- 1Laboratory of Microbial Biotechnology, Faculty of Sciences and Techniques, Sidi Mohamed Ben Abdellah University Fez, Fez, Morocco
- 2Innovation Center, Sidi Mohamed Ben Abdellah University Fez, Fez, Morocco
Being constituents of the effluents of many industries, heavy metals cause severe environmental pollution given the fact that they are recalcitrant and persistent in the environment. Conventional remediation strategies used to treat heavy metals loaded wastewater are neither economical nor environmentally friendly. To overcome these challenges, the rise of a new process that combines energy conservation and recovery was mandatory. Microbial fuel cells have emerged as a promising technology to mitigate environmental pollution; it provides a solution to wastewater treatment and the removal and/or recovery of heavy metals. They are bioelectrochemical systems that utilize the catalytic activity of microorganisms organized in biofilms to oxidize organic or inorganic compounds by producing electric current, thus providing a new opportunity for sustainable energy production and bioremediation. The removal and recovery of metals, such as Cr(VI), V(V), and Cu(II) have been evaluated using both single and double chambered MFCs. The fact that some heavy metals have high redox potential makes it possible to utilize them as effective electron acceptors instead of oxygen in the cathodic chamber of microbial fuel cells. Biotic/Abiotic cathode chambers can not only remove but also recover heavy metals. However, a number of challenges such us: low production rates and limited efficiencies make the application of this technology restricted to lab scale only. In this chapter, we review the removal/recovery of metals from effluents using the microbial fuel cells technology. We'll first summarize the principle of metal removal/recovery in microbial fuel cells, and then provide an overview of literature that attempted to treat metal loaded effluents in both single and double chambered microbial fuel cells while discussing power output, heavy metal removal efficiency and mechanisms involved in the process. Furthermore, the main challenges facing microbial fuel cells and their future applications in the treatment of heavy metals contaminated wastewater will be outlined.
Introduction
Water is a precious commodity that suffers from various forms of pollution and degradation: ecosystems and people's health are directly impacted. Although seemingly inexhaustible, all countries will, in the short or long term, face the problem of its scarcity, which makes wastewater one of the most valuable resources for water and energy, and its treatment a major concern of the public authorities.
Heavy metals pollution is nowadays recognized as one of the most concerning environmental issues. Due to their recalcitrance, persistence in the environment and toxicity, the treatment of heavy metals is of special importance. In recent years, various physical, chemical, and biological treatment approaches for the removal of heavy metals from wastewater have been largely practiced. These methods include chemical precipitation, coagulation-flocculation, adsorption, membrane filtration, and electrochemical treatment technologies (Fu and Wang, 2011). However, in a bid to make the treatment of these metals both economical and eco-friendly, a lot of attention has been raised to develop novel clean approaches for the recovery of metals (Wang and Ren, 2014).
Microbial fuel cells (MFCs) have been proven to be a promising technology to harvest energy and treat wastewater owing to their low-cost and sustainability (Chouler et al., 2016). In their simplest form, MFCs consist of an anodic and a cathodic compartment that are generally separated by a proton exchange membrane (PEM) to avoid the migration of electrolytes from one chamber to the other (Ho et al., 2018). In the anode compartment, bacteria are used as catalysts to break down organic matter and generate electrons and protons. Electrons are then transferred to the cathode via an external circuit, while the protons diffuse through the PEM (Nimje et al., 2012; Mathuriya and Yakhmi, 2014; Miskan et al., 2016). By serving as terminal electron acceptors in the cathode compartment, metals can be electrochemically reduced and eventually be recovered from the cathode surface (Ucar et al., 2017).
This chapter provides a brief introduction to the principle of metal removal and recovery in microbial fuel cells. Studies on metal removal/recovery in MFCs are also presented and reviewed separately in single chambered microbial fuel cells (SCMFCs) and double chambered microbial fuel cells (DCMFCs). Moreover, this review gives an insight into the major challenges holding back the application and the scaling-up of the MFC technology, including the thermodynamic limits, heavy metals biotoxicity, pH imbalance, and membrane biofouling.
Principle of Heavy Metals Removal in Microbial Fuel Cells
In principle, anaerobic microbes forming an electroactive biofilm are used as catalysts in MFCs to harvest energy from the organic matter existing in wastewater (Nancharaiah et al., 2015). During the substrate oxidation process, electrons and protons are released into the aqueous solution (Mathuriya and Yakhmi, 2014). While the electrons produced are transferred from the anode to the cathode via an external circuit, the protons issued from the anaerobic respiration diffuse through the PEM, thus electricity is generated. Once at the cathode, electrons and protons are used for the eventual oxygen reduction reaction to form water (Mathuriya and Yakhmi, 2014; Nancharaiah et al., 2015; He et al., 2016). The concept of electroactive bacteria is far from being a new one: Potter (1911) was the first to report the liberation of electrical energy by both bacteria and yeast back in 1911. Exoelectrogens or electroactive microorganisms can be defined as prokaryotes that have the capability of interacting with charged conductive electrode surfaces, using them as either donors or acceptors of electrons (Nealson, 2017), with both Gram-positive and Gram-negative electricigens reported. This electron transport is carried out via extracellular electron transport (ETT), in which several mechanisms are employed (Kumar et al., 2016). However, both directions of EET are possible; meaning that it is not limited to the anode where this electrode accepts electrons from microorganisms, but it can also occur at the cathode where microorganisms accept electrons from this electrode (Choi and Sang, 2016; Doyle and Marsili, 2018). There are three possible ways used by microorganisms to transfer electrons to the anode electrode, including (a) direct contact; (b) pili/conductive wires; (c) redox mediators or electron shuttle (Kumar et al., 2016). It should be noted that microorganisms can use one or the combination of multiple mechanisms at a time for electron transport.
In general, the process of organic matter oxidation at the anode is coupled to the reduction of oxygen at the cathode (Ucar et al., 2017). However, any compound with a comparable or higher redox potential than oxygen can be reduced at the cathode, thus, elements like permanganate, ferricyanide, nitrate, persulfate, dye molecules, and most importantly heavy metals were used and proven to be efficient electron acceptors in the cathode of MFCs (Mathuriya and Yakhmi, 2014; Wang and Ren, 2014; Nancharaiah et al., 2015; Modestra et al., 2017). This is due to the fact that the reduction of these compounds is thermodynamically favorable which makes the flow of electrons from the anode to the cathode occur spontaneously and without ny external power consumption (Wang and Ren, 2014) (Figure 1).
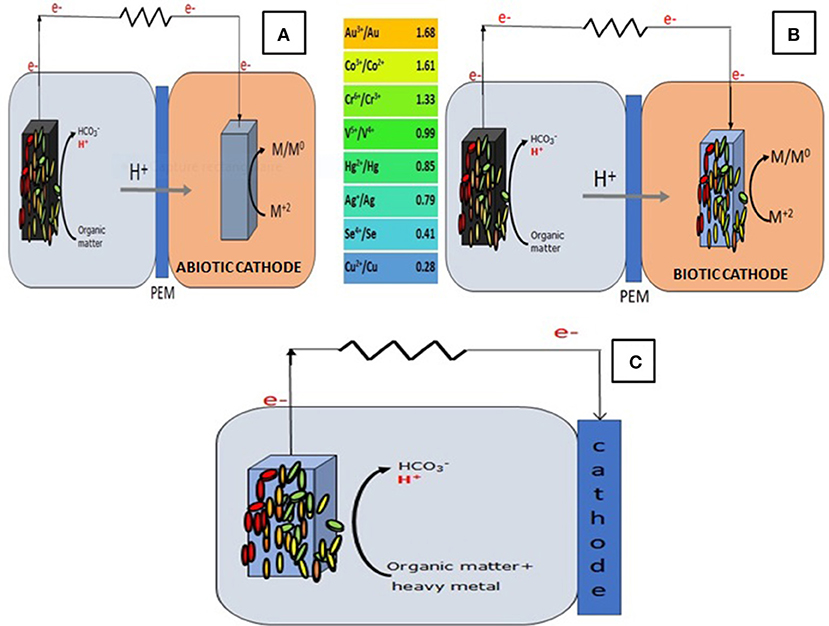
Figure 1. The principle of heavy metal removal in (A) a DCMFC with an abiotic cathode; (B) a DCMFC with a biocathode; (C) an air-cathode MFC.
Heavy Metal Removal Mechanisms
Although a decent amount of studies have investigated the capacity of MFCs to remove heavy metals, there still is a lack of information on the mechanisms involved in the process of their removal. In abiotic cathodes, reduction and deposition at the cathode surface are the commonly investigated removal mechanisms (Colantonio, 2016; Isosaari and Sillanpää, 2017). Besides cathodic reduction, heavy metal removal in abiotic cathodes was demonstrated via chemical precipitation and electrochemical reduction (Colantonio, 2016). In biocathodes, besides the mechanisms mentioned above; heavy metal removal were demonstrated to be induced via a number of other mechanisms that include bioreduction, bioaccumulation, biosorption, and biomineralization (Wu et al., 2017).
Electrode Material
Numerous factors were proven to influence the performance of MFCs including substrate, electron transfer mechanism in the anodic chamber, temperature, pH, terminal electron acceptors, configuration, the type of the PEM and the electrode material (Aghababaie et al., 2015). With the performance of the electrodes significantly affected by the type of material they are fabricated with, all throughout the last decade, many researchers focused on the electrode material because the performance of a MFC directly depends on the kinetics of its electrodes (Mustakeem, 2015).
