M., Zhang, W., Zhou, S., and Zhao, Y. (2020). Preparation of poly(vinylalcohol)/palygorskite-poly(ionic liquids) hybrid catalytic membranes to facilitate esterification. Separat. Purif. Technol. 230:115746. doi: 10.1016/j.seppur.2019.115746
Liang, X. (2013a). Novel acidic ionic liquid polymer for biodiesel synthesis from waste oils. Appl. Catal. A General 455, 206–210. doi: 10.1016/j.apcata.2013.01.036
Liang, X. (2013b). Novel efficient procedure for biodiesel synthesis from waste oils using solid acidic ionic liquid polymer as the catalyst. Indus. Eng. Chem. Res. 52, 6894–6900. doi: 10.1021/ie303564b
Liang, X. (2014). Novel ionic liquid supported on a magnetic core and its catalytic activities. Indus. Eng. Chem. Res. 53, 17325–17332. doi: 10.1021/ie502681w
Liu, C. Z., Wang, F., Stiles, A. R., and Guo, C. (2012). Ionic liquids for biofuel production: opportunities and challenges. Appl. Energy 92, 406–414. doi: 10.1016/j.apenergy.2011.11.031
Liu, F., Kong, W., Wang, L., Yi, X., Noshadi, I., Zheng, A., et al. (2015). Efficient biomass transformations catalyzed by graphene-like nanoporous carbons functionalized with strong acid ionic liquids and sulfonic groups. Green Chem. 17, 480–489. doi: 10.1039/C4GC01052C
Liu, F., Wang, L., Sun, Q., Zhu, L., Meng, X., and Xiao, F. S. (2012). Transesterification catalyzed by ionic liquids on superhydrophobic mesoporous polymers: heterogeneous catalysts that are faster than homogeneous catalysts. J. Am. Chem. Soc. 134, 16948–16950. doi: 10.1021/ja307455w
Luo, H., Fan, W., Li, Y., and Nan, G. (2013). Biodiesel production using alkaline ionic liquid and adopted as lubricity additive for low-sulfur diesel fuel. Bioresource Technol. 140, 337–341. doi: 10.1016/j.biortech.2012.11.112
Mansir, N., Teo, S. H., Rashid, U., Saiman, M. I., Tan, Y. P., Alsultan, G. A., et al. (2018). Modified waste egg shell derived bifunctional catalyst for biodiesel production from high FFA waste cooking oil. A review. Renew. Sustain. Energy Rev. 82, 3645–3655. doi: 10.1016/j.rser.2017.10.098
McKendry, P. (2002). Energy production from biomass (part 1): overview of biomass. Bioresour. Technol. 83, 37–46. doi: 10.1016/S0960-8524(01)00118-3
Mekhilef, S., Siga, S., and Saidur, R. (2011). A review on palm oil biodiesel as a source of renewable fuels. Renew. Sustain. Energy Rev. 15, 1937–1949. doi: 10.1016/j.rser.2010.12.012
Montalban, M. G., Villora, G., and Licence, P. (2018). Ecotoxicity assessment of dicationic versus monocationic ionic liquids as a more environmentally friendly alternative. Ecotoxicol. Environ. Safety 150, 129–135. doi: 10.1016/j.ecoenv.2017.11.073
Montgomery, D. C. (2013). Design and Analysis of Experiments, 8th Edn. Hoboken, NJ: John Wiley & Sons, Inc.
