- Center of Excellence in Transportation Electrification and Energy Storage, Hydro-Québec, Varennes, QC, Canada
Sodium-ion batteries offer a promising alternative to lithium-ion batteries due to their low cost, environmental friendliness, high abundance of sodium, and established electrochemical process. However, problems, such as low capacity, low storage voltage and capacity fade of electrode materials, must be resolved for the applications of sodium ion batteries. Many Ti-containing compounds were reported as cathode and anode materials, but very few studies focus on the role of Ti in electrodes used in sodium-ion batteries. This paper systemically reviews the roles of Ti in electrodes of sodium ion batteries. The Ti4+/Ti3+ redox couple is a good choice for anodes due to its low potential and it exhibits different storage voltages in different structures. Although Ti4+ does not participate in charge transfer in cathodes, it can indirectly enhance the capacity, cycling life and rate performance via structure change, cation order-disorder transition, and its interaction with the crystal lattice structure. This review will provide a new insight in designing and understanding novel high-performance electrodes.
Introduction
Due to environmental concerns associated with gasoline engines, electric vehicles (EVs) powered by lithium-ion batteries are becoming increasingly popular (Armand and Tarascon, 2008). However, the high cost and safety issues of lithium-ion batteries (LIB) are limiting their practical applications (Goodenough and Kim, 2009) and provide an opportunity for other batteries to meet the needs of energy storage (Dunn et al., 2011; Yang et al., 2011; Wang et al., 2018a). Sodium-ion batteries (SIB) have the advantage of an abundant sodium resource and compatibility with an aluminum current collector for both cathodes and anodes, which reduce battery cost and make them a promising alternative to lithium ion batteries (Pan et al., 2013a; Slater et al., 2013).
Research on sodium-ion batteries began in the early 1980's (Delmas et al., 1980), but the successful commercialization of lithium ion batteries in 1990 distracted the attention from research and development of SIB (Ellis and Nazar, 2012). Since 2010, numerous novel electrode materials for sodium-ion batteries have been reported. The cathodes are classified according to their structure, namely, Prussian blue, NASICON, olivine, tunnel-type, and oxides. Prussian blue analogs are attractive cathodes for sodium-ion batteries due to their open channel-like structure, compositional and electrochemical tenability. But water control during synthesis and the potential toxicity from cyanide release limit their applications (Wessells et al., 2011a,b). NASICON compounds, such as Na3V2(PO4)3 and Na3V2(PO4)2F3, were also used as electrodes for sodium-ion batteries. Na3V2(PO4)3/C cathode has a voltage plateau of 3.4 V with capacity of ~100 mAh/g, excellent rate capacity and cycle stability (Jian et al., 2012), whereas Na3V2(PO4)2F3 compounds possess two plateaus at 3.6 and 4.1 V as well as a capacity of 100 mAh/g in the 2.5–4.3 V voltage range. Although these cathodes exhibit promising Na storage performance, the highly toxic V3+/V5+ and the possibility of releasing fluorine gas becomes an environmental issue in large-scale production (Gover et al., 2006). Unlike LiFePO4, the olivine NaFePO4 is thermally unstable in ambient environment and has to be produced (Kim et al., 2015) by the ion exchange of Li+ in olivine LiFePO4 with Na+, which complicates the production and increases cost (Oh et al., 2012). The commercial success of layered-LiCoO2 in lithium-ion batteries (Mizushima et al., 1980) has prompted extensive investigation of its sodium counterpart NaxTMO2 (TM: Ni, Mn, Co, Ti) which crystallize into layered or tunneled structures depending on sodium content. The layered-structure consists of TM-O and Na-O layers. Compared to Li+, Na+ has a larger radius and stronger interaction with O2−. The sodium-layered structure is further divided into O3, P3, P2, and O2. The O and P represent the octahedral and trigonal prismatic coordination environment of alkali ions, respectively; 3 and 2 describe the number of TM layers of repeated stacking (Delmas et al., 1980). The oxides P2/O3-NaxCoO2, NaxMnO2 NaxVO2 and NaxNiMnO2 etc. are also investigated (Delmas et al., 1981; Braconnier et al., 1982; Maazaz et al., 1983; Miyazaki et al., 1983; Jeong and Manthiram, 2001; Lu and Dahn, 2001), and some of the plateaus in the charge/discharge profile are attributed to Na+/vacancy ordering, which affects the rate and cycling performance. Electrodes-containing Ti, such as Na0.6Cr0.6Ti0.4O2, Na2/3Ni1/3Mn1/2Ti1/6O2, Na0.8Ni0.4Ti0.6O2, and Na0.67Co0.33Ti0.67O2, etc., show a smooth sloping curve during sodium insertion and extraction, suggesting that Ti-substitution interrupted the Na+/vacancy ordering (Yoshida et al., 2014; Yu et al., 2014; Guo et al., 2015a; Wang et al., 2015c). Tunnel-type Na0.44MnO2 was first proposed as a cathode by Doeff et al. (1994). It has a unique structure that prevents interaction with H3O+, which is useful as a cathode or anode for non-aqueous and aqueous sodium-ion batteries (Whitacre et al., 2010; Hosono et al., 2012). Ti- substitution for Mn in Na0.44MnO2 smooth's its multi-plateaus discharge-charge curve (Wang et al., 2015a).
The carbon-based compounds (Alcántara et al., 2001; Li et al., 2015, 2016b; Zheng et al., 2017; Wang et al., 2018b), alloys (Xiao et al., 2012; Farbod et al., 2014; Lim et al., 2017), organic compounds (Wang et al., 2014; Wu et al., 2015d), sulfides (Newman and Klemann, 1980), phosphates (Wu et al., 2013; Pang et al., 2014), and oxides (Senguttuvan et al., 2011; Trinh et al., 2012; Pan et al., 2013b; Sun et al., 2013; Wang et al., 2013; Xu et al., 2014; Zhao et al., 2015; Ding et al., 2017) are widely investigated as anodes. Hard carbon, obtained at high temperatures (1,600°C), exhibits a high reversible capacity of 330 mAh/g at a rate of 0.1C in the range of 0–2.5 V (Lu et al., 2018; Wang et al., 2018b; Zhao et al., 2018b). The near zero voltage vs. Na+/Na may result in sodium metal deposition on a hard carbon surface caused by improper battery operation or fast charging, which is a major safety concern (Chevrier and Ceder, 2011). In addition, low initial columbic efficiency and relatively high cost need to be resolved for practical application (Zhang et al., 2016). Although alloys, such as Sb/C (610 mAh/g) (Darwiche et al., 2012; Wu et al., 2014), SnSb/C (544 mAh/g) (Xiao et al., 2012), P (1,800 mAh/g) (Ramireddy et al., 2015), deliver high reversible capacities, the large volume change during cycling leads to electrode instability, a common problem in lithium-ion batteries too (Goodenough and Kim, 2009; Chevrier and Ceder, 2011). Organic compounds, such as Na2C8H4O4, Na2C16H10O4 etc., are also actively explored for sodium-ion batteries due to their low cost and high performance (Zhao et al., 2012b; Wang et al., 2014; Wu et al., 2015d). But the poor electronic conductivity, low initial coulombic efficiency, and poor cyclic stability still need to be improved. Sulfide electrodes, for example, TiS2 and MoS2, with ~200 mAhg−1 at the storage voltage of 2.0 V, have been investigated since 1976, but the voltage is slightly higher for the anode and the samples are sensitive to air (Silbernagel and Whittingham, 1976; Newman and Klemann, 1980; Hu et al., 2014). NASICON-type NaTi2(PO4)3, obtained by Ti4+/Ti3+ conversion, exhibits a reversible Na storage capacity of ca. 120 mAh/g at 2.1 V vs. Na+/Na, which is suitable as an anode for aqueous sodium-ion batteries (Park et al., 2011; Li et al., 2013; Wang et al., 2015b). Metal-oxides, e.g., Fe2O3, Sb2O4 and MnO2 etc. demonstrate a capacity around 200 mAh/g; however, the conversion reaction involved in charge transfer may result in low initial coulombic efficiency and electrode instability (Reddy and Reddy, 2004; Sun et al., 2011; Jian et al., 2014). Ti-containing oxides, such as Li4Ti5O12, attracted a lot of attention due to their high reversibility in lithium-ion batteries (Ferg et al., 1994; Wagemaker et al., 2008). Research on Ti-containing oxides has expanded since 2010. For example, monoclinic Na2Ti3O7 (Senguttuvan et al., 2011); spinel Li4Ti5O12 (Zhao et al., 2012a), P2-layered Na0.66Li0.22Ti0.78O2 (Wang et al., 2013), O3-type Na0.68Mg0.34Ti0.66O2 (Zhao et al., 2018a), Anatase/Rutile TiO2 (Wu et al., 2015b; Lan et al., 2017), NaTiOPO4 (Mu et al., 2016) and NaTiO2 (Wu et al., 2015a). All of these oxides exhibit Ti3+/Ti4+ charge transfer, but the sodium storage voltages are very different.
Herein, we focused on the roles of Ti in electrodes for sodium-ion batteries. Various Ti-containing compounds are shown in Figure 1. In anode materials, Ti4+/Ti3+ participate in charge transfer and demonstrate different electrochemical performances corresponding to their different structures. In the cathode, the doped Ti4+ in NaxTMO2 oxides does not participate in charge transfer. However, Ti-substitution results in a structural change that interrupts the Na+/vacancy orderings, which in turn affects the capacity, cycling and rate performance. This review provides a deep understanding of the Ti's roles in the development of novel materials for sodium-ion batteries.
Anode Materials
Titanium dioxides with different polymorphs, such as anatase, rutile, TiO2 (B) and amorphous, have been explored as anode materials for sodium ion batteries due to their high theoretical capacity of 335 mAh/g, high rate performance, good cyclability, non-toxicity and low cost (Xiong et al., 2011; Wu et al., 2015b; Lan et al., 2017; Li et al., 2017; He et al., 2018). In addition, Density Function Theory (DFT) calculations show that the energy required for insertion of two Na+ into unit cells of anatase and rutile are 11.10 and 20.65 eV, respectively. The difference in the energy values indicate an easier and faster Na+ intercalation as well as diffusion in anatase than in rutile, which is due to the presence of 2D tunnels in the anatase structure (Su et al., 2015). As such, the electrochemical performance of anatase is better than rutile. On the other hand, DFT calculations predict that introduction of defects in the structure can narrow the energy gap in rutile, increase the electronic conductivity and Na+ diffusivity, as well as the rate performance, which are proved by experimental results (Chen et al., 2015; Usui et al., 2015; He et al., 2017).