Anode Material
The step in which the adequate anode material is being selected, is critical for the MFC performance in terms of microbial adhesion, transport of electrons from microbes to the electron acceptor and electrochemical efficiency (Dumitru and Scott, 2016). Therefore, the materials of which anodes are constructed need to possess a number of specific properties that help improve the interaction between the electroactive bacteria and the surface of the electrode as well as current collection. A large variety of anode materials have been evaluated for the enhancement of MFC performances including carbon- and metallic-based materials (Santoro et al., 2017). Owing to their severel characteristics that include being chemically stable, highly conductive, biocompatible and able to create a large surface area, carbon-based materials have been widely employed as anodes in MFCs. Among carbon-based materials, graphite rods (Liu et al., 2004), graphite plates (Rabaey et al., 2003), graphite felt (Zhang, Y. et al., 2012), carbon cloth (Liu et al., 2012), carbon brush (Yang et al., 2017), carbon mesh (Zhang et al., 2013), carbon veil (Gajda et al., 2017), carbon paper (Zhang, Y. et al., 2012), carbon felt (Paul et al., 2017), etc., are used as commercially available anode electrode material and have been examined as anode electrodes for MFCs (Hindatu et al., 2017). On the other hand, the antimicrobial property of metals makes it impossible for bacteria to grow on their surface. However, Baudler et al. (2015) recently demonstrated that this commonly reported antimicrobial characteristic does not apply to electrogens as they have the ability to grow on the surface of these metals, forming a highly performing electroactive biofilm. Thus, metal based materials have been investigated for MFC anodes with the main advantage of being more conductive than carbon based materials, robust and inexpensive. Among them, copper, nickel, silver, gold, and titanium were successfully explored as anode electrode materials (Fan and Liu, 2015).
Cathode Material
The cathode performance is considered a major obstacle holding back the development of MFCs. Thus, the fabrication of cathode materials that combine high power generation and columbic efficiency and reduced expenses is the most crucial and challenging aspect for the MFC technology to be successfully applied, particularly for the purpose of the treatment of wastewater (Rahimnejad et al., 2015). In addition to being robust, a good cathode must be mechanically strong, highly conductive, and possesses catalytic properties (Mustakeem, 2015).
Most of the previously mentioned base materials employed for anodes in MFCs have also been employed for cathodes, with platinum being the most widely used cathode catalyst. In general, cathodes can be classified into two categories: abiotic and biocathodes, while biotic cathodes can be either aerobic or anaerobic (Modestra et al., 2016).
Cathodic Electron Acceptor
During organic matter degradation, electrons released travel to the anodes to eventually be oxidized by electron acceptors at the cathode (Yusuf and Naeyor, 2011). While most studies focused on the electron donor/substrate used in the anode, less attention was drawn to cathodic electron acceptors even though they represent one of the major factors influencing the performance of MFCs. Some of the characteristics that make a good electron acceptor are: fast kinetics, high redox potential, availability in the environment, sustainability, and low cost (Lu and Li, 2012).
So far, oxygen is recognized as one of the most useful and commonly utilized electron acceptors in MFCs owing to its ready availability, high oxidation potential, and the advantage of producing a clean product that is water, once it is reduced (Logan and Regan, 2006). Yet, many studies stated that the oxygen supply required at the cathode is power-hungry (Logan, 2009; Strik et al., 2010). However, due to the urgent need of alternative electron acceptors with diverse redox and more effective cathode reactions, recent studies started to evaluate the feasibility of using different electron acceptors (Ucar et al., 2017).
Besides oxygen, ferricyanide (Wei et al., 2012; Wu et al., 2013; Onyeka et al., 2017) and permanganate (You et al., 2006; Cai et al., 2016) are another commonly used terminal electron acceptors in MFC studies. When used as the cathodic electron acceptor in a MFC, You et al. (2006) reported that permanganate recovered much more electrical power than ferricyanide with a generated maximum power density of 115.60 and 25.62 mW/m2, respectively.
In a bid to improve power generation, reduce costs and expand the MFC based technology in different areas, many researchers begun to consider and investigate the performances and applicability of using alternative electron acceptors (Ucar et al., 2017). It has been recently found that when used as electron acceptors; some heavy metals; known for their extreme toxicity and recalcitrance in the environment but more importantly their rarity, can be deposited and thus recovered at the cathode, suggesting that MFCs are not only capable of generating electricity but have also the ability to eliminate/recover these compounds from wastewater (He et al., 2015). These metals include: uranium, silver, chromium, mercury, copper, among others (Ucar et al., 2017).
Removal of Heavy Metals in MFCs
Both single and double chambered MFCs were employed for the removal of heavy metals, in which the function of the anodic chamber in mainly to break down the organic matter serving as both the carbon and electron donor, while the removal of heavy metals takes place in the anaerobic cathodic compartment (Mathuriya and Yakhmi, 2014). Reduction of metals such as chromium, silver, copper, cobalt, selenite, vanadium, iron, cadmium, and zinc are presented and discussed in the section that follows in both double (abiotic/biocathodes) (Tables 1, 2) and single chambered MFCs (Table 3), separately.
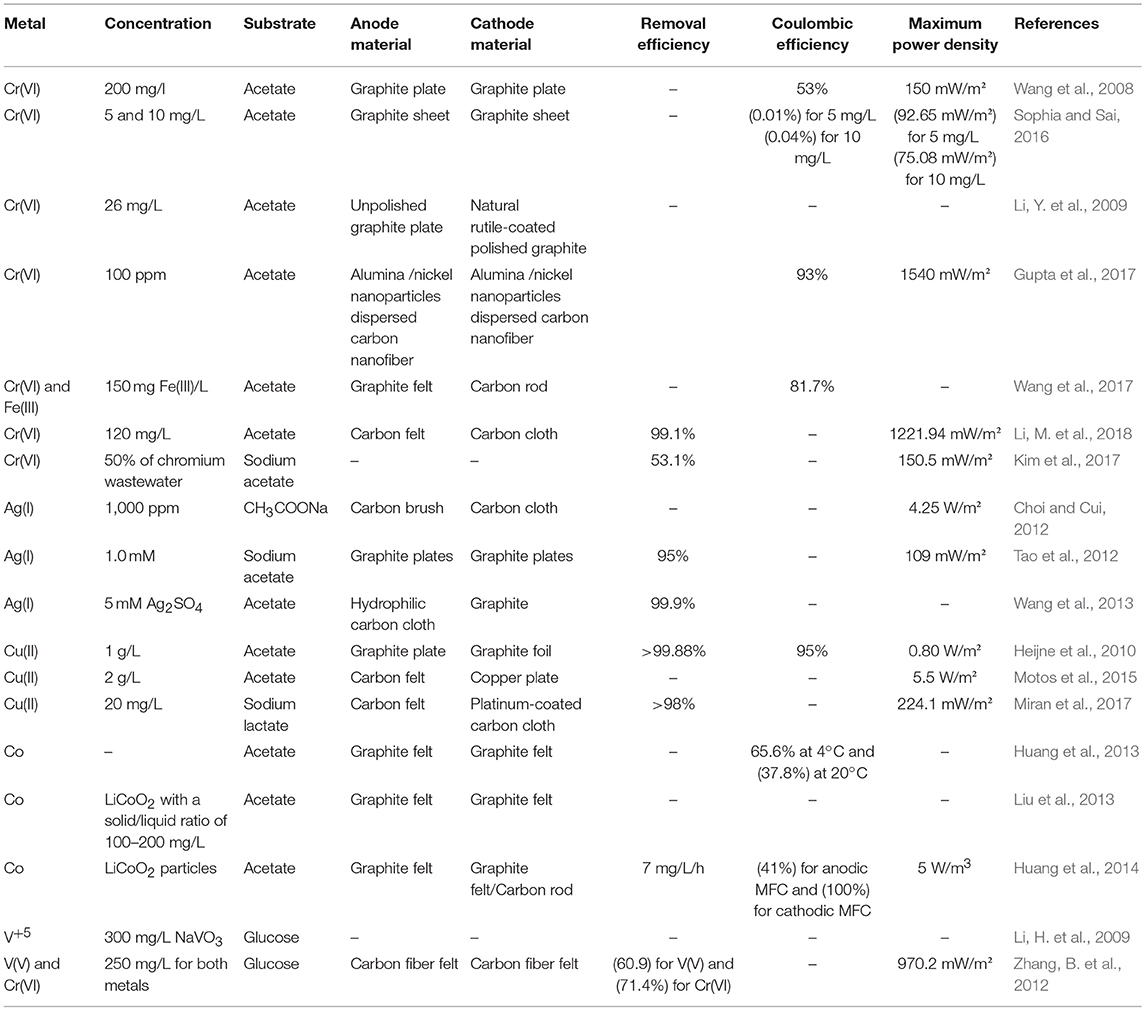
Table 1. Bioelectrochemical removal/recovery of metals in double chambered microbial fuel cells with abiotic cathodes.
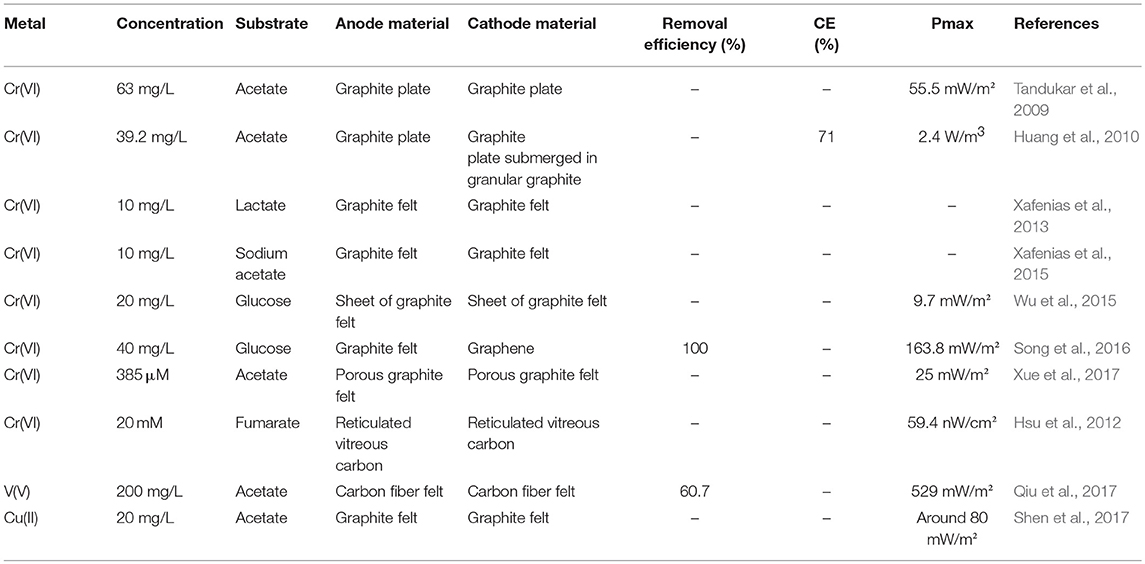
Table 2. Bioelectrochemical removal/recovery of metals in double chambered microbial fuel cells with biocathodes.