Monyem, A., and Van Gerpen, J. H. (2001). The effect of biodiesel oxidation on engine performance and emissions. Biomass Bioenergy 20, 317–325. doi: 10.1016/S0961-9534(00)00095-7
Muhammad, N., Elsheikh, Y. A., Abdul Mutalib, M. I., Bazmi, A. A., Khan, R. A., Khan, H., et al. (2015). An overview of the role of ionic liquids in biodiesel reactions. J. Indus. Eng. Chem. 21, 1–10. doi: 10.1016/j.jiec.2014.01.046
Nabi, M. N., Akhter, M. S., and Shahadat, M. M. Z. (2006). Improvement of engine emissions with conventional diesel fuel and diesel-biodiesel blends. Bioresour. Technol. 97, 372–378. doi: 10.1016/j.biortech.2005.03.013
Nagarajan, S., Chou, S. K., Cao, S., Wu, C., and Zhou, Z. (2013). An updated comprehensive techno-economic analysis of algae biodiesel. Bioresource Technol. 145, 150–156. doi: 10.1016/j.biortech.2012.11.108
Noshadi, I., Kanjilal, B., Du, S., Bollas, G. M., Suib, S. L., Provatas, A., et al. (2014). Catalyzed production of biodiesel and bio-chemicals from brown grease using ionic liquid functionalized ordered mesoporous polymer. Appl. Energy 129, 112–122. doi: 10.1016/j.apenergy.2014.04.090
Novoselov, K. S., Geim, A. K., Morozov, S. V., Jiang, D., Zhang, Y., Dubonos, S. V., et al. (2004). Electric field effect in atomically thin carbon films. Science 306, 666–669. doi: 10.1126/science.1102896
Olkiewicz, M., Torres, C. M., Jimenez, L., Font, J., and Bengoa, C. (2016). Scale-up and economic analysis of biodiesel production from municipal primary sewage sludge. Bioresource Technol. 214, 122–131. doi: 10.1016/j.biortech.2016.04.098
Osada, I., de Vries, H., Scrosati, B., and Passerini, S. (2016). Ionic-liquid-based polymer electrolytes for battery applications. Angew. Chem. 55, 500–513. doi: 10.1002/anie.201504971
Pan, H., Li, H., Liu, X. F., Zhang, H., Yang, K. L., Huang, S., et al. (2016). Mesoporous polymeric solid acid as efficient catalyst for (trans)esterification of crude Jatropha curcas oil. Fuel Process. Technol. 150, 50–57. doi: 10.1016/j.fuproc.2016.04.035
Pan, H., Li, H., Zhang, H., Wang, A., and Yang, S. (2019). Acidic ionic liquid-functionalized mesoporous melamine-formaldehyde polymer as heterogeneous catalyst for biodiesel production. Fuel 239, 886–895. doi: 10.1016/j.fuel.2018.11.093
Pandey, S. (2006). Analytical applications of room-temperature ionic liquids: a review of recent efforts. Anal. Chim. Acta 556, 38–45. doi: 10.1016/j.aca.2005.06.038
Panwar, N. L., Kaushik, S. C., and Kothari, S. (2011). Role of renewable energy sources in environmental protection: a review. Renew. Sustain. Energy Rev. 15, 1513–1524. doi: 10.1016/j.rser.2010.11.037
Pei, B., Xiang, X., Liu, T., Li, D., Zhao, C., Qiu, R., et al. (2019). Preparation of chloromethylated pitch–based hyper–crosslinked polymers and an immobilized acidic ionic liquid as a catalyst for the synthesis of biodiesel. Catalysts 9:963. doi: 10.3390/catal9110963
Pirez, C., Caderon, J. M., Dacquin, J. P., Lee, A. F., and Wilson, K. (2012). Tunable KIT-6 mesoporous sulfonic acid catalysts for fatty acid esterification. ACS Catal. 2, 1607–1614. doi: 10.1021/cs300161a
Qian, W., Texter, J., and Yan, F. (2017). Frontiers in poly(ionic liquid)s: syntheses and applications. Chem. Soc. Rev. 46, 1124–1159. doi: 10.1039/C6CS00620E