TiO2
Anatase
Anatase TiO2 has tetragonal unit cell with space group (S.G.) I41/amd. Its crystal structure is built on corner and edge sharing of TiO6 octahedra. There are two dimensional tunnels along its a and b axes, which facilitate Na+ diffusion and accommodation. Despite the reduction of titanium ion to metal (Ti0) detected on the electrode surfaces, it is attributed to the side reactions at the electrolyte/electrode interface, whereas the Ti4+ ↔ Ti3+ redox reaction is considered as the contribution to the reversible capacity of the electrode. Although different shapes of sodium discharge/charge curves (with no obvious potential plateaus and with mulita-plateaus) are reported, the investigations on the reaction mechanism suggest that the capacity observed at the high potential section (> ~0.8 V) during discharge is mainly associated to the pseudo-capacitive process as well as the side reactions and SEI layer formation. Further discharge to 0.01/0.1 V, the crystallinity of anatase decreases greatly due to the insertion of Na+ in the octahedral sites in the crystal structure. This crystallinity to amorphous/partial amorphous transition is irreversible upon the extraction of Na ions during charge (Wu et al., 2015b; Li et al., 2017). In fact, certain amounts of Na ions still rest in the host crystal structure after charge, the estimated reversible Na+ insertion/extraction is ~0.41 Na+ per TiO2 (Vcutoffdischarge = 0.1 V) corresponding to ~140 mAh/g (Wu et al., 2015c). On the other hand, the preservation of anatase's crystallinity during Na+ insertion is also reported, even to a low voltage of 0.01 V (Kim et al., 2014) and 0 V (González et al., 2014). In spite of the discrepancies concerning the crystallinity of anatase during cycling, the working mechanism of the electrode is agreed to be the redox reaction between Ti4+ and Ti3+ introduced by Na+ insertion/extraction, see Figure 2A.
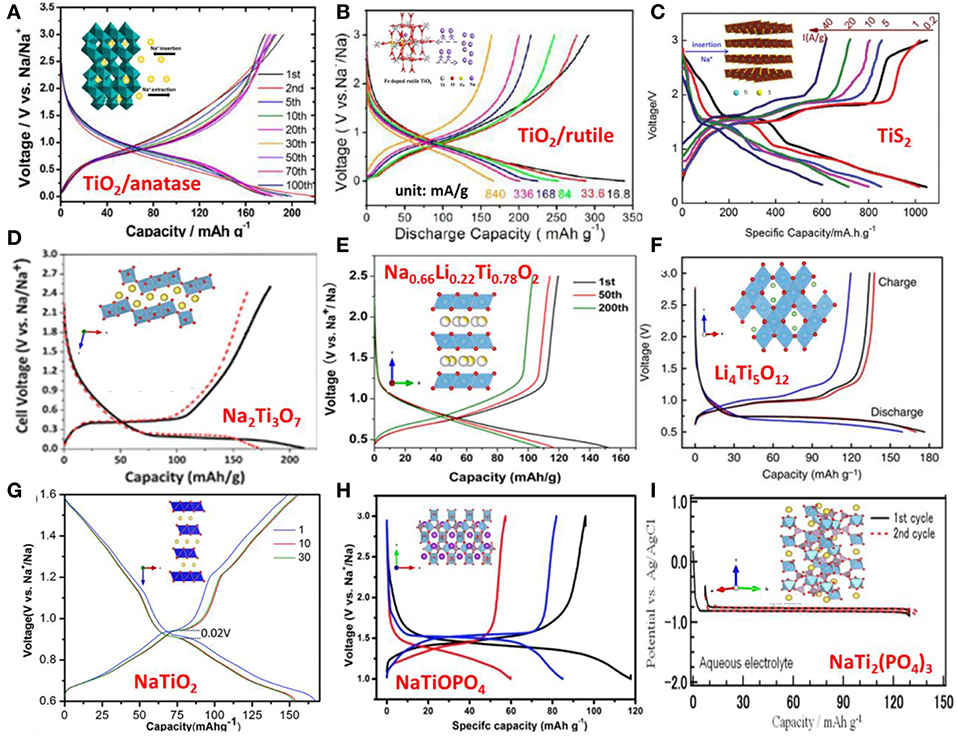
Figure 2. The structure and charge–discharge profiles of (A) Anatase TiO2, with permission from ACS; (B) Rutitle TiO2, with permission from ACS; (C) TiS2, With permission from Wiley; (D) Na2Ti3O7; with permission from RSC. (E) Na0.66Li0.22Ti0.78O2; with permission from Springer. (F) Li4Ti5O12; with permission from Springer. (G) NaTiO2; with permission from ACS. (H) NaTiOPO4; with permission from RSC. (I) NaTi2(PO4)3. With permission from ECS.
Rutile
The rutile TiO2 crystal has a tetragonal unit cell with S.G. P42/mnm, with the Ti4+ ions surrounded by an octahedron of 6 oxygen atoms. The TiO6 octahedrons are connected with edge and corner sharing. The rutile structure has a one-dimensional tunnel that enables the Na+ to diffuse through and accommodates them, making rutile a candidate anode material for Na-ion batteries. Despite this, rutile has good cycling stability and high sodium storage performance, and it is rarely studied compared to its counterpart, anatase, due to slow sodium diffusion (Usui et al., 2015) and its very low electronic conductivity (Chen et al., 2015). Substitution of Ti4+ with non-tetravalent cations is considered an effective way to enhance Na+ diffusivity and electric conductivity owing to the increased amount of vacancies. In fact, the measured electronic conductivities of doped rutile are 3–4 orders higher than the un-doped one, which agrees to the decreased band gap energy predicated by DFT calculation and leads to the high rate performance. The Fe and Nb doped rutile anodes were investigated and they showed high capacity (Fe-TiO2, 327.1 mAh/g at 16.8 mA/g) and high rate performance (Nb-TiO2, 120 mAh/g at 16.75A/g) (Usui et al., 2015; He et al., 2017). Their galvanostatic discharge-charge profiles show rounded potential shoulders below 1 V which are attributed to the Ti4+/Ti3+ redox reaction due to reversible Na+ insertion and extraction in the rutile structure, whereas the part above is assigned to the pseudo-capacitive sodium ion adsorption as well as the side reactions and SEI formation. XRD analysis demonstrated that (1) crystal structure change of rutile is reversible and its crystallinity is maintained during cycling; Na ions rest in the octahedral sites, formed by corner-sharing of the octahedrons, during discharge leading to the lattice expansion in a axis direction; whereas a axis contraction is observed during charge; (2) no Na2O and Ti metal are observed during discharge. Based on the analysis, the working mechanism of rutile is considered not based on conversion reaction, but based on intercalation/de-intercalation of Na+, similar to the case of anatase, Figure 2B.
TiS2
TiS2 has been investigated as an anode material for sodium ion battery for similar reasons as TiO2. Four electrons can be transferred through electrochemical conversion of TiS2 to metallic Ti, leading to a theoretical capacity of 957 mA h/g (Tao et al., 2018).
TiS2 has a layered structure with a van der Waals force bounding the TiS2 layers. Every layer contains two hexagonally closed packed S atoms sublayers with Ti4+ sandwiched in between. Ti4+ is located at the center of S octahedron and each sulfide is connected to three Ti4+ ions. TiS2 crystallizes into a hexagonal lattice with space group PAs an anode material of sodium ion battery; the weak van der Waals force between TiS2 layers facilitates the Na+ intercalation/de-intercalation and diffusion during discharge/charge. The working mechanism of TiS2 electrode has been studied in the voltage window of 0.3 to 3 V. The phase transformation is proposed as a reversible stepwise intercalation followed by a conversion reaction:
Some intermediate compounds also appeared before forming NaTiS2, such as Na0.22TiS2 and Na0.55TiS2. Obviously, after activation of the electrode material in the initial cycle, Ti4+ was reduced to a lower valence state (Ti0) in the subsequent cycles, whereas conversion of NaTiS2 to Timetal and Na2S was not seen with the cut off voltage of 1 V (Liu et al., 2016). DFT calculations proposed that TiS2 is a semi-conductor in nature whereas NaTiS2 and Ti0.77S are more metallic, which can enhance the electronic conductivity of the electrode, leading to the excellent rate capacity of 621 mAh/g at 40 A/g (Tao et al., 2018), Figure 2C.
Spinel-Li4Ti5O12
The spinel Li4Ti5O12, a well-known “zero-strain” anode material for long-life lithium-ion batteries (Ferg et al., 1994; Chen et al., 2001; Wagemaker et al., 2008), is considered a promising anode for sodium ion batteries. The spinel Li4Ti5O12 follows a typical bi-phase reaction mechanism and exhibits a flat voltage plateau of 1.55 V vs. Li+/Li and high rate performance in lithium-ion batteries.
