- 1Chemical and Biomolecular Engineeering, The University of Tennessee, Knoxville, TN, United States
- 2Bredesen Center for Interdisciplinary Research and Education, The University of Tennessee, Knoxville, TN, United States
- 3Oak Ridge National Laboratory, Oak Ridge, TN, United States
- 4Georgia Institute of Technology, School of Civil and Environmental Engineering, Atlanta, GA, United States
Valorization of waste streams is becoming increasingly important to improve resource recovery and economics of bioprocesses for the production of fuels. The pyrolysis process produces a significant portion of the biomass as an aqueous waste stream, called bio-oil aqueous phase (BOAP), which cannot be effectively converted into fuel. In this report, we detail the separation and utilization of this stream for the production of electrons, hydrogen, and chemicals, which can supplement fuel production improving economics of the biorefinery. Separation methods including physical separation via centrifugal separator, chemical separation via pH manipulation, and electrochemical separation via capacitive deionization are discussed. Bioelectrochemical systems (BES) including microbial fuel cells (MFCs), microbial electrolysis cells (MECs), and electro-fermentation processes are reviewed for their potential to generate current, hydrogen, and chemicals from BOAP. Recent developments in MECs using complex waste streams and electro-active biocatalyst enrichment have resulted in advancement of the technology toward performance metrics closer to commercial requirements. Current densities above 10 A/m2 have been reported using BOAP, which suggest further work to demonstrate the technology at pilot scale should be undertaken. The research on electro-fermentation is revealing potential to generate alcohols, diols, medium chain fatty acids, esters, etc. using electrode-based electrons. The ability to derive electrons and chemical building blocks from waste streams illustrate the advancement of the BES technology and potential to push the frontiers of bioenergy generation one step further toward development of a circular bioeconomy.
Introduction
Production of biofuels from biomass can occur in the biorefinery via a number of pathways. The three pathways being investigated most prominently are: (a) biochemical conversion or fermentation to alcohols, (b) thermoconversion to hydrocarbon fuels via pyrolysis bio-oil, and (c) hydrothermal liquefaction (HTL), also via bio-oil to produce hydrocarbon fuels (USDOE, 2014). The latter pathway is more suitable for wet biomass such as algae and wastes, including sludge, while the earlier two pathways are more suitable for lignocellulosic biomass. Since the oxygen content of the feedstock is usually very high, in the range of 30–40% by wt, the efficiency of producing a biofuel depends heavily on the deoxygenation processes. Also, water is integral part of the process streams, due to the intrinsic water content of the feedstock and its production during the chemical reactions involved in pretreatment. The high oxygen content and the presence of water result in an organics-rich aqueous phase after initial processing of biomass. In the case of fermentation, fuel production occurs in the aqueous phase; however, for the latter two pathways where higher temperatures or gas-phase reactions are employed, post-conversion cooling results in condensation of water in the product stream. The aqueous phase is usually emulsified with organics, and is called bio-oil. In catalytic pyrolysis, the aqueous phase separates out, while in fast and intermediate pyrolysis, the aqueous phase remains emulsified with the oil phase (Elliott et al., 2009). Water is sometimes added to the emulsion to effect phase separation. The resulting aqueous phase, termed as bio-oil aqueous phase (BOAP), contains a large fraction of the heavily oxygenated compounds derived from biomass. The aqueous phase is not ideal for fuel production and alternate means of valorizing this material are usually needed (Huber et al., 2004; Kechagiopoulos et al., 2006; Fu et al., 2012; Elkasabi et al., 2015; Paasikallio et al., 2015; Mukarakate et al., 2017). Recovery of the carbon and energy content from this aqueous phase can be an important aspect of improving the conversion efficiency of the thermochemical biorefinery process.
In the biochemical pathway, separation of the alcohol fuel from the fermentation broth results in wastewater containing residual sugars, fermentation products such as organic acids, aldehydes, etc., as well as pre-treatment products including lignin-degradation products like phenols and sugar-degradation products such as furanic compounds (Humbird et al., 2011). Thus, all biorefinery processes generate an aqueous phase, which results in a waste or is treated as a low value stream. A number of different methods have been investigated to recover the carbon and energy from the aqueous waste (Pirwitz et al., 2016; Campanario and Ortiz, 2017; Mukarakate et al., 2017). Conversion of the aqueous phase organics to fuel molecules has been reported with a HZSM-5 catalyst with a 40% conversion of the BOAP organics (Mukarakate et al., 2017). Due to the low degree of conversion, additional processes may be necessary for better valorization. An indirect route to fuels via supercritical oxidation and Fischer Tropsch reactions has also been suggested (Campanario and Ortiz, 2017). This pathway was reported to have a 38.5% carbon efficiency and included four steps requiring pressure swing adsorption, distillation, and hydrocracking. Aqueous phase resulting from HTL of algal biomass has been suggested as a substrate for additional biomass growth to improve algal biorefining yields (Pirwitz et al., 2016).
Several new methods of valorization have been reported in recent years including use of bioelectrochemical systems (BESs) (Borole et al., 2009b; Thygesen et al., 2010; Borole, 2016; Lu and Ren, 2016). Different types of BESs exist including microbial fuel cells (MFCs), microbial electrolysis cells (MECs), electro-fermentation, etc. (Logan and Regan, 2006; Logan et al., 2008; Liu et al., 2010; Rabaey and Rozendal, 2010; Logan and Rabaey, 2012). Electricity is a product of MFCs, which may or may not result in effective valorization due to the low price of electricity; however, MECs that generate hydrogen, can provide an important ingredient required for hydrocarbon fuel production. The organic phase of the bio-oil also contains high levels of oxygen, requiring hydro-deoxygenation to produce a fuel. Typically, natural gas is used to supply the hydrogen. Deriving the hydrogen from an intrinsic waste stream, however, can offer a valuable proposition. This can reduce the fossil fuel required, thus reducing greenhouse gas (GHG) emissions. A third method called electro-fermentation has been reported in recent years for production of chemicals using electrons as a reducing power or redox control to produce reduced products (Schievano et al., 2016). Production of alcohols, organic acids, diols, etc. has been demonstrated via this approach using waste streams, e.g., production of propanediol from glycerine (Xafenias et al., 2015).