Riisager, A., Fehrmann, R., Haumann, M., and Wasserscheid, P. (2006a). Supported ionic liquid phase (SILP) catalysis: an innovative concept for homogeneous catalysis in continuous fixed-bed reactors. Eur. J. Inorganic Chem. 4, 695–706. doi: 10.1002/ejic.200500872
Riisager, A., Fehrmann, R., Haumann, M., and Wasserscheid, P. (2006b). Supported ionic liquids: versatile reaction and separation media. Topics Catal. 40, 91–102. doi: 10.1007/s11244-006-0111-9
Rouquerol, J., Avnir, D., Fairbridge, C. W., Everett, D. H., Haynes, J. M., Pernicone, N., et al. (1994). Recommendations for the characterization of porous solids. Pure Appl. Chem. 66, 1739–1758. doi: 10.1351/pac199466081739
Safaei, M., Foroughi, M. M., Ebrahimpoor, N., Jahani, S., Omidi, A., and Khatami, M. (2019). A review on metal-organic frameworks: synthesis and applications. TrAC Trends Anal. Chem. 118, 401–425. doi: 10.1016/j.trac.2019.06.007
Sasidharan, M., Kiyozumi, Y., Mal, N. K., Paul, M., Rajamohanan, P. R., and Bhaumik, A. (2009). Incorporation of tin in different types of pores in SBA-15: Synthesis, characterization and catalytic activity. Microporous Mesoporous Mater. 126, 234–244. doi: 10.1016/j.micromeso.2009.05.038
Selvam, T., Machoke, A., and Schwieger, W. (2012). Supported ionic liquids on non-porous and porous inorganic materials-a topical review. Appl. Catal. A General 445–446, 92–101. doi: 10.1016/j.apcata.2012.08.007
Sharma, S., Saxena, V., Baranwal, A., Chandra, P., and Pandey, L. M. (2018). Engineered nanoporous materials mediated heterogeneous catalysts and their implications in biodiesel production. Mater. Sci. Energy Technol. 1, 11–21. doi: 10.1016/j.mset.2018.05.002
Sirisomboonchai, S., Abuduwayiti, M., Guan, G., Samart, C., Abliz, S., Hao, X., et al. (2015). Biodiesel production from waste cooking oil using calcined scallop shell as catalyst. Energy Convers. Manage. 95, 242–247. doi: 10.1016/j.enconman.2015.02.044
Su, C. H. (2013). Recoverable and reusable hydrochloric acid used as a homogeneous catalyst for biodiesel production. Appl. Energy 104, 503–509. doi: 10.1016/j.apenergy.2012.11.026
Sun, Q., Dai, Z., Meng, X., and Xiao, F. S. (2015). Porous polymer catalysts with hierarchical structures. Chem. Soc. Rev. 44, 6018–6034. doi: 10.1039/C5CS00198F
Tabatabaei, M., Aghbashlo, M., Dehghani, M., Panahi, H. K. S., Mollahosseini, A., Hosseini, M., et al. (2019). Reactor technologies for biodiesel production and processing: a review. Progress Energy Combust. Sci. 74, 239–303. doi: 10.1016/j.pecs.2019.06.001
Takase, M., Zhang, M., Feng, W., Chen, Y., Zhao, T., Cobbina, S. J., et al. (2014). Application of zirconia modified with KOH as heterogeneous solid base catalyst to new non-edible oil for biodiesel. Energy Convers. Manage. 80, 117–125. doi: 10.1016/j.enconman.2014.01.034
Tang, J., Liu, J., Li, C., Li, Y., Tade, M. O., Dai, S., et al. (2015). Synthesis of nitrogen-doped mesoporous carbon spheres with extra-large pores through assembly of diblock copolymer micelles. Angew. Chem. Int. Edn. 54, 588–593. doi: 10.1002/anie.201407629
Tesfa, B., Mishra, R., Gu, F., and Powles, N. (2010). Prediction models for density and viscosity of biodiesel and their effects on fuel supply system in CI engines. Renew. Energy 35, 2752–2760. doi: 10.1016/j.renene.2010.04.026