Hu et al. first investigated Li4Ti5O12 as anode material for sodium-ion batteries in 2013 (Sun et al., 2013). The spinel-Li4Ti5O12 (shown in Figure 2F) has a cubic lattice; its symmetry is described by Fd-3m space group with eight formula units of (Li)8a[Li1/3Ti5/3]16d(O4)32e. Each unit cell consists of three sub-lattices sites, 8a, 16d, and 32e. The Li and O ions completely occupy the tetrahedral 8a sites and the octahedral 32e sites, respectively, while the octahedral 16d sites are randomly occupied by Li and Ti ions at the ratio of 1:5. During Li intercalation, the Li+ ions originally reside on the 8a sites, together with the newly incorporated Li+ ions, fill the 16c sites (Samin et al., 2015). In sodium-ion batteries, Li4Ti5O12 exhibits a stable specific capacity of 155 mAh/g with coulombic efficiency of >99% at a sodium storage voltages of 0.93 V and current rate of 0.1C. This storage voltage is higher than the sodium metal deposition voltage, making it safer than hard carbon. Hu et al. combined density DFT calculations, in-situ synchrotron XRD and scanning transmission electron microscope (STEM) imaging techniques to predict and confirm the three-phase separation mechanism, which was different from the bi-phase reaction mechanism in lithium-ion batteries. Upon discharge, Na+ ions will occupy the 16c sites exclusively to form a Na6Li phase, and at the same time, Li8a ions are pushed to the nearest neighbor Li4Ti5O12 (Li4) phase to form Li7Ti5O12 (Li7) phase which is equivalent to a lithium insertion process. In this manner two new phases, Na6Li and Li7, are created. As discharge continues without further nucleation of Na6Li, sodium insertion will proceed on the Na6Li/Li7 boundary, resulting in the transformation of Li to a Na6Li phase and pushing this boundary forward. Meanwhile, the Li16c ions from the initial Li7 phase will diffuse into the nearby Li4 phase to form Li7 and thus the Li7/Li4 boundary proceeds. Although the operating voltage and high capacity are attractive, three-phase separation causes electrode cracking and delaminating from the current collector, adversely affecting cycle life. Furthermore, a full battery cell with the spinel-Li4Ti5O12 as an anode and Na3V2(PO4)3/C as a cathode has been tested. It displays an average operating voltage plateau at ~2.4 V and delivers 135 mAh/g based on mass of anode. The electrochemical performance can be further enhanced by optimizing both electrodes and their weight ratio (Sun et al., 2013).
P2-Type Layered Oxides
Hu et al. explored a new “zero-strain” anode Na0.66[Li0.22Ti0.78]O2 (as shown in Figure 2E) with a P2-layered structure, which is an analog to Li4Ti5O12, as a promising electrode material for room-temperature sodium-ion batteries (Wang et al., 2013). In this structure, the large sodium ions occupy the trigonal prismatic sites in one layer, whereas smaller Li and Ti ions co-occupy the neighboring layer. In addition, some of the Na ions (0.425) occupy the 2d site sharing the edges with the TiO6 octahedra, whereas others (0.211) occupy the 2b site sharing two faces with the TiO6 octahedra. This situation is similar to other P2 materials. The reversible capacity of Na0.66[Li0.22Ti0.78]O2 is ca. 110 mAh/g, and the average sodium storage voltage is ca. 0.75 V. The volume change during sodium insertion and extraction was only 0.77%, indicative of “zero-strain” characteristics; the material exhibits over 1,200 cycles with a capacity retention of 75%. The measured Na+ ion diffusion coefficient is ca. 1 × 10−10 cm2/s. It is also interesting to find out that the final discharge product is a mixture of several phases that retained the P2-structure, as confirmed by in-situ and ex-situ X-ray diffraction (XRD). The differences in these P2-phases lie in the sodium compositions and occupations (2b, 2d). These results demonstrate that P2-layered oxides are promising anode materials for long-life rechargeable sodium-ion batteries. In addition, P2-layered Na0.6Cr0.6Ti0.4O2 (Wang et al., 2015c), P2-Na0.66Co0.33Ti0.67O2 (Guo et al., 2015b), Na0.67Ni0.33Ti0.67O2 (Shanmugam and Lai, 2014), and Na2/3V2/3Ti1/3O2 (Fielden et al., 2017) showed similar sodium storage voltage around 0.75 V and capacity ca. 100 mAh/g with low volume change during sodium intercalation/extraction.
In some P2 layered oxides, such as Na0.6Cr0.6Ti0.4O2, Na0.67Ni0.33Ti0.67O2 and Na2/3V2/3Ti1/3O2, the valence states of Cr/Ni/V are 3+/2+/3+, which can be oxidized to 4+/3+/4+ on Na de-intercalation, enabling them as possible positive electrodes. Additionally, the valence state of Ti in these compounds is 4+; it can be reduced to 3+ on Na intercalation, making it a possible negative electrode. On the basis of these results, a sodium-ion full cell has been demonstrated using the same material as both negative and positive electrodes, for example, P2-Na0.6[Cr0.6Ti0.4]O2. The greatest advantage of using the same materials as both negative and positive electrodes is reducing the process cost of electrodes significantly. The symmetric full cell using P2-Na0.6[Cr0.6Ti0.4]O2 delivers an average operating voltage plateau at ~2.53 V. The energy densities of this system are calculated to be 82 and 94 Wh/kg at current rates of 1C and C/5 based on the mass of positive and negative electrodes.
O3-Layered Oxides
In 1983, NaTiO2 was first investigated as an electrode for sodium ion batteries by Maazaz et al. (1983). NaTiO2 (shown in Figure 2G) adopts the α-NaFeO2, structure which is an ordered variant of the rock-salt structure. The layers of edge-sharing TiO6 octahedra and edge-sharing NaO6 octahedra alternate along the rock-salt [111] direction (i.e., there are alternating Na-O and Ti-O slabs) (Clarke et al., 1998). They evaluated its potential as a Na+ insertion/extraction. This material delivers ~75 mAh/g, corresponding to 0.3 Na+, during multiple phases' transformation from O3 to O'3. It has an average sodium storage voltage of 1 V, while used as an anode in SIB. In 2014, Ceder et al. re-investigated O3-NaTiO2 as an anode material for sodium-ion batteries (Wu et al., 2015a). Approximately 0.5 Na is reversibly intercalated in NaTiO2, corresponding to a reversible capacity of 152 mAh/g. Special Na+/vacancy ordering was observed and accompanied with an unusual lattice parameter variation, yielding a constant inter-slab distance and slight change in the in-plane Ti–Ti distance in the O'3 phase. The O3-type Na0.8Ni0.4Ti0.6O2 was investigated as an anode by Zhou and co-workers (Guo et al., 2015a). The reversible capacity of Na0.8Ni0.4Ti0.6O2 is 107 mAh/g with a distinct step around 0.7 V. Na0.8Ni0.4Ti0.6O2-based symmetric cell has a voltage of 2.8 V, a reversible discharge capacity of 85 mAh/g, 75% capacity retention after 150 cycles. In our opinion, the maximum sodium content in Na0.8Ni0.4Ti0.6O2 is 1, so insertion and extraction of 0.2 mol Na+ corresponds to 52 mAh/g, and the extra capacities are attributed to the participation of conductive additives, such as carbon. Recently, the Hu Group reported an O3-type oxide with low sodium content, Na0.66Mg0.34Ti0.66O2, as an anode for sodium-ion batteries (Zhao et al., 2018a). This material delivers a capacity of about 98 mAh/g in a voltage range of 0.4–2.0 V and exhibits a better cycling stability (ca. 94.2 % of capacity retention after 128 cycles). In-situ XRD reveals a single-phase reaction in the discharge–charge process, which is different from the common phase transitions reported in O3-type electrodes; this single-phase mechanism ensures long-term cycling stability and high rate capacity.
Monoclinic Na2TixO2x+1
Na2Ti3O7
In 2011, Palacin et al. reported that Na2Ti3O7 consisting of zigzag-type layers are formed by stacking TiO6 octahedra ribbon, the Na+ cations locate between the TiO6 ribbons. Na2Ti3O7 takes up 2 Na+ in a formula unit at a low storage voltage of 0.3 V vs. Na+/Na (Senguttuvan et al., 2011). The structure and electrochemical performance was shown in Figure 2D. However, the initial coulombic efficiency was rather low and only 10 cycles were shown. Hu et al. found that the sodium storage behavior was affected by the particle size of Na2Ti3O7 (Pan et al., 2013b). A nano-sized Na2Ti3O7 anode exhibited higher storage capacity than a micro-sized one. After optimization of the electrolyte and binder, the Na2Ti3O7 electrode exhibited a reversible capacity of 188 mAh/g in 1 M NaFSI/PC electrolyte and sodium alginate as a binder at a current rate of 0.1C in a voltage range of 0.0–3.0 V. Unfortunately, the cycling properties are not satisfactory. In order to improve cycle life, Na2Ti3O7@MWCNTs (Wei et al., 2014), Na2Ti3O7/C (Ding et al., 2017), Na2Ti3O7@N-Doped Carbon Hollow Spheres (Xie et al., 2017), Na2Ti3O7/Titanium Peroxide (Zhao et al., 2015) and F-Doping Na2Ti3O7 (Chen et al., 2018) were investigated. However, their performances were not satisfactory due to the low electronic conductivities and structural instability which needs to be further improved. In addition, full sodium-ion batteries have been tested based on advantageous electrochemical features of nanostructured Na2Ti3O7. –for example, by using VOPO4 material as a cathode, the full cell showed the operating voltages close to 2.9 V and delivered a reversible capacity of 114 mAh/g at a rate of 0.1C. It also shows outstanding rate capability (~74 mA h/g at 2C rate) and excellent cycling stability (92.4% capacity retention after 100 cycles) (Li et al., 2016a).
Na2Ti6O13
Na2Ti6O13 crystallizes in a monoclinic crystalline structure with continuous tunnel channels along the c axis, offering space to accommodate the alkali metals (Dominko et al., 2006). In the crystal structure of Na2Ti6O13, all of the oxygen atoms belong to at least two octahedra. Transformation of the crystal structure of Na2Ti3O7 to that of Na2Ti6O13 is described as a condensation of the two-dimensional layers of octahedra to a three-dimensional structure by sharing these one-coordinated oxygen atoms. It means that there is no terminal oxygen atom in the crystal structure of Na2Ti6O13; all oxygen atoms are linearly coordinated by two titanium atoms. The TiO6 octahedra in Na2Ti6O13 are more regular, there are no very long or very short Ti-O distances. In 2011, T. Brousse et al. investigated Na2Ti6O13 as the negative electrode for sodium-ion batteries (Trinh et al., 2012). The electrode showed a 0.8 V plateau vs. Na/Na+, with an initial discharge capacity and initial columbic efficiency of only 22 mAh/g and 27%, respectively, Shen et al. subsequently performed DFT simulation and showed that a maximum of 4 Na+ are inserted into this structure (Shen and Wagemaker, 2014). They obtained 196 mAh/g by lowering the cut-off voltage from 0.3 to 0 V.