In DCMFCs With Abiotic Cathode
Chromium
Chromium (Cr) is available in the environment under two oxidation states: Cr(III) (trivalent) and Cr(VI) (hexavalent) (Oliveira, 2012). Cr(VI) was proven to be toxic, mutagenic and highly carcinogenic (Hamilton et al., 2018; Renitta et al., 2018). Because of its wide range of applications such as tanneries and metal plating, Cr(VI) is known to be one of the most alarming contaminants of the environment, which makes its remediation one of a major concern (Peng et al., 2018; Renitta et al., 2018). Wang et al. (2008) were the first to explore the electrochemical reduction of Cr(VI) in MFCs using graphite plate electrodes. A maximum power density of 150 mW/m2 was generated with a reduction rate of 0.67 g/m3/h at a concentration of 200 mg Cr(VI)/L. With the use of a cost-effective salt bridge instead of an expensive membrane like Nafion or Ultrex, Sophia and Sai (2016) reduced the total of 5 mg/L of chromium and 80% of 10 mg/L with a maximum power density of 92.65 and 75.08 mW/m2, respectively. It should be mentioned that most of the studies in DCMCs used only a proton or cation exchange membrane. Studies with these two types of membranes showed a decline in bioelectricity production and resulted in performances that are less stable. This decrease was explained by the pH imbalance between the two compartments of the MFC. To tackle this problem, Kim et al. (2017) evaluated and compared MFCs performances in the presence of a PEM and a bipolar membrane for the treatment of hexavalent chromium. Better removal efficiencies of Cr(VI) and simultaneous higher bioelectricity were obtained with the bipolar membrane. Moreover, when using the PEM, the lower pH at the cathode led to a decrease in the pH of the anode chamber because of the flow of protons across the PEM, and thus caused the inhibition of the anodic oxidation reaction. This work established that by using a bipolar membrane both current production and chromium removal were improved. In an attempt to reduce the operational costs of MFCs while enhancing the reduction rates of MFCs, Gupta et al. (2017) tested for the first time the feasibility of using an alumina (AA)/nickel (Ni) nanoparticles (NPs)-dispersed carbon nanofiber (CNF)-based cheap electrode in a mediator-less DCMFC. At 100 ppm-concentration, Cr(VI) was completely removed with a maximum power density of 1,540 mW/m2 and a cathodic columbic efficiency of 93%. Fe(III) is known to be an environmentally friendly and cost effective mediator in MFCs. In order to elucidate the mechanism behind Fe(III) mediation and the boost of Cr(VI) reduction, Wang et al. (2017) investigated its role as a mediator in a MFC to enhance the reduction rate of Cr(VI). It was observed that both the conversion of chromium and the cathodic coulombic efficiency (CE) showed an in increase in the presence of Fe(III). In addition, Fe(III) majorly contributed in the decrease the overpotential for Cr(VI) reduction. Higher concentrations of Cr(VI) were successfully removed by Li, M. et al. (2018) in a DCMFC, by utilizing three different types of cathodes including carbon felt, carbon brush cathode and carbon cloth. Amongst the three cathodes, the MFC functioning with the carbon cloth exhibited a higher power density than the other cathodes, while removing the total of 80 mg/L of Cr(VI) via the electrochemical reduction of Cr2O to Cr(OH)3.
Silver
Silver, as a naturally occurring precious metal exists in the effluents of many industries (Birloaga and Vegliò, 2018). Silver has many valuable features and properties such as good electrical and heat conductivity, malleability, ductility, light-reflectivity and strength, which makes this metal find application in electronic, jewelery, and photographic industries (Nawaz and Sengupta, 2017). Due to its limited availability and decreasing natural resources, the recovery of silver from industrial water is essential for environmental and economic profits (Ho et al., 2018). Chemical precipitation, adsorption, biosorption, and bioreduction are amongst the conventional methods that are currently used for Ag(I) removal from wastewater (Li, X. et al., 2018). In a cost-effective MFC, silver recovery efficiencies as high as 99.91–98.26% were obtained with initial concentrations ranging from 50 to 200 ppm, with a maximum power density of 4.25 W/m2 after 8 h operation (Choi and Cui, 2012). In another study, Tao et al. (2012) reported the cathodic reduction of both Ag+ ions and [AgS2O3]− to Ag0, with acetate as electron donor. With an initial metal concentration of 1 mM, power densities of 109 and 35 mW/m−3 for Ag+ and [AgS2O3]− were achieved and 95% of Ag(I) was removed. However, for a better representation of the practical and more complex real silver wastewater, Wang et al. (2013) investigated the recovery of silver from wastewater in the presence of ammonia. A maximum electricity of 3.2 J was generated and 1.6 g of pure silver was recovered and deposited on the cathode, while 1 g COD (83%) was removed from the anode solution simultaneously.
Copper
The increasing imbalance between copper demand and copper supply in the globe has made its extraction/recovery from a vast variety of industrial effluents and radioactive wastes become crucial both from the aspect of economics and environmental protection (Kilicarslan and Saridede, 2013; Ahmed et al., 2016). Additionally, copper is known to be highly toxic and can result in some seriously harmful biochemical effects on human beings, which makes its removal a necessity. Heijne et al. (2010) investigated copper recovery in a DCMFC by the use of a bipolar membrane to separate the two chambers. Removal efficiencies of around 99.88% were obtained with maximum power density of 0.43 W/m2 generated under anaerobic conditions in the cathodic compartment. While most studies attempted the treatment of copper in the cathode department, Miran et al. (2017) demonstrated efficient copper removal in the anodic chamber of a DCMFC by using a sulfate reducing bacteria-enriched anode. They reported that the MFC performance improved at low metal concentrations of Cu2+up to 20 mg/L, this resulted from the stimulation of biological reactions by this metal. A novel cell design was operated by Motos et al. (2015) with the aim of increasing current and power density of a copper reducing DCMFC. To do so, a new strategy was adopted to decrease the internal resistance by making four main changes: reducing the distance between the anode and the cathode from 3 to 0.5 cm, using an anion exchange membrane to reduce the internal resistance, using a copper plate instead of graphite paper as cathode, and carbon felt instead of graphite paper as anode to attain high specific surface area.
Cobalt
Although cobalt is a metal of great value from a biological point of view, as it is an essential enzyme cofactor in most living organisms (Lwalaba et al., 2017), when present in excess, this metal can cause serious health hazards to living being and ecosystems due to its toxicity. Excessive exposure to cobalt was shown to induce numerous diseases such as contact dermatitis, asthma, pneumonia, and lung cancer (Pimentel et al., 2014).
Owing to the high positive potential (+1.61 V vs. SHE) of the Co(III)/Co(II) redox couple, Co(III) is an efficient terminal electron acceptor in MFCs Nancharaiah et al. (2015). Huang et al. (2013) achieved cobalt leaching from LiCoO2 in MFCs by the use of acetate as a substrate with an acclimated bioanode. It was found that factors like solid/liquid ratio, external resistor, solution conductivity, pH, and temperature had an influence on the system performance. A pH of 1.0 and a temperature of 35°C appeared to improve cobalt leaching. The improvement of both cobalt leaching (by 308%) and acid utilization efficiencies (by 171%) was successfully accomplished in DCMFCs by adding a concentration of 10 mg/L of Cu(II) (Liu et al., 2013). It was demonstrated that a pH of 2.0 was more preferable than 3.0. While previous studies focused on the use of either MFCs on MECs for cobalt leaching, a new approach that combines the two technologies was adopted by Huang et al. (2014). The self-driven MFC-MEC system was able to attain the complete cobalt recovery starting by the release of Co(II) from LiCoO2 on the cathodes of MFCs, then using the energy harvested from these MFCs to power MECs where the reduction of Co(II) to final Co(0) took place.
Vanadium
About 38,000 tons of vanadium is produced in the world annually. This element has the property of making steel vibration- and shock- resistant, which is why is it often used as steel additive (Weckhuysen and Keller, 2003). Despite its importance as micro-nutrient, vanadium was proven to be implicated in many human diseases (Mukherjee et al., 2004).
By using the dissimilatory microbes of Rhodoferax ferrierducens as an inoculum in the anode chamber,(Li, H. et al., 2009) achieved the reduction of V5+. Adding 300 mg/L of NaVO3 in the anode increased the maximum current production up to 0.6 mA. A number of parameters affected the reduction of V5+ including: pH, temperature and stirring speed. In another study, the use of two different electron acceptors in the cathodic compartment was reported for the very first time (Zhang, B. et al., 2012). It was possible to simultaneously reduce both V(V) and Cr(VI) after 240 h operation, the V(V) and Cr(VI) reduction efficiencies were 67.9 and 75.4%, respectively. A maximum power density of 970.2 mW/m2 was obtained in this study. It should be noted that the power output obtained in this study was higher than that in MFCs where vanadium was the only electron acceptor.
DCMFCs With Biotic Cathodes
Conventional MFCs consist of bioanodes and abiotic cathodes. Abiotic cathodes usually require the presence of a mediator or a catalyst such as platinum to accomplish the oxygen reduction (He and Angenent, 2006). However, increased expenses, the poor stability for long-term operation caused by catalyst poisoning and the low operational sustainability prevent the practical applications of Pt-based cathodes (Kalathil et al., 2017). Such drawbacks can be vanquished by employing biotic cathodes, in which microorganisms are used to assist cathodic reactions. Biocathodes possess some interesting features that make them present many benefits over abiotic cathodes due to the reduced costs; since pricey mediators or metal catalysts such as platinum can be omitted (Watanabe, 2008). In addition, they eliminate the need for electron mediators and avoid sulfur poisoning of Pt chamber which could enhance the stability and sustainability of MCFs at hostile conditions of wastewaters (Rahimnejad et al., 2015). In a comparative study, Jang et al. (2013) reported that unlike the current from abiotic cathode MFCs that did not change, the current of biotic cathode MCs increased and stabilized after 8 weeks operation. In addition, both power densities and coulombic efficiencies were much higher in biotic cathode MFCs (430 W/m3 and 59.6%) than the MFC with abiotic cathode (257 W/m3 and 15.6%). Electron microscopic observation revealed the production of nanowires by bacteria developed on both the anode and the biocathode. These nanowires can be used by bacteria to accept and transfer electrons from the electrode. The addition of the respiratory inhibitor azide to the catholyte did not affect the abiotic cathode, while the current dropped in MFCs with biotic cathode.