Tran, N. N., Tisma, M., Budzaki, S., McMurchie, E. J., Gonzalez, O. M. M., Hessel, V., et al. (2018). Scale-up and economic analysis of biodiesel production from recycled grease trap waste, Applied Energy, 229, 142–150. doi: 10.1016/j.apenergy.2018.07.106
Troter, D. Z., Todorovic, Z. B., Dokic-Stojanovic, D. R., Stamenkovic, O. S., and Veljkovic, V. B. (2016). Application of ionic liquids and deep eutectic solvents in biodiesel production: a review. Renew. Sustain. Energy Rev. 61, 473–500. doi: 10.1016/j.rser.2016.04.011
Ullah, Z., Bustam, M. A., and Man, Z. (2015). Biodiesel production from waste cooking oil by acidic ionic liquid as a catalyst. Renew. Energy 77, 521–526. doi: 10.1016/j.renene.2014.12.040
Ullah, Z., Khan, A. S., Muhammad, N., Ullah, R., Alqahtani, A. S., Shah, S. N., et al. (2018). A review on ionic liquids as perspective catalysts in transesterification of different feedstock oil into biodiesel. J. Mol. Liquids 266, 673–686. doi: 10.1016/j.molliq.2018.06.024
Vallette, H., Pican, S., Boudou, C., Levillain, J., Plaquevent, J. C., and Gaumont, A. C. (2006). Palladium catalyzed C-P cross-coupling reactions in ionic liquids. Tetrahedron Lett. 47, 5191–5193. doi: 10.1016/j.tetlet.2006.05.100
Valtchev, V., Mintova, S., and Tsapatsis, M. (2009). Ordered Porous Solids: Recent Advances and Prospects, 1st Edn. Oxford: Elsevier.
Van Gerpen, J. (2005). Biodiesel processing and production. Fuel Process. Technol. 86, 1097–1107. doi: 10.1016/j.fuproc.2004.11.005
Ventura, S. P. M., e Silva, F. A., Quental, M. V., Mondal, D., Freire, M. G., and Coutinho, J. A. P. (2017). Ionic-liquid-mediated extraction and separation processes for bioactive compounds: past, present, and future trends. Chem. Rev. 117, 6984–7052. doi: 10.1021/acs.chemrev.6b00550
Wan, H., Chen, C., Wu, Z., Que, Y., Feng, Y., Wang, W., et al. (2015b). Encapsulation of heteropolyanion-based ionic liquid within the metal–organic framework MIL-100(Fe) for biodiesel production. ChemCatChem 7, 441–449. doi: 10.1002/cctc.201402800
Wan, H., Wu, Z., Chen, W., Guan, G., Cai, Y., Chen, C., et al. (2015a). Heterogenization of ionic liquid based on mesoporous material as magnetically recyclable catalyst for biodiesel production. J. Mol. Catal. A Chem. 398, 127–132. doi: 10.1016/j.molcata.2014.12.002
Wang, H., Maiyalagan, T., and Wang, X. (2012). Review on recent Progress in nitrogen-doped graphene: synthesis, characterization, and its potential applications. ACS Catal. 2, 781–794. doi: 10.1021/cs200652y
Wang, Y., Wang, D., Tan, M., Jiang, B., Zheng, J., Tsubaki, N., et al. (2015). Monodispersed hollow SO3H-functionalized carbon/silica as efficient solid acid catalyst for esterification of oleic acid. ACS Appl. Mater. Interfaces 7, 26767–26775. doi: 10.1021/acsami.5b08797
Wang, Y., Zhao, D., Wang, L., Wang, X., Li, L., Xing, Z., et al. (2018). Immobilized phosphotungstic acid based ionic liquid: application for heterogeneous esterification of palmitic acid. Fuel 216, 364–370. doi: 10.1016/j.fuel.2017.11.153
Watanabe, M., Thomas, M. L., Zhang, S., Ueno, K., Yasuda, T., and Dokko, K. (2017). Application of ionic liquids to energy storage and conversion materials and devices. Chem. Rev. 117, 7190–7239. doi: 10.1021/acs.chemrev.6b00504