Na2Ti4O9
Kataoka et al. reported a tunnel-type Na2Ti4O9 as a negative electrode for sodium-ion batteries (Kataoka and Akimoto, 2016). The crystal structure of Na2Ti4O9 shows all of the TiO6 octahedra are strongly distorted. The remarkably defective occupations for all of three sodium sites in the Na2Ti4O9 sample; 72% for Na1, 69% for Na2 and 58% for Na3 sites, should be noted. The electrochemical measurements of Na2Ti4O9 showed the reversible sodium insertion and extraction reactions at 1.1 V, 1.5 V, and 1.8 V vs. Na/Na+. The reversible capacity for the sodium cell was 100 mAh/g at a current density of 12 mA/g, which progressively faded to 45 mAh/g after 60 cycles.
ATiOPO4 (A = NH4, K, Na)
KTiOPO4 is a well-known excellent non-linear optical material. Its crystal structure contains polytitanate chains interconnected by PO4 tetrahedral units and belongs to the Pna21 space group; K+ ions occupy two different sites, K1 and K2. The K1 site is located near the center of a passage and the K2 site is near the point of intersection of TiO6 and PO4, as shown in Figure 2H. The preparation of NaTiOPO4 by the traditional solid-state reaction is difficult. Fortunately, it was found that NaTiOPO4 is easily synthesized using a hydrothermal method and KTiOPO4 can be prepared by ion-exchange of K+ with and Na+ due to the difference in their ionic radii. These materials were investigated by electrochemical discharge/charge, with average charge voltages of 1.45 V (NH4TiOPO4), 1.4 V (KTiOPO4), and 1.5 V (NaTiOPO4), and capacities of 100 mAh/g (NH4TiOPO4), 60 mAh/g (KTiOPO4), 80 mAh/g (NaTiOPO4), respectively (Mu et al., 2016). In-situ XRD was performed to understand the reaction, and the results revealed a bi-phase reaction mechanism in NaTiOPO4 during sodium intercalation and de-intercalation.
NASICON Compounds
The crystal structure of NASICON compounds was determined in 1968. In general, the NASICON-type compounds (NaxM1M2(XO4)3 (M = V, Ti, Fe, Tr or Nb etc.; X = P, or S, x = 0–4) exhibit an open three-dimensional structure with two types of interstitial positions (M1, M2), where the conductor cations are distributed. The matrix can be broken down into fundamental groups of 2MO6 octahedra separated by 3 XO4 tetrahedra that share common corner oxygen's. As there are no shared edges or shared faces in the matrix, all of the large sodium sites are connected. The NASICON-type NaTi2(PO4)3 (shown in Figure 2I) exhibits a reversible Na storage capacity of ca.120 mAh/g at ca. 2.1 V vs. Na+/Na via Ti4+/Ti3+ conversion (Park et al., 2011). The storage voltage is high for organic sodium-ion batteries, but suitable for aqueous ion batteries. Reported examples of NASICON compounds include Na0.44MnO2/NaTi2(PO4)3 (Kim et al., 2013), Na2CuFe(CN)6/NaTi2(PO4)3 (Wessells et al., 2011a), Na2NiFe(CN)6/ NaTi2(PO4)3 (Wessells et al., 2011b), Na3V2(PO4)3/ NaTi2(PO4)3 (Song et al., 2014).
The discussion above demonstrates that the sodium storage voltages of Ti- containing anodes depend on their crystal structures, especially the local environments of Ti ions, although the redox reaction always involving Ti4+ and Ti3+. Their structures, voltages and electrochemical performances are summarized in Figure 3A in the order of voltage increase.
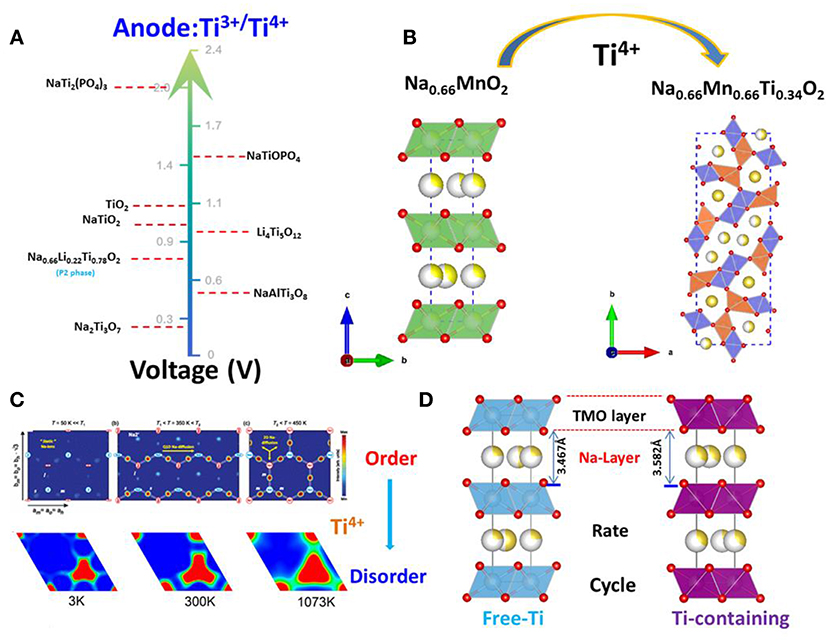
Figure 3. Roles of Ti in the electrodes. (A) The voltage of Ti3+/Ti4+ in the different structure as anode for sodium-ion batteries; (B) Ti-doping resulting in the structure change from the P2-layered to tunnel-type. (C) Upper: For the three temperature regions, there is a clear evolution from static Na+ ions to quasi-1D ion diffusion along a axis to finally fully 2D ion diffusion; With permission from APS. Lower: The mean square displacements (MSDs) of Na+ ions in P2-Na0.6[Cr0.6Ti0.4]O2 are displayed at 1,200, 1,500, 1,800, 2,400, and 2,700 K; With permission from Springer. (D) The schematic diagram of Ti-substitution increases the crystal lattice of P2-layered oxides.
The average Na+ ion insertion potentials of electrode materials can also be estimated by Density Function Theory calculation which plays an important role in new material design. The equilibrium intercalation voltage depends on the chemical potential difference of sodium in the electrode materials and can be approximated by equation (1) without considering the small contributions of entropy and volume changes to the cell voltage (Aydinol and Ceder, 1997; Meng and Arroyo-De Dompablo, 2009).
where x1 and x2 are the Na+ contents in the pristine and final electrodes; F is the Faraday constant; z is electronic charge of sodium ions, i.e., 1; Etotal is the total energy per formula unit which is a function of the crystal structure. In the above mentioned Ti containing compounds, despite all Ti ions situated at the center of TiO6 octahedra, the differences in the connections between TiO6 octahedra as well as between TiO6 and other components constitute the different environments of Ti in various structures, as a consequence, the total energies are different, and therefore have different average cell voltages. For example, Na0.66[Li0.22Ti0.78]O2 (0.47 V), Li4Ti5O12 (0.91 V), Na2Ti3O7 (0.3 V) (Rousse et al., 2013). In general, Ti-containing anodes have low electronic conductivity. Among the six structures mentioned in this section, layered oxides showed good cycle life due to their single-phase reaction mechanism and low volume change, but their capacities must increase from the present value of ca. 100 mAh/g. Therefore, future work should focus on increasing the capacities (200–300 mAh/g) and electronic conductivities of the “zero strain” layered oxides with storage voltages at ca. 1 V.
Function of Ti in the Cathode
In contrast to the anode, the cathode materials include the redox couples Ni2+/Ni3+/Ni4+, Mn2+/Mn3+/Mn4+, Co3+/Co4+, V3+/V4+, Cu2+/Cu3+, Cr3+/Cr4+, etc. Although Ti4+-dopant does not participate in charge transfer involving Na+ extraction and insertion, it affects the crystal structure, including cation-ordering and lattice structure.
Structure Change
In the case of P2-type materials, the value of “x” in NaxMO2 is typically 2/3, leaving many vacancies in the alkali layer. NaxMnO2 (0.6 < × < 0.72) is a typical P2-layered oxide. Caballero reported a layered sodium manganese oxide (Na0.6MnO2) synthesized by a sol–gel method (Caballero et al., 2002). This material delivered a constant specific capacity of 140 mAh/g at 0.1 mA/cm2 in a voltage window of 2.0–3.8V. However, the continuous strains and distortions resulting from insertion and extraction of Na+ caused the host structure to gradually collapse and yielded an amorphous material after eight cycles. This led to a progressive reduction of the cell capacity, regardless of the voltage window used. In 2014, DFT calculations by Ceder et al. revealed that Na+ ordering in NaxMnO2 was controlled by the underlying combination of electrostatic and electronic structure interactions via Jahn–Teller effect, enabling some Na to occupy the highly distorted octahedral site. This led to Na and Mn charge-ordered stripes, which yielded a fascinating low-temperature magnetic ordering (Li et al., 2014). In 2015, Wang et al. reported a tunnel-type structure, Nax[MnxTi1−x]O2 (x = 0.44, 0.50, 0.55, 0.60, 0.63, 0.66), which has a large S-shaped tunnel and a small hexagonal tunnel formed by MnO6 and MnO5 polyhedrals (Wang et al., 2015b). The structure of these materials was similar to tunnel-type Na0.44MnO2 (S.G.: Pbam) (as shown in Figure 3B) with sodium ions in the S-shaped and hexagonal tunnels. Unlike layered NaxMnO2, tunnel-type Nax[MnxTi1−x]O2 is stable in an aqueous solution. Hence, Na0.66[Mn0.66Ti0.34]O2 can be used as a positive electrode material for aqueous sodium-ion batteries. In particular, it showed the highest reversible capacity (76 mAh/g) at a current rate of 2C among all the oxide electrode materials, with an average operating voltage of 1.2 V when coupled with a NaTi2(PO4)3/C negative electrode. The Na0.66[Mn0.66Ti0.34]O2|NaTi2(PO4)3/C aqueous sodium-ion cell demonstrates excellent cycle performance with minimal capacity decay after 300 cycles.