Although metals have been used as electron acceptors in abiotic cathodes, the study of biocathodes for metal elimination/recovery is still in its infancy; hence the small number of work conducted in this area, with chromium being the first and most extensively studied metal. Tandukar et al. (2009) were one of the first groups to adopt biocathodes for the treatment of Cr(VI)-contaminated wastewater. Starting with initial hexavalent chromium concentrations below 80 mg/L, the reduction was relatively fast, however, at 80 mg Cr(VI)/L the reduction became extremely slow. A maximum power density of 55.5 mW/m2 was achieved.
It should be noted that inoculation and MFC architecture play an important role in the improvement of hexavalent chromium reduction rate and power output, as established by Huang et al. (2010). By working with a MFC architecture with a relatively large cathode specific surface area of 340–900 m2 m−3 and employing indigenous bacteria from Cr (VI)-contaminated site as inoculums, Huang et al. (2010) were able to enhance Cr (VI) reduction and power generation using biotic cathode. At an initial hexavalent chromium concentration of 39.2 mg/L, a maximum power density of 2.4 W/m3 was achieved. They also demonstrated that initial Cr (VI) concentration and the conductivity of the solution had an effect on Cr (VI) reduction rate, power generation and coulombic efficiency. Wu et al. (2015) enhanced Cr(VI) reduction efficiency in biocathode MFCs by establishing a novel acclimatization method. After enriching a stable electroactive biofilm on a MFC anode, this electrode was transferred to the cathode and serve as a biotic cathode in a hexavalent chromium reducing MFC. The direct inversion of the bioanode appeared to improve the electricity generation by increasing both voltage and power density and lowering the internal resistance compared to the control. With the material of the cathode being one the most factors limiting MFCs performances, researchers like Song et al. (2016) focused on the fabrication of a simple and effective biocathode for the treatment of hexavalent chromium by using microbially reduced graphene. Compared to graphite felt biocathode (28.6 mW/m2), the maximum power density of the MFC was 5.7 times higher with a graphene biocathode (163.8 mW/m2), with a superior removal of Cr(VI) within 48 h as well. The mechanism behind this enhancement was explained by the fact that more adsorption sites were available owing to the high surface area of the graphene electrode. Besides electrode materials, Xafenias et al. (2015) demonstrated that membranes also affect the performance of MFCs. They reported that the employment of appropriately selective membranes for the separation of the anolyte from the catholyte to prevent the crossover of the negatively charged hexavalent chromium ions to the anodic chamber is crucial, as the presence of Cr(VI) in the anodes of MFCs will brutally and irreversibly inhibit anodic current production.
Besides chromium, vanadium was also studied by Qiu et al. (2017) in the biocathode of a DCMFC. With an initial concentration of 200 mg/L and after 7 d operation, the nearly total removal of V(V) was achieved due to synergistical electrochemical and microbial reductions. A higher maximum power density of 529 mW/m2 was achieved in the MFC with biotic cathode than that in MFC with abiotic cathode 478 mW/m2.
In Air Cathode MFCs
Until now, most studies focused on the reduction of metal ions in anaerobic cathodes of DCMFCs to avoid the migration of metals from the air-cathode to the anode, and to prevent the anodic biofilm from being potentially inhibited. However, the separation of metals and organic matter is not practical when it comes to real wastewater treatment, because both of these elements co-exist in the same water stream (Wang and Ren, 2014). Besides, the high cost of the proton exchange membrane in DCMCs also represents a major obstacle, and the internal resistance is higher than that of SCMFCs which results in lower power output. To defeat these difficulties, recent studies started to consider SCMFCs where the configuration is simplified, the operation cost is reduced and the same solution that contains both contaminants and organic matter is supplied to one chamber. Yet, the number of works conducted in this area is still pretty limited (Wang and Ren, 2014).
Catal et al. (2009) were the first to investigate the capability of a SCMFC to simultaneously generate electricity and remove metals, in this case selenite, using acetate and glucose as carbon sources. With glucose as substrate, selenite did not have any effect on power production up to 125 mg/l. Besides selenium, Abourached et al. (2013) evaluated the impacts of two other heavy metals (Cd and Zn) on power output. High power production (3.6 W/m2) and high Cd (90%) and Zn (97%) removal efficiencies were attained simultaneously.
Fe(III) and Cr(VI) were both evaluated as electron acceptors in SCMFCs by Li et al. (2014). In general, higher metals concentration generally resulted in higher power output, but a chromium concentration of 10 mg/L started to irreversibly inhibit power production. However, iron concentrations did not negatively affect the performances of SCMFCs. The conversion efficiencies of chromium and iron were high (>89%) and coulombic efficiency ranged from 23 to 100%.
In a more recent study by Wu et al. (2018), copper was effectively removed at a concentration of 12.5 mg/L of Cu2+. Simultaneous copper removal and electricity generation were achieved with a removal efficiency of 98.3% and a maximum power density 10.2 W/m3.
Challenges and Opportunities for the Scaling-up of MFCs Treating Heavy Metals
The major challenge facing MFCs has to be their inability to treat all metal ions. The MFC system has been used to reduce various heavy metal ions, including chromium, copper, cobalt, vanadium, and mercury. However, it should be noted that only when the reduction is thermodynamically favorable; meaning that the target metal has a positive standard potential; metals can serve as direct electron acceptors without any external power supply (Nancharaiah et al., 2015). However, metals with comparable or lower redox potentials than the anode potentials cannot spontaneously accept electrons from the cathode and therefore require an external power supply to force the electrons travel to the cathode in order to be reduced. Nickel, lead, cadmium, and zinc are among these metals (Wang and Ren, 2014).
For instance, nickel [Ni(II)] has an electrochemical redox potential of −0.25V (vs. NHE) therefore cannot be spontaneously reduced in a MFC. Qin et al. (2012) investigated the treatment of wastewater containing nickel ion (Ni2+) in both a MEC (microbial electrolysis cell) and a MFC in order to compare the performances of the two. At a concentration of 1,000 mg/L of Ni2+, the removal efficiency with the MEC was three times higher than that with a MFC. The removal efficiencies were 33 and 9% in the MEC and MFC, respectively. They attributed the removal of Ni2+ in the MFC to the adsorption by the membrane and the cathode. These results demonstrated that the nickel reduction at the cathode compartment was unachievable through the bioelectrochemical reaction in the MFC but also indicated that the MEC has a great potential in the efficient removal of Ni2+ from wastewater.
In an interesting attempt to overcome this thermodynamic limit of MFCs, Choi et al. (2014) came up with an innovative configuration using two reactors where one reactor (Cr-MFC) is used to power another reactor (Cd-MFC) for the reduction of cadmium. They managed to successfully compensate for the insufficient voltage and power needed to recover cadmium by combining (in series) two double chambered MFCs. The Cr-MFC operated as a redox-flow battery, and they were able to drain electrical energy from the DCMFC by using an additional passage. In another attempt to recover cadmium, Zhang et al. (2015) stacked MFCs using either Cr(VI) (Cr-MFCs) or Cu(II) (Cu-MFCs) as a final electron acceptor to self-drive MECs using Cd(II) (Cd-MECs) as an electron acceptor. The reduction of Cr(VI) and Cu(II) in MFCs and Cd(II) in MECs was successfully and simultaneously achieved with no external energy supply.
Another challenge that has been holding back the application of MFCs for the removal/recovery of heavy metals is the deterioration of performances and removal efficiencies. Having clear impacts and inhibitory effects on the activity and metabolism of microbes, it is only normal that the biotoxicity of certain heavy metals would negatively affect the performance of MFCs.
Xu et al. (2016) investigated the impacts of various heavy metal ions including: copper, nickel, cadmium, manganese, zinc, chromium, and cobalt on energy output of MFCs. All metals with no exception were proven to be toxic to S. oneidensis MR-1 and showed an inhibition of voltage at a certain concentration. However, at concentrations <100 ug/L for copper and 1 mg/L for cadmium, a significant improvement of voltage output was noticed. These results were explained by the improvement of riboflavin production and bacteria attachment on the electrode by the addition of Cu2+ or Cd2+, resulting in the enhancement of bacterial extracellular electron transfer. In a SCMFC constructed by Wu et al. (2018), the tolerable concentration of copper was ~12.5 mg L−1. A higher concentration of 15 mg/L resulted in a diminution in copper removal and inhibited the electricity production. According to a research by Abourached et al. (2013), heavy metals negatively affected the anodic performance by principally inhibiting the microbes at the anode due to their toxicity.
It should be mentioned that even though tubular MFCs were also employed (Huang et al., 2011), air-cathode and double chambered MFCs remain the mostly used designs for the removal of heavy metals. And although each one of these two configurations has its own benefits, they both come with many significant drawbacks. For example, the advantages of SCMFCs are the simple configuration and the low operational costs owing to the lack of a PEM and the direct utilization of passive air that eliminates the need of aeration (Liu et al., 2005, 2008; Fan et al., 2007). Yet, one of the main disadvantages of PEM-less MFCs is that the CE is much lower than those containing a PEM which is caused by substrate consumption by the oxygen diffused from the cathode to the anode. Besides, the thermodynamic and kinetic constraints of oxygen reduction reaction in the cathode of SCMFCs results in lower power densities (Yang et al., 2016). However, the presence of the PEM which is an essential constituent in DCMFCs because it physically separates the anode compartment from cathode compartment whilst permitting the diffusion of protons from the anode to the cathode, maintains the ion and electron balance, reduces oxygen diffusion to the anode and, thus, results in improved CE values (Lu et al., 2015; Yang et al., 2016). Nevertheless, the use of membranes can create pH imbalances between the anode and the cathode chamber which is caused by the anode chamber acidification developed due to the limited proton transfer across the PEM and the accumulation of protons in this chamber (Logan et al., 2015; Songhe and Bing, 2015). This problem leads to deteriorated performances and a limited extent of power generation by inhibiting the microbial activity. In DCMFCs, the pH imbalance that contributes to potential losses and, therefore, power losses can be lessened by the addition of phosphate buffers during operation to reduce the pH difference between the anode and cathode. However, in addition to being non-sustainable the use of buffers increases the operating costs and material demand (Fornero et al., 2010). To tackle the problem of proton accumulation in the anode chamber that negatively affects both operational stability and electricity generation in DCMFCs, Yang et al. (2016) developed a novel hybrid MFC-stack based on single and double chambered MFCs for self-sustaining pH control. The highest power output was generated by the hybrid stack compared with the stacks that consisted exclusively of SCMFCs or DCMFCs.