Wu, Z., Chen, C., Guo, Q., Li, B., Que, Y., Wang, L., et al. (2016a). Novel approach for preparation of poly (ionic liquid) catalyst with macroporous structure for biodiesel production. Fuel 184, 128–135. doi: 10.1016/j.fuel.2016.07.004
Wu, Z., Chen, C., Wan, H., Wang, L., Li, Z., Li, B., et al. (2016b). Fabrication of magnetic NH2-MIL-88B (Fe) confined Brønsted ionic liquid as an efficient catalyst in biodiesel synthesis. Energy Fuels 30, 10739–10746. doi: 10.1021/acs.energyfuels.6b01212
Wu, Z., Chen, C., Wang, L., Wan, H., and Guan, G. (2016c). Magnetic material grafted poly(phosphotungstate-based acidic ionic liquid) as efficient and recyclable catalyst for esterification of oleic acid. Indus. Eng. Chem. Res. 55, 1833–1842. doi: 10.1021/acs.iecr.5b02906
Xie, W., Hu, L., and Yang, X. (2015a). Basic ionic liquid supported on mesoporous SBA-15 silica as an efficient heterogeneous catalyst for biodiesel production. Indus. Eng. Chem. Res. 54, 1505–1512. doi: 10.1021/ie5045007
Xie, W., and Wan, F. (2018). Basic ionic liquid functionalized magnetically responsive Fe3O4@HKUST-1 composites used for biodiesel production. Fuel 220, 248–256. doi: 10.1016/j.fuel.2018.02.014
Xie, W., and Wan, F. (2019). Immobilization of polyoxometalate-based sulfonated ionic liquids on UiO-66-2COOH metal-organic frameworks for biodiesel production via one-pot transesterification-esterification of acidic vegetable oils. Chem. Eng. J. 365, 40–50. doi: 10.1016/j.cej.2019.02.016
Xie, W., and Wang, H. (2020). Immobilized polymeric sulfonated ionic liquid on core-shell structure Fe3O4/SiO2 composites: a magnetically recyclable catalyst for simultaneous transesterification and esterifications of low-cost oils to biodiesel. Renew. Energy 145, 1709–1719. doi: 10.1016/j.renene.2019.07.092
Xie, W., Yang, X., and Fan, M. (2015b). Novel solid base catalyst for biodiesel production: mesoporous SBA-15 silica immobilized with 1,3-dicyclohexyl-2-octylguanidine. Renew. Energy 80, 230–237. doi: 10.1016/j.renene.2015.02.014
Yaakob, Z., Narayanan, B. N., Padikkaparambil, S., Unni, K. S., and Akbar, P. M. (2014). A review on the oxidation stability of biodiesel. Renew. Sustain. Energy Rev. 35, 136–153. doi: 10.1016/j.rser.2014.03.055
Ye, C., Qi, Z., Cai, D., and Qiu, T. (2019). Design and synthesis of ionic liquid supported hierarchically porous Zr metal–organic framework as a novel Brønsted–Lewis acidic catalyst in biodiesel synthesis. Indus. Eng. Chem. Res. 58, 1123–1132. doi: 10.1021/acs.iecr.8b04107
Yuan, J., and Antonietti, M. (2011). Poly(ionic liquid)s: Polymers expanding classical property profiles. Polymer 52, 1469–1482. doi: 10.1016/j.polymer.2011.01.043
Yuan, S., Wang, M., Liu, J., and Guo, B. (2020). Recent advances of SBA-15-based composites as the heterogeneous catalysts in water decontamination: a mini-review. J. Environ. Manage. 254:109787. doi: 10.1016/j.jenvman.2019.109787
Yusuf, N. N. A. N., and Kamarudin, S. K. (2013). Techno-economic analysis of biodiesel production from Jatropha curcas via a supercritical methanol process. Energy Convers. Manage. 75, 710–717. doi: 10.1016/j.enconman.2013.08.017