The O3-type NaFeO2 attracted a lot of attention due to its inexpensive and non-toxic features, and is expected to have a reversible Fe4+/Fe3+ conversion (Yabuuchi et al., 2012). Recent studies showed a reversible Na storage in NaFeO2 at 3.3 V with a small polarization by limiting the cut-off voltage. In contrast, the Li analog, O3-LiFeO2 (Kanno et al., 1996), is nearly electrochemically inactive due to a low energy barrier for Fe4+ migrating to the alkali layer. The charge capacity in the half cell was ascribed to the dominant reaction of oxygen removal at the solid/electrolyte interface rather than Fe4+/Fe3+ conversion as the Fe3+ 3d-orbital is strongly hybridized with the oxygen 2p orbital in the Li system; oxygen removal is more favorable energetically than the oxidation to Fe4+. Substitution of Ti for Fe in NaFeO2 changes the structure from the O3-phase to a tunnel type. For example, the tunnel-type Na0.875Fe0.875Ti1.125O4 delivered a capacity of 45 mAh/g corresponding to the extraction/insertion of 0.37 Na+ ions. Compared to NaFeO2, Na0.875Fe0.875Ti1.125O4 exhibits the low capacities due to the lower quantity of sodium participates in the redox reaction, but the sodium storage voltage improves from 3.2 to 3.6 V (Pan et al., 2013a; Bhange et al., 2017).
Cation-Disorder
NaxCoO2 is known as the first layered oxide investigated as a Na intercalation host (Delmas et al., 1981). Depending on the sodium content and temperature of this compound, an interplay between Na+-Na+ and Na+-Co3+/4+ electrostatic interactions coupled with Co3+/4+ charge ordering results in the formation of numerous Na+/vacancy-ordered superstructures (see the Figure 3C; Medarde et al., 2013). These ordered superstructures have distinct voltage plateaus, lowering the Na+ ion diffusion coefficient and even reducing the dimensionality of ionic transport. The combination of these characteristics, in turn, result in rapid capacity-fading during cycling, as in the case of NaxCoO2 (Berthelot et al., 2011), NaxMnO2 (Jeong and Manthiram, 2001), NaxVO2 (Guignard et al., 2013), Na2/3Ni1/3Mn2/3O2 (Lu and Dahn, 2001), and Na2/3Co2/3Mn1/3O2 (Carlier et al., 2011). In 2015, Wang et al. reported a critical difference in ionic radii between M1 and M2 is ~15% in layered oxides P2-NaM1M2O2 (M1, M2 are transitional metals) which governs cation order-disorder transition (Wang et al., 2015c). If the radius difference is higher than 15% and M1/M2 content is close to a rational ratio, then an ordered structure is expected; otherwise M1 and M2 is disordered. The presence of charge ordering is determined by the redox potentials (that is, Fermi level of M1 and M2). Small differences are favorable for charge ordering, even if M1 and M2 are chemically disordered. Finally, Na+/vacancy ordering and charge ordering are coupled to each other. There would be no charge ordering without Na+/vacancy ordering and vice versa. The ratio of ionic radii of Ti4+/Ni2+, Ti4+/Co2+, Ti4+/Cr3+, Ti4+/Mn3+ are 1.14, 1.04, 1.02, 1.14, respectively, which are <1.15. This means that the transition layered oxides are all disordered. In addition, there are large differences in redox potentials (or Fermi level) of Ti4+/Ni2+, Ti4+/Co2+, Ti4+/Cr3+, Ti4+/Mn3+ which leads to charge disordering, and hence Na+/vacancy disordering. The behavior of charge/discharge profiles of Na2/3Ni1/3Ti2/3O2 (Shanmugam and Lai, 2014), Na2/3Co1/3Ti2/3O2 (Guo et al., 2015b) and Na0.6Cr0.6Ti0.4O2 exhibit a smooth slope without the plateau that is attributed to the Na+/vacancy ordering. Wang et al. analyzed neutron powder diffraction data collected from Na0.6[Cr0.6Ti0.4]O2 on cooling to 3 K; the results showed no evidence of Cr/Ti and Na+/vacancy orderings, and charge and Na+/vacancy orderings were also not observed in the tunnel-type Ti-substituted Na0.44MnO2 In contrast, Na+/vacancy ordering was found in Na0.7CoO2 which exhibits the different transport channels at the different temperatures (see in Figure 3C).
Distance Between Layers
A larger crystal lattice enhances ionic transport of Na+, thus improving rate and cycle performance (As shown in Figure 3D). Normally, Ti-doping will lead to an increase in the alkali-layer distance of 1–3%, such as in NaCrO2 (Li et al., 2019), Na2/3CoO2 (Sabi et al., 2017) and Na0.67Fe0.5Mn0.5O2 (Park et al., 2018). Recently, Lee et al. reported enhanced rate capability and cycle performance by substitution of Ti for Fe in P2-Type Na0.67Fe0.5Mn0.5O2 cathode for sodium-ion batteries (Park et al., 2018). They found 5% Ti substitution increases the crystal lattice from 3.528 to 3.571 Å. This small change promotes Na-ion diffusion and prevents the phase transition from the P2 to the OP4/“Z” structure. In addition, Du's group reported that with 5% Ti-substitution in NaCrO2, the crystal lattice expanded 1%, which resulted in an increase of 25% in capacity retention at the current rate of 30C and enhancement in cycle life (Li et al., 2019).
Summary
The role of Ti in the cathode and anode for sodium-ion batteries are reviewed in the present work. In the anode, the redox couple, Ti4+/Ti3+, exhibits different storage voltages and imparted properties that are beneficial in designing different materials for various applications, such as NaTi2(PO4)3 for aqueous batteries, P2-Na0.66Li0.22Ti0.78O2 for long cycle batteries, etc. The substitution of Ti disrupts the structure ordering and expands the crystal lattice which prolongs the cycle life and enhances rate performance. In addition, Ti-substituted Na2/3MnO2 prevents H3O+ intercalation, making it a perfect cathode material for aqueous sodium-ion batteries. Developing practical cathode and anode materials for sodium-ion batteries is a big challenge. In spite of the advances discussed in this review, there is still substantial room for further improvement.
Although the above-mentioned challenges still remain, the recent progress in the development of Ti-compounds is significant. There is now an improved understanding of the effects of Ti addition on the crystal structure, including the lattice expansion and distortion, the cation and vacancy orderings, as well as on the electronic configurations of the redox couples. This knowledge helps researchers to identify other dopants and materials for designing and developing new high-performance electrode materials. We believe that further studies will lead to more exciting results. This review highlights the importance of designing and exploring disordered transition metal/charge in electrodes as an effective strategy to improve Na-storage properties, including rate capacity, cycle stability and high-power performance.
Author Contributions
YW wrote the draft. All authors contributed to the writing, discussion, and revision of the final version.
Funding
Centre d'excellence en électrification des transports et stockage d'énergie, Québec, Canada.
Conflict of Interest Statement
YW, WZ, AG, CK, and KZ are employed by Hydro Quebec.
References
Alcántara, R., Jiménez-Mateos, J. M., Lavela, P., and Tirado, J. L. (2001). Carbon black: a promising electrode material for sodium-ion batteries. Electrochem. Commun. 3, 639–642. doi: 10.1016/S1388-2481(01)00244-2
Armand, M., and Tarascon, J. M. (2008). Building better batteries. Nature 451, 652–657. doi: 10.1038/451652a
Aydinol, M. K., and Ceder, G. (1997). First-principles prediction of insertion potentials in Li-Mn oxides for secondary Li batteries. J. Electrochem. Soc. 144, 3832–3835. doi: 10.1149/1.1838099
Berthelot, R., Carlier, D., and Delmas, C. (2011). Electrochemical investigation of the P2-NaxCoO2 phase diagram. Nat. Mater. 10, 74–U73. doi: 10.1038/nmat2920
Bhange, D. S., Ali, G., Kim, J-Y., Chung, K. Y., and Nam, K-W. (2017). Improving the sodium storage capacity of tunnel structured NaxFexTi2−xO4 (x = 1, 0.9 & 0.8) anode materials by tuning sodium deficiency. J. Power Sources 366, 115–122. doi: 10.1016/j.jpowsour.2017.08.112
Braconnier, J. J., Delmas, C., and Hagenmuller, P. (1982). Etude par desintercalation electrochimique des systemes NaxCrO2 et NaxNiO2. Mater. Res. Bull. 17, 993–1000. doi: 10.1016/0025-5408(82)90124-6
Caballero, A., Hernan, L., Morales, J., Sanchez, L., Pena, J. S., and Aranda, M., (2002). Synthesis and characterization of high-temperature hexagonal P2-Na0.6MnO2 and its electrochemical behaviour as cathode in sodium cells. J. Mater. Chem. 12, 1142–1147. doi: 10.1039/b108830k