Besides the pH imbalance that limits the extent of power generation, the use of PEMs in DCMFCs has another downside to it, which is membrane fouling. This unwanted phenomenon always occurs in MFCs, as biofilm will inevitably grow on and inside separators during long-term operation (Flimban et al., 2018). Therefore, the effects that membrane fouling have on the MFC performance were investigated by many researchers (Christgen, 2010; Ghasemi et al., 2012; Xu et al., 2012; Liu et al., 2014; Songhe et al., 2015; Cheng et al., 2017). As a result of inadequate regular PEM cleaning, Flimban et al. (2018) demonstrated that PEM biofouling in both anodic and cathodic sides, significantly affected CEs and maximum power densities which resulted in a decrease in energy production. It was also revealed by SEM that the significant amount of microbes accumulated and their end-products that caused the formation of a thick biofilm on the PEM surface resulted in a decline as well as the prevention of the passage of H+ from the anodic to the cathodic compartment. In a similar study, organic and inorganic compounds were found out to reinforce the layer of biofouling (Miskan et al., 2016). As the thickness of the biofouling layer increased, the proton conductivity of the Nafion 117 membrane decreased, leading to an increment in membrane resistance due to the limited proton transfer through the membrane. Additionally, the power density of the MFC exhibited a 55% reduction from 1 W/m2 at 2 months of operation to 0.45 W/m2 at 6 months of operation. Since PEMs can be utilized in both single and double chambered MFCs, Xu et al. (2012) studied the effects of biofouling in an air-cathode MFC. They reported that the main characteristics of PEM including: ion exchange capacity, conductivity and diffusion coefficients of cations were significantly reduced after biofouling, leading to the deterioration of performances and a decrease in electricity generation. In addition to the limited cation transfer that caused the physical blockage of charge transfer, membrane biofouling also contributed to cathodic potential loss. However, to guarantee time stability and sustainable electricity generation, the fouled PEM has to be recovered or replaced with a new one (Xu et al., 2012). Since the biofouling of the PEM could occur 6 months after the starting up, authors like Flimban et al. (2018) recommended to clean the PEM membrane every 6–7 months in order to avoid biofouling. Unfortunately, this could be practical on a laboratory scale but not on large scales. Additionally, as PEM is pricey and occupy about 38% capital cost of MFCs (Tang et al., 2010; Songhe et al., 2015); if replaced; the high cost of PEMs would be a big obstacle, especially for the scaling up and future practical application of MFCs. Generally, MFC separators are fabricated with ion-exchange perfluorinated membranes, the most common of them being Nafion. In an effort to overcome the major obstacle of membrane biofouling, Angioni et al. (2017) tested the feasibility of using composite separators based on polybenzimidazole as an alternative to Nafion membrane. Besides their easy synthesis/process, low-cost and chemical stability, polybenzimidazole based membranes lead to a significant boost of power density, operating life, and the efficiency of wastewater treatment with reference to Nafion membrane. In contrast to Nafion, whose surface was colonized by a dense biofilm, which negatively affected the MFC performances over long-term operation, polybenzimidazole membranes protected the MFC from biofouling, as the adhesion of bacteria was inhibited by this polymer (Angioni et al., 2017).
Outlook
Conventional methods used to treat wastewater loaded by heavy metals depend on the origin of the water to be treated and the treatment objective related to the quality of the receiving environment. The main techniques used in wastewater treatment are physical (membrane filtration, ion exchange, etc.), chemical (precipitation, electrochemical methods, etc.), and biological (biosorption, bioremediation, etc.) (Wang and Ren, 2014). Different processes have demonstrated different efficiencies for wastewater. For example, chemical precipitation is the most traditional and widely used technique for the removal of metals from aqueous systems. However, the problem of chemical precipitation is the large amount of toxic sludge generated, which requires additional treatment before disposal. Physical and chemical methods are efficient but require high investment costs and are considered as high consumers of electrical energy.
Biological methods are considered as sustainable technologies of lower impact on the environment. Bioremediation is recognized as profitable, eco-friendly, non-invasive, cheaper than other conventional methods, and it is an enduring solution that can end with the degradation or transformation of environmental contaminants into nontoxic or less toxic forms. It can use microorganisms/enzymes to clean up metal-contaminated wastewater while maintaining regular metabolism (Fernandez et al., 2018). Advantageous characteristics that include small genome size, short replication times, relative simplicity of the cell, rapid evolution, and adaptation to the new environmental conditions made microorganisms the key players in bioremediation technologies (Dvořák et al., 2017; Mary et al., 2018). In general, bioremediation processes include bioaccumulation, biosorption, biotransformation and biomineralization using microbes. Amongst these, biosorption is a promising technology that presents major over conventional treatment methods such as low operational cost; simplicity; high efficiency; minimization of chemical and biological sludge; and the possibility of metal recovery (Fernandez et al., 2018).
Indeed, one of the pillars of the circular economy and the 4th Industrial Revolution is the valorization of chemical wastes accumulating in industry (Dvořák et al., 2017). The microbial fuel cells technology is a suitable candidate process that can valorize wastes and was demonstrated to not only treat chemical wastes but also achieve the recovery of chemical products such as heavy metals while simultaneously producing electrical energy. However, one of the biggest challenges in MFC development is the scaling-up of MFCs for practical applications, which includes increasing the capacity of wastewater treatment and transferring energy from multiple cells in one MFCs system. In general, a deterioration of performances is observed as the size of MFCs increases, probably due to the limitation of mass transfer in larger electrodes (Ge et al., 2013). Owing to the interconnection between many components of the MFC system that include anode, cathode, biofilm formation, and power generation; the scaling-up of MFCs should not be limited to the augmentation of the anode volume, but must also involve the appropriate amplification of electrodes, membranes, and catalyst coating power generation. Moreover, researchers should also focus more on the major challenges addressed in section Challenges and Opportunities for the Scaling-Up of MFCs Treating Heavy Metals; including membrane and cathode biofouling, pH imbalance, and MFCs architecture.
Author contributions
LE, AE, SI, and SE contributed conception of the manuscript and wrote sections of the manuscript. LE and AE wrote the first draft of the manuscript. All authors contributed to manuscript revision, read and approved the submitted version. LE, AE, and SE conception and editing of the manuscript, and final approval of the version to be published. SI contributed to critical revision of the manuscript.
Conflict of Interest Statement
The authors declare that the research was conducted in the absence of any commercial or financial relationships that could be construed as a potential conflict of interest.
Acknowledgments
We would like to thank the National Centre for Scientific and Technological Research of Morocco (CNRST, PPR: Priority research project) with the project number PPR/14.
References
Abourached, C., Catal, T., and Liu, H. (2013). Efficacy of single-chamber microbial fuel cells for removal of cadmium and zinc with simultaneous electricity production. Water Res. 51, 228–233. doi: 10.1016/j.watres.2013.10.062
Aghababaie, M., Farhadian, M., Jeihanipour, A., and Biria, D. (2015). Effective factors on the performance of microbial fuel cells in wastewater treatment – a review. Environ. Technol. Rev. 4, 71–89. doi: 10.1080/09593330.2015.1077896
Ahmed, I. M., Nayl, A. A., and Daoud, J. A. (2016). Leaching and recovery of zinc and copper from brass slag by sulfuric acid. J. Saudi Chem. Soc. 20, S280–S285. doi: 10.1016/j.jscs.2012.11.003
Angioni, S., Millia, L., Bruni, G., Ravelli, D., Mustarelli, P., and Quartarone, E. (2017). Novel composite polybenzimidazole-based proton exchange membranes as ef fi cient and sustainable separators for microbial fuel cells. J. Power Sources 348, 57–65. doi: 10.1016/j.jpowsour.2017.02.084
Baudler, A., Schmidt, I., Langner, M., Greiner, A., and Schroder, U. (2015). Does it have to be carbon? Metal anodes in microbial fuel cells and related bioelectrochemical systems. Energy Environ. Sci. 8, 2048–2055. doi: 10.1039/C5EE00866B
Birloaga, I., and Vegliò, F. (2018). Overview on hydrometallurgical procedures for silver recovery from various wastes. J. Environ. Chem. Eng. 6, 2932–2938. doi: 10.1016/j.jece.2018.04.040
Cai, J., Zheng, P., and Mahmood, Q. (2016). Effect of cathode electron acceptors on simultaneous anaerobic sulfide and nitrate removal in microbial fuel cell. Water Sci. Technol. 73, 947–954. doi: 10.2166/wst.2015.570
Catal, T., Bermek, H., and Liu, H. (2009). Removal of selenite from wastewater using microbial fuel cells. Biotechnol. Lett. 31, 1211–1216. doi: 10.1007/s10529-009-9990-8
Cheng, B., Wang, J., Liu, W., Bi, F., Jia, H., and Zhang, H. (2017). Membrane fouling reduction in a cost effective integrated system of microbial fuel cell and membrane bioreactor. Water Sci. Technol. 76, 653–661. doi: 10.2166/wst.2017.250
Choi, C., and Cui, Y. (2012). Recovery of silver from wastewater coupled with power generation using a microbial fuel cell. Bioresour. Technol. 107, 522–525. doi: 10.1016/j.biortech.2011.12.058
Choi, C., Hu, N., and Lim, B. (2014). Bioresource Technology Cadmium recovery by coupling double microbial fuel cells. Bioresour. Technol. 170, 361–369. doi: 10.1016/j.biortech.2014.07.087
Choi, O., and Sang, B. I. (2016). Extracellular electron transfer from cathode to microbes : application for biofuel production. Biotechnol. Biofuels 9:11. doi: 10.1186/s13068-016-0426-0
Chouler, J., Padgett, G. A., Cameron, P. J., Preuss, K., Titirici, M. M., Ieropoulos, I., et al. (2016). Towards effective small scale microbial fuel cells for energy generation from urine. Electrochim. Acta 192, 89–98. doi: 10.1016/j.electacta.2016.01.112
Christgen, B. (2010). Electricity Generation From Wastewater Using Microbial Fuel Cells : a Study of Electrode and Membrane Materials. Newcastle University.