Zhang, H., Li, H., Pan, H., Wang, A., Souzanchi, S., Xu, C., et al. (2018). Magnetically recyclable acidic polymeric ionic liquids decorated with hydrophobic regulators as highly efficient and stable catalysts for biodiesel production. Appl. Energy 223, 416–429. doi: 10.1016/j.apenergy.2018.04.061
Zhang, H., Li, H., Pan, H., Wang, A., Xu, C., and Yang, S. (2017). Magnetically recyclable basic polymeric ionic liquids for efficient transesterification of Firmiana platanifolia, L.f. oil into biodiesel. Energy Convers. Manage. 153, 462–472. doi: 10.1016/j.enconman.2017.10.023
Zhang, L., Cui, Y., Zhang, C., Wang, L., Wan, H., and Guan, G. (2012). Biodiesel production by esterification of oleic acid over Bronsted acidic ionic liquid supported onto Fe-incorporated SBA-15. Indus. Eng. Chem. Res. 51, 16590–16596. doi: 10.1021/ie302419y
Zhang, W., Li, M., Wang, J., Zhao, Y., Zhou, S., and Xing, W. (2017). Heterogeneous poly(ionic liquids) catalyst on nanofiber-like palygorskite supports for biodiesel production. Appl. Clay Sci. 146, 167–175. doi: 10.1016/j.clay.2017.05.031
Zhang, Y., Jiao, Q., Zhen, B., Wu, Q., and Li, H. (2013). Transesterification of glycerol trioleate catalyzed by basic ionic liquids immobilized on magnetic nanoparticles. Appl. Catal. A General 453, 327–333. doi: 10.1016/j.apcata.2012.12.029
Zhen, B., Jiao, Q., Wu, Q., and Li, H. (2014). Catalytic performance of acidic ionic liquid-functionalized silica in biodiesel production. J. Energy Chem. 23, 97–104. doi: 10.1016/S2095-4956(14)60122-4
Zhen, B., Jiao, Q., Zhang, Y., Wu, Q., and Li, H. (2012). Acidic ionic liquid immobilized on magnetic mesoporous silica: preparation and catalytic performance in esterification. Appl. Catal. A General 445–446, 239–245. doi: 10.1016/j.apcata.2012.08.023
Keywords: biodiesel, nanoporous materials, ionic liquids, catalyst, transesterification
Citation: Gholami A, Pourfayaz F and Maleki A (2020) Recent Advances of Biodiesel Production Using Ionic Liquids Supported on Nanoporous Materials as Catalysts: A Review. Front. Energy Res. 8:144. doi: 10.3389/fenrg.2020.00144
Received: 27 February 2020; Accepted: 11 June 2020;
Published: 17 July 2020.
Edited by:
Mohammad Rehan, King Abdulaziz University, Saudi ArabiaReviewed by:
Steven Lim, Tunku Abdul Rahman University, MalaysiaCopyright © 2020 Gholami, Pourfayaz and Maleki. This is an open-access article distributed under the terms of the Creative Commons Attribution License (CC BY). The use, distribution or reproduction in other forums is permitted, provided the original author(s) and the copyright owner(s) are credited and that the original publication in this journal is cited, in accordance with accepted academic practice. No use, distribution or reproduction is permitted which does not comply with these terms.
*Correspondence: Fathollah Pourfayaz, cG91cmZheWF6QHV0LmFjLmly
Disclaimer: All claims expressed in this article are solely those of the authors and do not necessarily represent those of their affiliated organizations, or those of the publisher, the editors and the reviewers. Any product that may be evaluated in this article or claim that may be made by its manufacturer is not guaranteed or endorsed by the publisher.
Research integrity at Frontiers
Learn more about the work of our research integrity team to safeguard the quality of each article we publish.