Carlier, D., Cheng, J. H., Berthelot, R., Guignard, M., Yoncheva, M., Stoyanova, R., et al. (2011). The P2-Na2/3Co2/3Mn1/3O2 phase: structure, physical properties and electrochemical behavior as positive electrode in sodium battery. Dalton Transact. 40, 9306–9312. doi: 10.1039/c1dt10798d
Chen, C., Vaughey, J., Jansen, A., Dees, D., Kahaian, A., Goacher, T., et al. (2001). Studies of Mg-substituted Li4−xMgxTi5O12 spinel electrodes (0 ≤ x ≤ 1) for lithium batteries. J. Electrochem. Soc. 148, A102–A104. doi: 10.1149/1.1344523
Chen, J., Song, W., Hou, H., Zhang, Y., Jing, M., Jia, X., et al. (2015). Ti3+ Self-doped dark rutile TiO2 ultrafine nanorods with durable high-rate capability for lithium-ion batteries. Adv. Funct. Mater. 25, 6793–6801. doi: 10.1002/adfm.201502978
Chen, Z., Lu, L., Gao, Y., Zhang, Q., Zhang, C., Sun, C., et al. (2018). Effects of F-doping on the electrochemical performance of Na2Ti3O7 as an anode for sodium-ion batteries. Materials 11:2206. doi: 10.3390/ma11112206
Chevrier, V. L., and Ceder, G. (2011). Challenges for Na-ion negative electrodes. J. Electrochem. Soc. 158, A1011–A1014. doi: 10.1149/1.3607983
Clarke, S. J., Fowkes, A. J., Harrison, A., Ibberson, R. M., and Rosseinsky, M. J. (1998). Synthesis, structure, and magnetic properties of NaTiO2. Chem. Mater. 10, 372–384. doi: 10.1021/cm970538c
Darwiche, A., Marino, C., Sougrati, M. T., Fraisse, B., Stievano, L., and Monconduit, L. (2012). Better cycling performances of bulk Sb in Na-ion batteries compared to Li-ion systems: an unexpected electrochemical mechanism. J. Am. Chem. Soc. 134, 20805–20811. doi: 10.1021/ja310347x
Delmas, C., Braconnier, J-J., Fouassier, C., and Hagenmuller, P. (1981). Electrochemical intercalation of sodium in NaxCoO2 bronzes. solid state ionics 3–4, 165–169. doi: 10.1016/0167-2738(81)90076-X
Delmas, C., Fouassier, C., and Hagenmuller, P. (1980). Structural classification and properties of the layered oxides. Physica B C 99, 81–85. doi: 10.1016/0378-4363(80)90214-4
Ding, C., Nohira, T., and Hagiwara, R. (2017). Electrochemical performance of Na2Ti3O7/C negative electrode in ionic liquid electrolyte for sodium secondary batteries. J. Power Sources 354, 10–15. doi: 10.1016/j.jpowsour.2017.04.027
Doeff, M. M., Peng, M. Y., Ma, Y. P., and Dejonghe, L. C. (1994). Orthorhombic NaxMnO2 as a cathode material for secondary sodium and lithium polymer batteries. J. Electrochem. Soc. 141, L145–L147. doi: 10.1149/1.2059323
Dominko, R., Baudrin, E., Umek, P., Arčon, D., Gaberšček, M., and Jamnik, J. (2006). Reversible lithium insertion into Na2Ti6O13 structure. Electrochem. Commun. 8, 673–677. doi: 10.1016/j.elecom.2006.02.017
Dunn, B., Kamath, H., and Tarascon, J-M. (2011). Electrical energy storage for the grid: a battery of choices. Science 334, 928–935. doi: 10.1126/science.1212741
Ellis, B. L., and Nazar, L. F. (2012). Sodium and sodium-ion energy storage batteries. Curr. Opin. Solid State Mater. Sci. 16, 168–177. doi: 10.1016/j.cossms.2012.04.002
Farbod, B., Cui, K., Kalisvaart, W. P., Kupsta, M., Zahiri, B., Kohandehghan, A., et al. (2014). Anodes for sodium ion batteries based on tin-germanium-antimony alloys. Acs Nano 8, 4415–4429. doi: 10.1021/nn4063598
Ferg, E., Gummow, R., De Kock, A., and Thackeray, M. (1994). Spinel anodes for lithium-ion batteries. J. Electrochem. Soc. 141, L147–L150. doi: 10.1149/1.2059324
Fielden, R., Cole, L., and Obrovac, M. N. (2017). Low voltage sodium intercalation in NaxVxTi1−xO2 (2/3 ≤ x ≤ 1). J. Electrochem. Soc. 164, A490–A497. doi: 10.1149/2.1361702jes
González, J. R., Alcántara, R., Nacimiento, F., Ortiz, G. F., and Tirado, J. L. (2014). Microstructure of the epitaxial film of anatase nanotubes obtained at high voltage and the mechanism of its electrochemical reaction with sodium. CrystEngComm 16, 4602–4609. doi: 10.1039/C4CE00272E
Goodenough, J. B., and Kim, Y. (2009). Challenges for rechargeable Li batteries. Chem. Mater. 22, 587–603. doi: 10.1021/cm901452z
Gover, R. K. B., Bryan, A., Burns, P., and Barker, J. (2006). The electrochemical insertion properties of sodium vanadium fluorophosphate, Na3V2(PO4)2F3. Solid State Ionics 177, 1495–1500. doi: 10.1016/j.ssi.2006.07.028
Guignard, M., Didier, C., Darriet, J., Bordet, P., Elkaïm, E., and Delmas, C. (2013). P2-NaxVO2 system as electrodes for batteries and electron-correlated materials. Nat. Mater. 12, 74–80. doi: 10.1038/nmat3478
Guo, S., Yu, H., Liu, P., Ren, Y., Zhang, T., Chen, M., et al. (2015a). High-performance symmetric sodium-ion batteries using a new, bipolar O3-type material, Na0.8Ni0.4Ti0.6O2. Energy Environ. Sci. 8, 1237–1244. doi: 10.1039/C4EE03361B
Guo, S. S., Liu, P., Sun, Y., Zhu, K., Yi, J., Chen, M. W., et al. (2015b). A high-voltage and ultralong-life sodium full cell for stationary energy storage. Angew. Chem. Int. Ed. 54, 11701–11705. doi: 10.1002/anie.201505215
He, H., Gan, Q., Wang, H., Xu, G-L., Zhang, X., Huang, D., et al. (2018). Structure-dependent performance of TiO2/C as anode material for Na-ion batteries. Nano Energy 44, 217–227. doi: 10.1016/j.nanoen.2017.11.077
He, H., Sun, D., Zhang, Q., Fu, F., Tang, Y., Guo, J., et al. (2017). Iron-doped cauliflower-like rutile TiO2 with superior sodium storage properties. ACS Appl. Mater. Interfaces 9, 6093–6103. doi: 10.1021/acsami.6b15516
Hosono, E., Saito, T., Hoshino, J., Okubo, M., Saito, Y., Nishio-Hamane, D., et al. (2012). High power Na-ion rechargeable battery with single-crystalline Na0.44MnO2 nanowire electrode. J. Power Sources 217, 43–46. doi: 10.1016/j.jpowsour.2012.05.100
Hu, Z., Wang, L., Zhang, K., Wang, J., Cheng, F., Tao, Z., et al. (2014). MoS2 nanoflowers with expanded interlayers as high-performance anodes for sodium-ion batteries. Angew. Chem. Int. Ed. 53, 12794–12798. doi: 10.1002/anie.201407898
Jeong, Y. U., and Manthiram, A. (2001). Synthesis of NaxMnO2+δ by a reduction of aqueous sodium permanganate with sodium iodide. J. Solid State Chem. 156, 331–338. doi: 10.1006/jssc.2000.9003
Jian, Z., Zhao, B., Liu, P., Li, F., Zheng, M., Chen, M., et al. (2014). Fe2O3 nanocrystals anchored onto graphene nanosheets as the anode material for low-cost sodium-ion batteries. Chem. Commun. 50, 1215–1217. doi: 10.1039/C3CC47977C
Jian, Z., Zhao, L., Pan, H., Hu, Y-S., Li, H., Chen, W., et al. (2012). Carbon coated Na3V2(PO4)3 as novel electrode material for sodium ion batteries. Electrochem. Commun. 14, 86–89. doi: 10.1016/j.elecom.2011.11.009
Kanno, R., Shirane, T., Kawamoto, Y., Takeda, Y., Takano, M., Ohashi, M., et al. (1996). Synthesis, structure, and electrochemical properties of a new lithium iron oxide, LiFeO2, with a corrugated layer structure. J. Electrochem. Soc. 143, 2435–2442. doi: 10.1149/1.1837027
Kataoka, K., and Akimoto, J. (2016). Synthesis and electrochemical sodium and lithium insertion properties of sodium titanium oxide with the tunnel type structure. J. Power Sources 305, 151–155. doi: 10.1016/j.jpowsour.2015.11.090
Kim, D. J., Ponraj, R., Kannan, A. G., Lee, H-W., Fathi, R., Ruffo, R., et al. (2013). Diffusion behavior of sodium ions in Na0.44MnO2 in aqueous and non-aqueous electrolytes. J. Power Sources 244, 758–763. doi: 10.1016/j.jpowsour.2013.02.090
Kim, J., Seo, D-H., Kim, H., Park, I., Yoo, J-K., Jung, S-K., et al. (2015). Unexpected discovery of low-cost maricite NaFePO4 as a high-performance electrode for Na-ion batteries. Energy Environ. Sci. 8, 540–545. doi: 10.1039/C4EE03215B
Kim, K-T., Ali, G., Chung, K. Y., Yoon, C. S., Yashiro, H., Sun, Y-K., et al. (2014). Anatase titania nanorods as an intercalation anode material for rechargeable sodium batteries. Nano Lett. 14, 416–422. doi: 10.1021/nl402747x
Lan, T., Wang, T., Zhang, W., Wu, N-L., and Wei, M. (2017). Rutile TiO2 mesocrystals with tunable subunits as a long-term cycling performance anode for sodium-ion batteries. J. Alloys Compd. 699, 455–462. doi: 10.1016/j.jallcom.2016.12.337
Li, H., Peng, L., Zhu, Y., Chen, D., Zhang, X., and Yu, G. (2016a). An advanced high-energy sodium ion full battery based on nanostructured Na2Ti3O7/VOPO4 layered materials. Energy Environ. Sci. 9, 3399–3405. doi: 10.1039/C6EE00794E