Colantonio, N. (2016). Heavy Metal Removal From Wastewater Using Microbial Electrolysis Cells. McMaster University.
Doyle, L. E., and Marsili, E. (2018). Weak electricigens : a new avenue for bioelectrochemical research. Bioresour. Technol. 258, 354–364. doi: 10.1016/j.biortech.2018.02.073
Dumitru, A., and Scott, K. (2016). “Anode materials for microbial fuel cells,” in Microbial Electrochemical and Fuel Cells. Fundamentals and Applications, eds K. Scott and E. H. Yu (Sawston, Cambridge: Woodhead Publishing), 10–11.
Dvořák, P., Nikel, P. I., Damborsk?, J., and de Lorenzo, V. (2017). Bioremediation 3.0: engineering pollutant-removing bacteria in the times of systemic biology. Biotechnol. Adv. 35, 845–866. doi: 10.1016/j.biotechadv.2017.08.001
Fan, Y., Hu, H., and Liu, H. (2007). Enhanced Coulombic efficiency and power density of air-cathode microbial fuel cells with an improved cell configuration. J. Power Sources 171, 348–354. doi: 10.1016/j.jpowsour.2007.06.220
Fan, Y., and Liu, H. (2015). “Materials for microbial fuel cells,” in Materials for Low-Temperature Fuel Cells, 1st Edn., eds B. Ladewig, S. P. Jiang, and Y. Yan (Weinheim: Wiley-VCH Verlag GmbH & Co. KGaA), 145–165.
Fernandez, P. M., Vinarta, S. C., Bernal, A. R., Cruz, E. L., and Figueroa, L. I. C. (2018). Bioremediation strategies for chromium removal: current research, scale-up approach and future perspectives. Chemosphere 208, 139–148. doi: 10.1016/j.chemosphere.2018.05.166
Flimban, S. G., Hassan, S. H., Rahman, M. M., and Oh, S. E. (2018). The effect of Nafion membrane fouling on the power generation of a microbial fuel cell. Int. J. Hydrogen Energy. Available online at: https://www.sciencedirect.com/science/article/pii/S0360319918305640?via%3Dihub (Accessed March 19, 2018).
Fornero, J. J., Rosenbaum, M., and Cotta, M. A. (2010). Carbon dioxide addition to microbial fuel cell cathodes maintains sustainable catholyte pH and improves anolyte pH, alkalinity, and conductivity. Environ. Sci. Technol. 44, 2728–2734. doi: 10.1021/es9031985
Fu, F., and Wang, Q. (2011). Removal of heavy metal ions from wastewaters : a review. J. Environ. Manage. 92, 407–418. doi: 10.1016/j.jenvman.2010.11.011
Gajda, I., Greenman, J., Santoro, C., Serov, A., Melhuish, C., Atanassov, P., et al. (2017). Improved power and long term performance of Microbial Fuel Cell with Fe-N-C catalyst in air-breathing cathode. Energy. 144, 1073–1079. doi: 10.1016/j.energy.2017.11.135
Ge, Z., Li, J., Xiao, L., Tong, Y., and He, Z. (2013). Recovery of electrical energy in microbial fuel cells: brief review. Environ. Sci. Technol Lett. 1, 137–141.
Ghasemi, M., Ramli, W., Daud, W., Ismail, M., Rahimnejad, M., Fauzi, A., et al. (2012). Effect of pre-treatment and biofouling of proton exchange membrane on microbial fuel cell performance. Int. J. Hydrogen Energy 38, 5480–5484. doi: 10.1016/j.ijhydene.2012.09.148
Gupta, S., Yadav, A., and Verma, N. (2017). Simultaneous Cr(VI) reduction and bioelectricity generation using microbial fuel cell based on alumina-nickel nanoparticles-dispersed carbon nanofiber electrode. Chem. Eng. J. 307, 729–738. doi: 10.1016/j.cej.2016.08.130
Hamilton, E. M., Young, S. D., Bailey, E. H., and Watts, M. J. (2018). Chromium speciation in foodstuffs: a review. Food Chem. 250, 105–112. doi: 10.1016/j.foodchem.2018.01.016
Hao, L., Zhang, B., Cheng, M., and Feng, C. (2016). Effects of various organic carbon sources on simultaneous V(V) reduction and bioelectricity generation in single chamber microbial fuel cells. Bioresour. Technol. 201, 105–110. doi: 10.1016/j.biortech.2015.11.060
He, C. S., Mu, Z. X., Yang, H. Y., Wang, Y. Z., Mu, Y., and Yu, H. Q. (2015). Electron acceptors for energy generation in microbial fuel cells fed with wastewaters: a mini-review. Chemosphere 140, 12–17. doi: 10.1016/j.chemosphere.2015.03.059
He, L., Du, P., Chen, Y., Lu, H., Cheng, X., Chang, B., et al. (2016). Advances in microbial fuel cells for wastewater treatment. Renew. Sustain. Energy Rev. 71, 388–403. doi: 10.1016/j.rser.2016.12.069
He, Z., and Angenent, L. T. (2006). Application of bacterial biocathodes in microbial fuel cells. Electroanalysis 18, 2009–2015. doi: 10.1002/elan.200603628
Heijne, A. T., Liu, F., Weijden, R., Weijma, J., Buisman, C. J., and Hamelers, H. V. (2010). Copper recovery combined with electricity production in a microbial fuel cell. Environ. Sci. Technol. 44, 4376–4381. doi: 10.1021/es100526g
Hindatu, Y., Annuar, M. S. M., and Gumel, A. M. (2017). Mini-review : anode modification for improved performance of microbial fuel cell. Renew. Sustain. Energy Rev. 73, 236–248. doi: 10.1016/j.rser.2017.01.138
Ho, N. A. D., Babel, S., and Sombatmankhong, K. (2018). Bio-electrochemical system for recovery of silver coupled with power generation and wastewater treatment from silver(I) diammine complex. J. Water Process Eng. 23, 186–194. doi: 10.1016/j.jwpe.2018.04.001
Hsu, L., Masuda, S. A., Nealson, H., and Pirbazari, M. (2012). Evaluation of microbial fuel cell Shewanella biocathodes for treatment of chromate contamination. RSC Adv. 2, 5844–5855. doi: 10.1039/C2RA20478A
Huang, L., Chai, X., Cheng, S., and Chen, G. (2011). Evaluation of carbon-based materials in tubular biocathode microbial fuel cells in terms of hexavalent chromium reduction and electricity generation. Chem. Eng. J. 166, 652–661. doi: 10.1016/j.cej.2010.11.042
Huang, L., Chen, J., Quan, X., and Yang, F. (2010). Enhancement of hexavalent chromium reduction and electricity production from a biocathode microbial fuel cell. Bioprocess Biosyst. Eng. 33, 937–945. doi: 10.1007/s00449-010-0417-7
Huang, L., Li, T., Liu, C., Quan, X., Chen, L., Wang, A., et al. (2013). Synergetic interactions improve cobalt leaching from lithium cobalt oxide in microbial fuel cells. Bioresour. Technol. 128, 539–546. doi: 10.1016/j.biortech.2012.11.011
Huang, L., Yao, B., Wu, D., and Quan, X. (2014). Complete cobalt recovery from lithium cobalt oxide in self-driven microbial fuel cell - Microbial electrolysis cell systems. J. Power Sources 259, 54–64. doi: 10.1016/j.jpowsour.2014.02.061
Isosaari, P., and Sillanpää, M. (2017). Use of sulfate-reducing and bioelectrochemical reactors for metal recovery from mine water. Separ. Purif. Rev. 46, 1–20. doi: 10.1080/15422119.2016.1156548
Jang, J. K., Kan, J., Bretschger, O., Gorby, Y. A., Hsu, L., Kim, B. H., et al. (2013). Electricity generation by microbial fuel cell using microorganisms as catalyst in cathode. J. Microbiol. Biotechnol. 23, 1765–1773. doi: 10.4014/jmb.1310.10117
Kalathil, S., Patil, S. A., and Pant, D. (2017). “Microbial fuel cells: electrode materials,” in Encyclopedia of Interfacial Chemistry: Surface Science and Electrochemistry, eds K. Wandelt and P. Vadgama (Amsterdam: Elsevier), 309–318. doi: 10.1016/B978-0-12-409547-2.13459-6
Kilicarslan, A., and Saridede, M. N. (2013). “Recovery of copper and zinc from brass wastes via ionic liquid leach,” in European Metallurgical Conference (Clausthal-Zellerfeld), 1167–1172.