Li, J., Liu, J., Sun, Q., Banis, M. N., Sun, X., and Sham, T-K. (2017). Tracking the effect of sodium insertion/extraction in amorphous and anatase TiO2 nanotubes. J. Phys. Chem. C 121, 11773–11782. doi: 10.1021/acs.jpcc.7b01106
Li, W., Wang, Y., Hu, G., Peng, Z., Cao, Y., Zeng, Y., et al. (2019). Ti-doped NaCrO2 as cathode materials for sodium-ion batteries with excellent long cycle life. J. Alloys Compd. 779, 147–155. doi: 10.1016/j.jallcom.2018.11.257
Li, X., Ma, X., Su, D., Liu, L., Chisnell, R., Ong, S. P., et al. (2014). Direct visualization of the jahn–teller effect coupled to Na ordering in Na5/8MnO2. Nat. Mater. 13:586. doi: 10.1038/nmat3964
Li, Y., Hu, Y-S., Li, H., Chen, L., and Huang, X. (2016b). A superior low-cost amorphous carbon anode made from pitch and lignin for sodium-ion batteries. J Mater. Chem. A 4, 96–104. doi: 10.1039/C5TA08601A
Li, Y., Xu, S., Wu, X., Yu, J., Wang, Y., Hu, Y-S., et al. (2015). Amorphous monodispersed hard carbon micro-spherules derived from biomass as a high performance negative electrode material for sodium-ion batteries. J. Mater. Chem. A 3, 71–77. doi: 10.1039/C4TA05451B
Li, Z., Young, D., Xiang, K., Carter, W. C., and Chiang, Y-M. (2013). Towards high power high energy aqueous sodium-ion batteries: the NaTi2(PO4)3/Na0.44MnO2 system. Adv. Energy Mater. 3, 290–294. doi: 10.1002/aenm.201200598
Lim, S. Y., Lee, J. H., Kim, S., Shin, J., Choi, W., Chung, K. Y., et al. (2017). Lattice water for the enhanced performance of amorphous iron phosphate in sodium-ion batteries. ACS Energy Lett. 2, 998–1004. doi: 10.1021/acsenergylett.7b00120
Liu, Y., Wang, H., Cheng, L., Han, N., Zhao, F., Li, P., et al. (2016). TiS2 nanoplates: a high-rate and stable electrode material for sodium ion batteries. Nano Energy 20, 168–175. doi: 10.1016/j.nanoen.2015.12.028
Lu, Y., Zhao, C., Qi, X., Qi, Y., Li, H., Huang, X., et al. (2018). Pre-oxidation-tuned microstructures of carbon anodes derived from pitch for enhancing Na storage performance. Adv. Energy Mater. 8:1800108. doi: 10.1002/aenm.201800108
Lu, Z., and Dahn, J. R. (2001). In situ x-ray diffraction study of P 2 - Na2/3 [Ni1/3Mn2/3]O2. J. Electrochem. Soc. 148, A1225–A1229. doi: 10.1149/1.1407247
Maazaz, A., Delmas, C., and Hagenmuller, P. (1983). A study of the NaxTiO2 system by electrochemical deintercalation. J. Inclus. Phenom. 1, 45–51. doi: 10.1007/BF00658014
Medarde, M., Mena, M., Gavilano, J. L., Pomjakushina, E., Sugiyama, J., Kamazawa, K., et al. (2013). 1D to 2D Na+ion diffusion inherently linked to structural transitions in Na0.7CoO2. Phys. Rev. Lett. 110:266401. doi: 10.1103/PhysRevLett.110.266401
Meng, Y. S., and Arroyo-De Dompablo, M. E. (2009). First principles computational materials design for energy storage materials in lithium ion batteries. Energy Environ. Sci. 2, 589–609. doi: 10.1039/b901825e
Miyazaki, S., Kikkawa, S., and Koizumi, M. (1983). Chemical and electrochemical deintercalations of the layered compounds LiMO2 (M = Cr, Co) and NaMO2 (M=Cr, Fe, Co, Ni). Synth. Met. 6, 211–217. doi: 10.1016/0379-6779(83)90156-X
Mizushima, K., Jones, P. C., Wiseman, P. J., and Goodenough, J. B. (1980). LixCoO2 (0 < x < 1): a new cathode material for batteries of high energy density. Mater. Res. Bull. 15, 783–789. doi: 10.1016/0025-5408(80)90012-4
Mu, L., Ben, L., Hu, Y-S., Li, H., Chen, L., and Huang, X. (2016). Novel 1.5 V anode materials, ATiOPO4 (A = NH4, K, Na), for room-temperature sodium-ion batteries. J. Mater. Chem. A 4, 7141–7147. doi: 10.1039/C6TA00891G
Newman, G. H., and Klemann, L. P. (1980). Ambient temperature cycling of an Na - TiS2 cell. J. Electrochem. Soc. 127, 2097–2099. doi: 10.1149/1.2129353
Oh, S-M., Myung, S-T., Hassoun, J., Scrosati, B., and Sun, Y-K. (2012). Reversible NaFePO4 electrode for sodium secondary batteries. Electrochem. Commun. 22, 149–152. doi: 10.1016/j.elecom.2012.06.014
Pan, H., Hu, Y-S., and Chen, L. (2013a). Room-temperature stationary sodium-ion batteries for large-scale electric energy storage. Energy Environ. Sci. 6, 2338–2360. doi: 10.1039/c3ee40847g
Pan, H. L., Lu, X., Yu, X. Q., Hu, Y. S., Li, H., Yang, X. Q., et al. (2013b). Sodium storage and transport properties in layered Na2Ti3O7 for room-temperature sodium-ion batteries. Adv. Energy Mater. 3, 1186–1194. doi: 10.1002/aenm.201300139
Pang, G., Yuan, C., Nie, P., Ding, B., Zhu, J., and Zhang, X. (2014). Synthesis of NASICON-type structured NaTi2(PO4)3-graphene nanocomposite as an anode for aqueous rechargeable Na-ion batteries. Nanoscale 6, 6328–6334. doi: 10.1039/C3NR06730K
Park, J-K., Park, G-G., Kwak, H. H., Hong, S-T., and Lee, J-W. (2018). Enhanced rate capability and cycle performance of titanium-substituted P2-Type Na0.67Fe0.5Mn0.5O2 as a cathode for sodium-ion batteries. ACS Omega 3, 361–368. doi: 10.1021/acsomega.7b01481
Park, S. I., Gocheva, I., Okada, S., and Yamaki, J-I. (2011). Electrochemical properties of NaTi2(PO4)3 Anode for rechargeable aqueous sodium-ion batteries. J. Electrochem. Soc. 158, A1067–A1070. doi: 10.1149/1.3611434
Ramireddy, T., Xing, T., Rahman, M. M., Chen, Y., Dutercq, Q., Gunzelmann, D., et al. (2015). Phosphorus–carbon nanocomposite anodes for lithium-ion and sodium-ion batteries. J. Mater. Chem. A 3, 5572–5584. doi: 10.1039/C4TA06186A
Reddy, R. N., and Reddy, R. G. (2004). Synthesis and electrochemical characterization of amorphous MnO2 electrochemical capacitor electrode material. J. Power Sources 132, 315–320. doi: 10.1016/j.jpowsour.2003.12.054
Rousse, G., Arroyo-De Dompablo, M. E., Senguttuvan, P., Ponrouch, A., Tarascon, J-M., and Palacín, M. R. (2013). Rationalization of intercalation potential and redox mechanism for A2Ti3O7 (A = Li, Na). Chem. Mater. 25, 4946–4956. doi: 10.1021/cm4032336
Sabi, N., Sarapulova, A., Indris, S., Ehrenberg, H., Alami, J., and Saadoune, I. (2017). Effect of titanium substitution in a a P2-Na2/3Co0.95Ti0.05O2 Cathode material on the structural and electrochemical properties. ACS Appl. Mater. Interfaces 9, 37778–37785. doi: 10.1021/acsami.7b11636
Samin, A., Kurth, M., and Cao, L. (2015). Ab initio study of radiation effects on the Li4Ti5O12 electrode used in lithium-ion batteries. AIP Adv. 5:47110. doi: 10.1063/1.4917308
Senguttuvan, P., Rousse, G., Seznec, V., Tarascon, J-M., and Palacín, M. R. (2011). Na2Ti3O7: Lowest voltage ever reported oxide insertion electrode for sodium ion batteries. Chem. Mater. 23, 4109–4111. doi: 10.1021/cm202076g
Shanmugam, R., and Lai, W. (2014). Na2/3Ni1/3Ti2/3O2: “bi-functional” electrode materials for Na-ion batteries. Ecs Electrochem. Lett. 3, A23–A25. doi: 10.1149/2.007404eel
Shen, K., and Wagemaker, M. (2014). Na2+xTi6O13 as Potential negative electrode material for Na-ion batteries. Inorg. Chem. 53, 8250–8256. doi: 10.1021/ic5004269
Silbernagel, B. G., and Whittingham, M. S. (1976). An NMR study of the alkali metal intercalation phase LixTiS2: relation to structure, thermodynamics, and ionicity. J. Chem. Phys. 64, 3670–3673. doi: 10.1063/1.432731
Slater, M. D., Kim, D., Lee, E., and Johnson, C. S. (2013). Sodium-ion batteries. Adv. Funct. Mater. 23, 947–958. doi: 10.1002/adfm.201200691
Song, W., Ji, X., Zhu, Y., Zhu, H., Li, F., Chen, J., et al. (2014). Aqueous sodium-ion battery using a Na3V2(PO4)3 electrode. ChemElectroChem. 1, 871–876. doi: 10.1002/celc.201300248
Su, D., Dou, S., and Wang, G. (2015). Anatase TiO2: better anode material than amorphous and rutile phases of TiO2 for Na-ion batteries. Chem. Mater. 27, 6022–6029. doi: 10.1021/acs.chemmater.5b02348
Sun, Q., Ren, Q-Q., Li, H., and Fu, Z-W. (2011). High capacity Sb2O4 thin film electrodes for rechargeable sodium battery. Electrochem. Commun. 13, 1462–1464. doi: 10.1016/j.elecom.2011.09.020
Sun, Y., Zhao, L., Pan, H., Lu, X., Gu, L., Hu, Y-S., et al. (2013). Direct atomic-scale confirmation of three-phase storage mechanism in Li4Ti5O12 anodes for room-temperature sodium-ion batteries. Nat. Commun. 4:1870. doi: 10.1038/ncomms2878
Tao, H., Zhou, M., Wang, R., Wang, K., Cheng, S., and Jiang, K. (2018). TiS2 as an advanced conversion electrode for sodium-ion batteries with ultra-high capacity and long-cycle life. Adv. Sci. 1801021. doi: 10.1002/advs.201801021
Trinh, N. D., Crosnier, O., Schougaard, S., and Brousse, T. (2012). Synthesis, characterizations and electrochemical studies of Na2Ti6O13 for sodium ion batteries. ECS Trans. 35, 91–98. doi: 10.1149/1.3655691
Usui, H., Yoshioka, S., Wasada, K., Shimizu, M., and Sakaguchi, H. (2015). Nb-doped rutile TiO2: a potential anode material for Na-ion battery. ACS Appl. Mater. Interfaces 7, 6567–6573. doi: 10.1021/am508670z
Wagemaker, M., Van Eck, E. R., Kentgens, A. P., and Mulder, F. M. (2008). Li-ion diffusion in the equilibrium nanomorphology of spinel Li4+xTi5O12. J. Phys. Chem. B 113, 224–230. doi: 10.1021/jp8073706
Wang, S., Wang, L., Zhu, Z., Hu, Z., Zhao, Q., and Chen, J. (2014). All organic sodium-ion batteries with Na4C8H2O6. Angew. Chem. Int. Ed. 53, 5892–5896. doi: 10.1002/anie.201400032
Wang, Y., Feng, Z., Laul, D., Zhu, W., Provencher, M., Trudeau, M. L., et al. (2018a). Ultra-low cost and highly stable hydrated FePO4 anodes for aqueous sodium-ion battery. J. Power Sour. 374, 211–216. doi: 10.1016/j.jpowsour.2017.10.088
Wang, Y., Feng, Z., Zhu, W., Gariépy, V., Gagnon, C., Provencher, M., et al. (2018b). High capacity and high efficiency maple tree-biomass-derived hard carbon as an anode material for sodium-ion batteries. Materials 11:1294. doi: 10.3390/ma11081294
Wang, Y., Liu, J., Lee, B., Qiao, R., Yang, Z., Xu, S., et al. (2015a). Ti-substituted tunnel-type Na0.44MnO2 oxide as a negative electrode for aqueous sodium-ion batteries. Nat. Commun. 6:6401. doi: 10.1038/ncomms7401
Wang, Y., Mu, L., Liu, J., Yang, Z., Yu, X., Gu, L., et al. (2015b). A novel high capacity positive electrode material with tunnel-type structure for aqueous sodium-ion batteries. Adv. Energy Mater. 5:1501005. doi: 10.1002/aenm.201501005
Wang, Y., Xiao, R., Hu, Y-S., Avdeev, M., and Chen, L. (2015c). P2-Na0.6[Cr0.6Ti0.4]O2 cation-disordered electrode for high-rate symmetric rechargeable sodium-ion batteries. Nat. Commun. 6:6954. doi: 10.1038/ncomms7954
Wang, Y. S., Yu, X. Q., Xu, S. Y., Bai, J. M., Xiao, R. J., Hu, Y. S., et al. (2013). A zero-strain layered metal oxide as the negative electrode for long-life sodium-ion batteries. Nat. Commun. 4:2365. doi: 10.1038/ncomms3365
Wei, Z., Ying, W., Zongling, H., and Jingze, L. (2014). Synthesis of Na2Ti3O7@MWCNTs anode material by spray-drying method of sol-gel precursor for sodium-ion batteries. Sci. Sin. Chim. 44:1347. doi: 10.1360/N032014-00115
Wessells, C. D., Huggins, R. A., and Cui, Y. (2011a). Copper hexacyanoferrate battery electrodes with long cycle life and high power. Nat. Commun. 2:550. doi: 10.1038/ncomms1563
Wessells, C. D., Peddada, S. V., Huggins, R. A., and Cui, Y. (2011b). Nickel hexacyanoferrate nanoparticle electrodes for aqueous sodium and potassium ion batteries. Nano Lett. 11, 5421–5425. doi: 10.1021/nl203193q
Whitacre, J. F., Tevar, A., and Sharma, S. (2010). Na4Mn9O18 as a positive electrode material for an aqueous electrolyte sodium-ion energy storage device. Electrochem. Commun. 12, 463–466. doi: 10.1016/j.elecom.2010.01.020
Wu, D., Li, X., Xu, B., Twu, N., Liu, L., and Ceder, G. (2015a). NaTiO2: a layered anode material for sodium-ion batteries. Energy Environ. Sci. 8, 195–202. doi: 10.1039/C4EE03045A
Wu, L., Bresser, D., Buchholz, D., Giffin, G. A., Castro, C. R., Ochel, A., et al. (2015c). Unfolding the mechanism of sodium insertion in anatase TiO2 nanoparticles. Adv. Energy Mater. 5:1401142. doi: 10.1002/aenm.201401142
Wu, L., Bresser, D., Buchholz, D., and Passerini, S. (2015b). Nanocrystalline TiO2(B) as anode material for sodium-ion batteries. J. Electrochem. Soc. 162, A3052–A3058. doi: 10.1149/2.0091502jes
Wu, L., Hu, X., Qian, J., Pei, F., Wu, F., Mao, R., et al. (2014). Sb-C nanofibers with long cycle life as an anode material for high-performance sodium-ion batteries. Energy Environ. Sci. 7, 323–328. doi: 10.1039/C3EE42944J
Wu, W., Mohamed, A., and Whitacre, J. F. (2013). Microwave Synthesized NaTi2(PO4)3 as an aqueous sodium-ion negative electrode. J. Electrochem. Soc. 160, A497–A504. doi: 10.1149/2.054303jes
Wu, X., Jin, S., Zhang, Z., Jiang, L., Mu, L., Hu, Y-S., et al. (2015d). Unraveling the storage mechanism in organic carbonyl electrodes for sodium-ion batteries. Sci. Adv. 1:e1500330. doi: 10.1126/sciadv.1500330
Xiao, L., Cao, Y., Xiao, J., Wang, W., Kovarik, L., Nie, Z., et al. (2012). High capacity, reversible alloying reactions in SnSb/C nanocomposites for Na-ion battery applications. Chem. Commun. 48, 3321–3323. doi: 10.1039/c2cc17129e
Xie, F., Zhang, L., Su, D., Jaroniec, M., and Qiao, S-Z. (2017). Na2Ti3O7@N-Doped carbon hollow spheres for sodium-ion batteries with excellent rate performance. Adv. Mater. 29:1700989. doi: 10.1002/adma.201700989
Xiong, H., Slater, M. D., Balasubramanian, M., Johnson, C. S., and Rajh, T. (2011). Amorphous TiO2 nanotube anode for rechargeable sodium ion batteries. J. Phys. Chem. Lett. 2, 2560–2565. doi: 10.1021/jz2012066
Xu, J., Ma, C., Balasubramanian, M., and Meng, Y. S. (2014). Understanding Na2Ti3O7 as an ultra-low voltage anode material for a Na-ion battery. Chem. Commun. 50, 12564–12567. doi: 10.1039/C4CC03973D
Yabuuchi, N., Yoshida, H., and Komaba, S. (2012). Crystal structures and electrode performance of alpha-NaFeO2 for rechargeable sodium batteries. Electrochemistry 80, 716–719. doi: 10.5796/electrochemistry.80.716
Yang, Z., Zhang, J., Kintner-Meyer, M. C. W., Lu, X., Choi, D., Lemmon, J. P., et al. (2011). Electrochemical energy storage for green grid. Chem. Rev. 111, 3577–3613. doi: 10.1021/cr100290v
Yoshida, H., Yabuuchi, N., Kubota, K., Ikeuchi, I., Garsuch, A., Schulz-Dobrick, M., et al. (2014). P2-type Na2/3Ni1/3Mn2/3−xTixO2 as a new positive electrode for higher energy Na-ion batteries. Chem. Commun. 50, 3677–3680. doi: 10.1039/C3CC49856E
Yu, H., Ren, Y., Xiao, D., Guo, S., Zhu, Y., Qian, Y., et al. (2014). An ultrastable anode for long-life room-temperature sodium-ion batteries. Angew. Chem. 126, 9109–9115. doi: 10.1002/ange.201404549
Zhang, B., Ghimbeu, C. M., Laberty, C., Vix-Guterl, C., and Tarascon, J. M. (2016). Correlation between microstructure and Na storage behavior in hard carbon. Adv. Energy Mater. 6:1501588. doi: 10.1002/aenm.201501588
Zhao, C., Avdeev, M., Chen, L., and Hu, Y-S. (2018a). An O3-type oxide with low sodium content as the phase-transition-free anode for sodium-ion batteries. Angew. Chem. Int. Ed. 57, 7056–7060. doi: 10.1002/anie.201801923
Zhao, C., Wang, Q., Lu, Y., Li, B., Chen, L., and Hu, Y-S. (2018b). High-temperature treatment induced carbon anode with ultrahigh Na storage capacity at low-voltage plateau. Sci. Bull. 63, 1125–1129. doi: 10.1016/j.scib.2018.07.018
Zhao, L., Pan, H-L., Hu, Y-S., Li, H., and Chen, L-Q. (2012a). Spinel lithium titanate (Li4Ti5O12) as novel anode material for room-temperature sodium-ion battery. Chinese Physics B 21:28201. doi: 10.1088/1674-1056/21/2/028201
Zhao, L., Zhao, J., Hu, Y-S., Li, H., Zhou, Z., Armand, M., et al. (2012b). Disodium terephthalate (Na2C8H4O4) as high performance anode material for low-cost room-temperature sodium-ion battery. Adv. Energy Mater. 2, 962–965. doi: 10.1002/aenm.201200166
Zhao, M., Huang, J., Guo, X., Chen, H., Zhao, H., Dong, L., et al. (2015). Preparation of Na2Ti3O7/titanium peroxide composites and their adsorption property on cationic dyes. J. Chem. 2015:12. doi: 10.1155/2015/363405
Keywords: sodium ion batteries, cathode, anode, titanium–based composite, order—disorder phase transition
Citation: Wang Y, Zhu W, Guerfi A, Kim C and Zaghib K (2019) Roles of Ti in Electrode Materials for Sodium-Ion Batteries. Front. Energy Res. 7:28. doi: 10.3389/fenrg.2019.00028
Received: 17 December 2018; Accepted: 05 March 2019;
Published: 27 March 2019.
Edited by:
Renaud Bouchet, Université Grenoble Alpes, FranceReviewed by:
Kai Zhu, Harbin Engineering University, ChinaWenping Sun, University of Wollongong, Australia
Copyright © 2019 Wang, Zhu, Guerfi, Kim and Zaghib. This is an open-access article distributed under the terms of the Creative Commons Attribution License (CC BY). The use, distribution or reproduction in other forums is permitted, provided the original author(s) and the copyright owner(s) are credited and that the original publication in this journal is cited, in accordance with accepted academic practice. No use, distribution or reproduction is permitted which does not comply with these terms.
*Correspondence: Karim Zaghib, emFnaGliLmthcmltQGlyZXEuY2E=