Kim, C., Rong, C., Eun, Y., Heo, J., Mook, S., Lim, D., et al. (2017). Hexavalent chromium as a cathodic electron acceptor in a bipolar membrane microbial fuel cell with the simultaneous treatment of electroplating wastewater. Chem. Eng. J. 328, 703–707. doi: 10.1016/j.cej.2017.07.077
Kumar, R., Singh, L., and Zularisam, A. W. (2016). Exoelectrogens: recent advances in molecular drivers involved in extracellular electron transfer and strategies used to improve it for microbial fuel cell applications. Renew. Sustain. Energy Rev. 56, 1322–1336. doi: 10.1016/j.rser.2015.12.029
Li, H., Feng, Y., Zou, X., and Luo, X. (2009). Study on microbial reduction of vanadium matallurgical waste water. Hydrometallurgy 99, 13–17. doi: 10.1016/j.hydromet.2009.05.019
Li, M., Zhou, S., Xu, Y., Liu, Z., Ma, F., Zhi, L., et al. (2018). Simultaneous Cr ( VI ) reduction and bioelectricity generation in a dual chamber microbial fuel cell. Chem. Eng. J. 334, 1621–1629. doi: 10.1016/j.cej.2017.11.144
Li, X., Wang, Y., Cui, X., Lou, Z., Shan, W., Xiong, Y., et al. (2018). Recovery of silver from nickel electrolyte using corn stalk-based sulfur-bearing adsorbent. Hydrometallurgy 176, 192–200. doi: 10.1016/j.hydromet.2018.01.024
Li, Y., Lu, A., Ding, H., Jin, S., Yan, Y., Wang, C., et al. (2009). Cr(VI) reduction at rutile-catalyzed cathode in microbial fuel cells. Electrochem. Commun. 11, 1496–1499. doi: 10.1016/j.elecom.2009.05.039
Li, Y., Wu, Y., Puranik, S., Lei, Y., Vadas, T., and Li, B. (2014). Metals as electron acceptors in single-chamber microbial fuel cells. J. Power Sources 269, 430–439. doi: 10.1016/j.jpowsour.2014.06.117
Liu, H., Cheng, S., Huang, L., and Logan, B. E. (2008). Scale-up of membrane-free single-chamber microbial fuel cells. J. Power Sources 179, 274–279. doi: 10.1016/j.jpowsour.2007.12.120
Liu, H., Cheng, S., and Logan, B. E. (2005). Production of electricity from acetate or butyrate using a single-chamber microbial fuel cell. Environ. Sci. Technol. 39, 658–662. doi: 10.1021/es048927c
Liu, H., Liu, H., Ramnarayanan, R., Ramnarayanan, R., Logan, B. E., and Logan, B. E. (2004). Production of electricity during wastewater treatment using a single chamber microbial fuel cell. Environ. Sci. Technol. 38, 2281–2285. doi: 10.1021/es034923g
Liu, J., Liu, L., Gao, B., Yang, F., and Crittenden, J. (2014). Integration of microbial fuel cell with independent membrane cathode bioreactor for power generation, membrane fouling mitigation and wastewater treatment. Int. J. Hydrogen Energy 39, 17865–17872. doi: 10.1016/j.ijhydene.2014.08.123
Liu, J., Qiao, Y., Xian, C., Lim, S., Song, H., and Ming Li, C. (2012). Graphene/carbon cloth anode for high-performance mediatorless microbial fuel cells. Bioresour. Technol. 114, 275–280. doi: 10.1016/j.biortech.2012.02.116
Liu, Y., Shen, J., Huang, L., and Wu, D. (2013). Copper catalysis for enhancement of cobalt leaching and acid utilization efficiency in microbial fuel cells. J. Hazard. Mater. 262, 1–8. doi: 10.1016/j.jhazmat.2013.08.004
Logan, B. E. (2009). Exoelectrogenic bacteria that power microbial fuel cells. Nat. Rev. Microbiol. 7, 375–381. doi: 10.1038/nrmicro2113
Logan, B. E., and Regan, J. M. (2006). Microbial fuel cells-challenges and applications. Environ. Sci. Technol. 40, 5172–5180. doi: 10.1021/es0627592
Logan, B. E., Wallack, M. J., Kim, K. Y., He, W., Feng, Y., and Saikaly, P. E. (2015). Assessment of microbial fuel cell configurations and power densities. Environ. Sci. Technol. Lett. 2, 206–214. doi: 10.1021/acs.estlett.5b00180
Lu, M., and Li, F. Y. (2012). Cathode reactions and applications in microbial fuel cells : a review. Crit. Rev. Environ. Sci. Technol. 42, 2504–2525. doi: 10.1080/10643389.2011.592744
Lu, Z., Chang, D., Ma, J., Huang, G., Cai, L., and Zhang, L. (2015). Behavior of metal ions in bioelectrochemical systems: a review. J. Power Sources 275, 243–260. doi: 10.1016/j.jpowsour.2014.10.168
Lwalaba, J., Lwalaba, W., Zvobgo, G., Fu, L., Zhang, X., Mulembo, T., et al. (2017). Alleviating effects of calcium on cobalt toxicity in two barley genotypes differing in cobalt tolerance. Ecotoxicol. Environ. Saf. 139, 488–495. doi: 10.1016/j.ecoenv.2017.02.019
Mary, J., Karthik, C., Ganesh, R., Kumar, S. S., Prabakar, D., Kadirvelu, K., et al. (2018). Biological approaches to tackle heavy metal pollution: a survey of literature. J. Environ. Manage. 217, 56–70. doi: 10.1016/j.jenvman.2018.03.077
Mathuriya, A. S., and Yakhmi, J. V. (2014). Microbial fuel cells to recover heavy metals. Environ. Chem. Lett. 12, 483–494. doi: 10.1007/s10311-014-0474-2
Miran, W., Jang, J., Nawaz, M., Shahzad, A., Eun, S., Ok, C., et al. (2017). Mixed sulfate-reducing bacteria-enriched microbial fuel cells for the treatment of wastewater containing copper. Chemosphere 189, 134–142. doi: 10.1016/j.chemosphere.2017.09.048
Miskan, M., Ismail, M., Ghasemi, M., Md Jahim, J., Nordin, D., and Abu Bakar, M. H. (2016). Characterization of membrane biofouling and its effect on the performance of microbial fuel cell. Int. J. Hydrogen Energy 41, 543–552. doi: 10.1016/j.ijhydene.2015.09.037
Modestra, J. A., Chiranjeevi, P., and Mohan, S. V. (2016). Cathodic material effect on electron acceptance towards bioelectricity generation and wastewater treatment. Renew. Energy 98, 178–187. doi: 10.1016/j.renene.2016.03.066
Modestra, J. A., Velvizhi, G., Krishna, K. V., Arunasri, K., Lens, P. N. L., Nancharaiah, Y., et al. (2017). “Bioelectrochemical systems for heavy metal removal and recovery,” in Sustainable Heavy Metal Remediation. Environmental Chemistry for a Sustainable World, Vol. 8, eds E. Rene, E. Sahinkaya, A. Lewis, P. Lens (Cham: Springer), 165–198.
Motos, P. R., Heijne, A. T., Weijden, R. V. D., Saakes, M., Buisman, C. J. N., and Sleutels, T. H. J. A. (2015). High rate copper and energy recovery in microbial fuel cells. Front. Microbiol. 6:527. doi: 10.3389/fmicb.2015.00527
Mukherjee, B., Patra, B., Mahapatra, S., Banerjee, P., Tiwari, A., and Chatterjee, M. (2004). Vanadium - An element of atypical biological significance. Toxicol. Lett. 150, 135–143. doi: 10.1016/j.toxlet.2004.01.009
Mustakeem, M. (2015). Electrode materials for microbial fuel cells : nanomaterial approach. Mater. Renew. Sustain. Energy 4, 1–11. doi: 10.1007/s40243-015-0063-8
Nancharaiah, Y. V., Venkata Mohan, S., and Lens, P. N. L. (2015). Metals removal and recovery in bioelectrochemical systems: a review. Bioresour. Technol. 195, 102–114. doi: 10.1016/j.biortech.2015.06.058
Nawaz, T., and Sengupta, S. (2017). Silver recovery from greywater: role of competing cations and regeneration. Separ. Purif. Technol. 176, 145–158. doi: 10.1016/j.seppur.2016.11.076
Nealson, K. H. (2017). Bioelectricity (electromicrobiology) and sustainability. Microb. Biotechnol. 10, 1114–1119. doi: 10.1111/1751-7915.12834
Nimje, V. R., Chen, C., Chen, H., Chen, C., Tseng, M., Cheng, K., et al. (2012). A single-chamber microbial fuel cell without an air cathode. Int. J. Mol. Sci. 13, 3933–3948. doi: 10.3390/ijms13033933
Oliveira, H. (2012). Chromium as an environmental pollutant: insights on induced plant toxicity. J. Bot. 2012, 1–8. doi: 10.1155/2012/375843
Onyeka, A. C., Uzoma, A. H., Ekwutosi, O. T., and Uchechukwu, E. E. (2017). Study on generation of bioelectricity using potassium ferricyanide electron acceptor in microbial fuel cell. Chem. Biomol. Eng. 2, 5–13. doi: 10.11648/j.cbe.20170201.12
Paul, D., Noori, M. T., Rajesh, P. P., Ghangrekar, M. M., and Mitra, A. (2017). Modification of carbon felt anode with graphene oxide-zeolite composite for enhancing the performance of microbial fuel cell. Sustain. Energy Technol. Assess. 26, 77–82. doi: 10.1016/j.seta.2017.10.001
Peng, H., Guo, J., Li, B., Liu, Z., and Tao, C. (2018). High-efficient recovery of chromium (VI) with lead sulfate. J. Taiwan Inst. Chem. Eng. 85, 149–154. doi: 10.1016/j.jtice.2018.01.028
Pimentel, C., Caetano, S. M., Menezes, R., Figueira, I., Santos, C. N., Ferreira, R. B., et al. (2014). Yap1 mediates tolerance to cobalt toxicity in the yeast Saccharomyces cerevisiae. Biochim. Biophys. Acta 1840, 1977–1986. doi: 10.1016/j.bbagen.2014.01.032
Potter, M. C. (1911). Electrical effects accompanying the decomposition of organic compounds. Proc. R. Soc. B Biol. Sci. 84, 260–276. doi: 10.1098/rspb.1911.0073
Qin, B., Luo, H., Liu, G., Zhang, R., Chen, S., Hou, Y., et al. (2012). Bioresource Technology Nickel ion removal from wastewater using the microbial electrolysis cell. Bioresour. Technol. 121, 458–461. doi: 10.1016/j.biortech.2012.06.068
Qiu, R., Zhang, B., Li, J., Lv, Q., Wang, S., and Gu, Q. (2017). Enhanced vanadium (V) reduction and bioelectricity generation in microbial fuel cells with biocathode. J. Power Sources 359, 379–383. doi: 10.1016/j.jpowsour.2017.05.099
Rabaey, K., Lissens, G., Siciliano, S. D., and Verstraete, W. (2003). A microbial fuel cell capable of converting glucose to electricity at high rate and efficiency. Biotechnol. Lett. 25:1531. doi: 10.1023/A:1025484009367
Rahimnejad, M., Adhami, A., Darvari, S., Zirepour, A., and Oh, S.-E. (2015). Microbial fuel cell as new technology for bioelectricity generation: a review. Alexandr. Eng. J. 54, 745–756. doi: 10.1016/j.aej.2015.03.031
Renitta, J., Pamela, J., Anoop Kumar, Y., and Desai, N. (2018). Biosorption and biotransformation of hexavalent chromium [Cr(VI)]: a comprehensive review. Chemosphere 207, 255–266. doi: 10.1016/j.chemosphere.2018.05.050
Santoro, C., Arbizzani, C., Erable, B., and Ieropoulos, I. (2017). Microbial fuel cells : from fundamentals to applications. A review. J. Power Sources 356, 225–244.doi: 10.1016/j.jpowsour.2017.03.109
Shen, J., Huang, L., Zhou, P., Quan, X., and Li, G. (2017). Correlation between circuital current, Cu (II) reduction and cellular electron transfer in EAB isolated from Cu (II) -reduced biocathodes of microbial fuel cells. Bioelectrochemistry 114, 1–7. doi: 10.1016/j.bioelechem.2016.11.002
Song, T., Jin, Y., Bao, J., Kang, D., and Xie, J. (2016). Graphene/biofilm composites for enhancement of hexavalent chromium reduction and electricity production in a biocathode microbial fuel cell. J. Hazard Mater. 317, 73–80. doi: 10.1016/j.jhazmat.2016.05.055
Songhe, Z., and Bing, H. (2015). “The influence of pH spitting on the internal resistance in microbial fuel cells,” in 2015 International Conference on Mechatronics, Electronic, Industrial and Control Engineering (MEIC-15) (Shenyang), 1587–1591.
Songhe, Z., Bing, H., and Yuxin, H. (2015). “Effects of three types of separator membranes on the microbial fuel cells performance,” in 2015 International Conference on Mechatronics, Electronic, Industrial and Control Engineering (Shenyang: Atlantis Press; Meic), 1592–1596.
Sophia, A. C., and Sai, S. (2016). Modified microbial fuel cell for Cr(VI) reduction and simultaneous bio-electricity production. J. Environ. Chem. Eng. 4, 2402–2409. doi: 10.1016/j.jece.2016.04.025
Strik, D. P. B. T. B., Hamelers, H. V. M., and Buisman, C. J. N. (2010). Solar energy powered microbial fuel cell with a reversible bioelectrode. Environ. Sci. Technol. 44, 532–537. doi: 10.1021/es902435v
Tandukar, M., Huber, S. J., Onodera, T., and Pavlostathi, S. G. (2009). Biological chromium(VI) reduction in the cathode of a microbial fuel cell. Environ. Sci. Technol. 43, 8159–8165. doi: 10.1021/es9014184
Tang, X., Guo, K., Li, H., Du, Z., and Tian, J. (2010). Microfiltration membrane performance in two-chamber microbial fuel cells. Biochem. Eng. J. 52, 194–198. doi: 10.1016/j.bej.2010.08.007
Tao, H. C., Gao, Z. Y., Ding, H., Xu, N., and Wu, W. M. (2012). Recovery of silver from silver(I)-containing solutions in bioelectrochemical reactors. Bioresour. Technol. 111, 92–97. doi: 10.1016/j.biortech.2012.02.029
Ucar, D., Zhang, Y., and Angelidaki, I. (2017). An overview of electron acceptors in microbial fuel cells. Front. Microbiol. 8:643. doi: 10.3389/fmicb.2017.00643
Wang, G., Huang, L., and, Zhang, Y. (2008). Cathodic reduction of hexavalent chromium [Cr(VI)] coupled with electricity generation in microbial fuel cells. Biotechnol. Lett. 30, 1959–1966. doi: 10.1007/s10529-008-9792-4
Wang, H., and Ren, Z. J. (2014). Bioelectrochemical metal recovery from wastewater: a review. Water Res. 66, 219–232. doi: 10.1016/j.watres.2014.08.013
Wang, Q., Huang, L., Pan, Y., Quan, X., and Li, G. (2017). Impact of Fe (III) as an effective electron-shuttle mediator for enhanced Cr(VI) reduction in microbial fuel cells : reduction of diffusional resistances and cathode overpotentials. J. Hazard Mater. 321, 896–906. doi: 10.1016/j.jhazmat.2016.10.011
Wang, Y.-H., Bai-Shi, W., Bin, P., Qing-Yun, C., and Wei, Y. (2013). Electricity production from a bio-electrochemical cell for silver recovery in alkaline media. Appl. Energy 112, 1337–1341. doi: 10.1016/j.apenergy.2013.01.012
Watanabe, K. (2008). Recent developments in microbial fuel cell technologies for sustainable bioenergy. J. Biosci. Bioeng. 106, 528–536. doi: 10.1263/jbb.106.528
Weckhuysen, B. M., and Keller, D. E. (2003). Chemistry, spectroscopy and the role of supported vanadium oxides in heterogeneous catalysis. Catal. Today 78, 25–46. doi: 10.1016/S0920-5861(02)00323-1
Wei, L., Han, H., and Shen, J. (2012). Effects of cathodic electron acceptors and potassium ferricyanide concentrations on the performance of microbial fuel cell. Int. J. Hydrogen Energy 37, 12980–12986. doi: 10.1016/j.ijhydene.2012.05.068
Wu, C. H., Lai, C. Y., Lin, C. W., and Kao, M. H. (2013). Generation of power by microbial fuel cell with ferricyanide in biodegradation of benzene. Clean Soil Air Water 41, 390–395. doi: 10.1002/clen.201200198
Wu, M. S., Xu, X., Zhao, Q., and Wang, Z. Y. (2017). Simultaneous removal of heavy metals and biodegradation of organic matter with sediment microbial fuel cells. RSC Adv. 7, 53433–53438. doi: 10.1039/C7RA11103G
Wu, X., Zhu, X., Song, T., Zhang, L., Jia, H., and Wei, P. (2015). Effect of acclimatization on hexavalent chromium reduction in a biocathode microbial fuel cell. Bioresour. Technol. 180, 185–191. doi: 10.1016/j.biortech.2014.12.105
Wu, Y., Zhao, X., Jin, M., Li, Y., Li, S., Kong, F., et al. (2018). Copper removal and microbial community analysis in single-chamber microbial fuel cell. Bioresour. Technol. 253:372–377. doi: 10.1016/j.biortech.2018.01.046
Xafenias, N., Zhang, Y., and Banks, C. J. (2013). Enhanced performance of hexavalent chromium reducing cathodes in the presence of Shewanella oneidensis MR-1 and lactate. Environ. Sci. Technol. 47, 4512–4520. doi: 10.1021/es304606u
Xafenias, N., Zhang, Y., and Banks, C. J. (2015). Evaluating hexavalent chromium reduction and electricity production in microbial fuel cells with alkaline cathodes. Int. J. Environ. Sci. Technol. 12, 2435–2446. doi: 10.1007/s13762-014-0651-7
Xu, J., Sheng, G., Luo, H., Li, W., and Wang, L. (2012). Fouling of proton exchange membrane (PEM) deteriorates the performance of microbial fuel cell. Water Res. 46, 1817–1824. doi: 10.1016/j.watres.2011.12.060
Xu, Y. S., Zheng, T., Yong, X. Y., Zhai, D. D., Si, R. W., Li, B., et al. (2016). Trace heavy metal ions promoted extracellular electron transfer and power generation by Shewanella in microbial fuel cells. Bioresour. Technol. 211, 542–547. doi: 10.1016/j.biortech.2016.03.144
Xue, H., Zhou, P., Huang, L., Quan, X., and Yuan, J. (2017). Cathodic Cr (VI) reduction by electrochemically active bacteria sensed by fluorescent probe. Sens Actuators B Chem. 243, 303–310. doi: 10.1016/j.snb.2016.11.154
Yang, Q., Liang, S., Liu, J., Jv, J., and Feng, Y. (2017). Analysis of Anodes of microbial fuel cells when. Catalysts 7:312. doi: 10.3390/catal7110312
Yang, W., Li, J., Ye, D., Zhang, L., Zhu, X., and Liao, Q. (2016). A hybrid microbial fuel cell stack based on single and double chamber microbial fuel cells for self-sustaining pH control. J. Power Sources 306, 685–691. doi: 10.1016/j.jpowsour.2015.12.073
You, S., Zhao, Q., Zhang, J., Jiang, J., and Zhao, S. (2006). A microbial fuel cell using permanganate as the cathodic electron acceptor. J. Power Sources 162, 1409–1415. doi: 10.1016/j.jpowsour.2006.07.063
Yusuf, O. L., and Naeyor, B. (2011). A novel electron acceptor for microbial fuel cells : nature of circuit connection on internal resistance. J. Biochem. Technol. 2, 216–220.
Zhang, B., Feng, C., Ni, J., Zhang, J., and Huang, W. (2012). Simultaneous reduction of vanadium(V) and chromium(VI) with enhanced energy recovery based on microbial fuel cell technology. J. Power Sources 204, 34–39. doi: 10.1016/j.jpowsour.2012.01.013
Zhang, F., Xia, X., Luo, Y., Sun, D., Call, D. F., and Logan, B. E. (2013). Improving startup performance with carbon mesh anodes in separator electrode assembly microbial fuel cells. Bioresour. Technol. 133, 74–81. doi: 10.1016/j.biortech.2013.01.036
Zhang, Y., Sun, J., Hu, Y., Li, S., and Xu, Q. (2012). Bio-cathode materials evaluation in microbial fuel cells : a comparison of graphite felt, carbon paper and stainless steel mesh materials. Int. J. Hydrogen Energy 37, 16935–16942. doi: 10.1016/j.ijhydene.2012.08.064
Keywords: single chambered microbial fuel cell, double chamber, biocathodes, heavy metals, wastewater treatment, challenges, pH imbalance, membrane fouling
Citation: Ezziat L, Elabed A, Ibnsouda S and El Abed S (2019) Challenges of Microbial Fuel Cell Architecture on Heavy Metal Recovery and Removal From Wastewater. Front. Energy Res. 7:1. doi: 10.3389/fenrg.2019.00001
Received: 28 June 2018; Accepted: 11 January 2019;
Published: 30 January 2019.
Edited by:
Vânia Oliveira, University of Porto, PortugalReviewed by:
Mirella Di Lorenzo, University of Bath, United KingdomEnrico Marsili, Nanyang Technological University, Singapore
Copyright © 2019 Ezziat, Elabed, Ibnsouda and El Abed. This is an open-access article distributed under the terms of the Creative Commons Attribution License (CC BY). The use, distribution or reproduction in other forums is permitted, provided the original author(s) and the copyright owner(s) are credited and that the original publication in this journal is cited, in accordance with accepted academic practice. No use, distribution or reproduction is permitted which does not comply with these terms.
*Correspondence: Soumya El Abed, c291bXlhLmVsYWJlZEB1c21iYS5hYy5tYQ==