Prior to the emergence of electro-fermentation, a similar approach was used to reduce carbon dioxide to reduced products termed “electro-fuels” (ARPA-E, 2014). While the goal was to produce fuels, the method could only produce volatile fatty acids and was, therefore, unsuccessful (Nevin et al., 2011). This result was due to the huge requirement for electrons to reduce CO2 (Desloover et al., 2012). It was recognized that use of partially reduced substrates available in waste streams can alleviate the high demand for reducing equivalents. Thus, research to use these waste-derived substrates was initiated (Rabaey et al., 2011). In this report, we discuss the prospect of marrying the electro-fermentation process with anode processes extracting electrons from waste, thereby resulting in the whole product being derived from waste.
Pyrolysis bio-oil has application-impeding properties including high content of oxygen and water and low values of pH as shown in Table 1 (IEA, 2004).
High fractions of water and oxygen in bio-oil lead to inefficient combustion, while high oxygen content makes bio-oil reactive and unstable. Furthermore, the presence of acidic compounds in bio-oil causes corrosion during storage/transportation and instabilities or chemical aging. In addition, the high viscosity of bio-oil causes handling problems during processing and utilization. Due to these impeding properties, bio-oil has to be treated by physical/chemical processes prior to utilization. Such processes may include:
• Extraction of hydrophobic and hydrophilic chemicals from bio-oil using solvent extraction
• Reduction of the acidity of bio-oil by adding alkali to increase the pH
• Separation of the aqueous and organic phases of bio-oil
• Separation of acidic components from bio-oil to utilize in microbial electrolysis applications.
Through these separations processes, the quality of bio-oil can be improved, while separated valuable components can be used in broader applications. Innovative technologies including centrifugal contactors, for continuous pH-neutralization of bio-oil and simultaneous phase separation, and capacitive deionization (CDI) for acid separation, can be employed in separations processes to facilitate the utilization of acidic bio-oil components in resource recovery. Specifically, extracted organic acids can be utilized in MECs to produce hydrogen, which can be further utilized to upgrade bio-oil through hydrodeoxygenation (HDO). Hydrogen provided by MECs for HDO may replace hydrogen supplied by natural gas (He and Song, 2014). By removing organic acids from bio-oil, its acidity and corrosivity problems can be resolved.
Recent investigations have focused on multiple aspects of the biomass to biofuels and bioproducts conversion processes leading to new insights into biorefining. A better understanding of the composition of streams, separation of complex mixtures into chemical groups, employment of novel biocatalytic and electrocatalytic steps and integration of these steps can lead to create value in the biorefinery. This paper reviews these developments with focus on valorization of biomass-derived waste streams for production of electricity, hydrogen, fuels, and chemicals. The focus is bio-oil derived from biomass via pyrolysis, which is compared with wastes from other biorefinery pathways and food industry processes. The use of BOAP for production of electricity and hydrogen is reviewed with the goal of evaluating the progress toward practical feasibility and achievement of productivities and current densities needed for commercial consideration of BESs (Lewis and Borole, 2017; Lewis et al., 2018). The findings are compared with those using other waste streams and conversion platforms. Production of chemicals via electro-fermentation using electrons derived from BOAP is also discussed along with a review of the existing literature on bioelectrochemical conversion pathways, which can contribute to the development of novel schemes for a bio-electro-refinery in the coming decade.
Bio-oil Components and Carbon in Aqueous Waste
A number of variations of the thermochemical pyrolysis method of biomass breakdown have been reported. These include fast pyrolysis, intermediate pyrolysis, catalytic pyrolysis, and an alteration of the fast pyrolysis with recycle of the tail gas called tail gas recycle pyrolysis (TGRP). The ultimate goal is production of hydrocarbon biofuels; however, the need for generating higher value products such as chemicals has been recognized and, thus, variations allowing optimization of the product portfolio to meet the desired needs are important. The differences in the products generated from the pyrolysis step via these approaches are shown in Table 2. The biomass used in all these studies was switchgrass. Due to the presence of hundreds of compounds in these pyrolysis oils and the use of different analytical methods, not all chemicals are measured/reported for all streams. Table 2, however, provides a fair quantification of the major components of the bio-oil and the associated aqueous phase. It should be observed that the aqueous phase typically contains higher levels of the carboxylic acids, which are typically responsible for the acidity of the bio-oil and are candidates for separation and conversion into products besides fuels. Table 2 shows that the total carbon present in the aqueous phase of the fast and intermediate pyrolysis is much higher than that for TGRP and catalytic pyrolysis. Several furans and methoxy phenols are present in the aqueous phase resulting from the fast and intermediate pyrolysis; however, they are typically deoxygenated in the TGRP and catalytic pyrolysis, resulting in lower concentration in the aqueous phase associated with those bio-oils. Ren et al. identified the presence of 2-methoxyphenol; 2-methyl, 4-methoxyphenol; and 2,6-dimethoxyphenol in the intermediate pyrolysis product (Ren et al., 2016). The combined concentration of these three methoxyphenols was reported to be 0.58 g/L, which represents 4.5% of the total COD present in the aqueous phase. The level of phenols and furans in the aqueous phase can be important if biological processing of the stream is desired as discussed further in section Bioelectrochemical Platform Converting “Waste to Electrons to Products.”
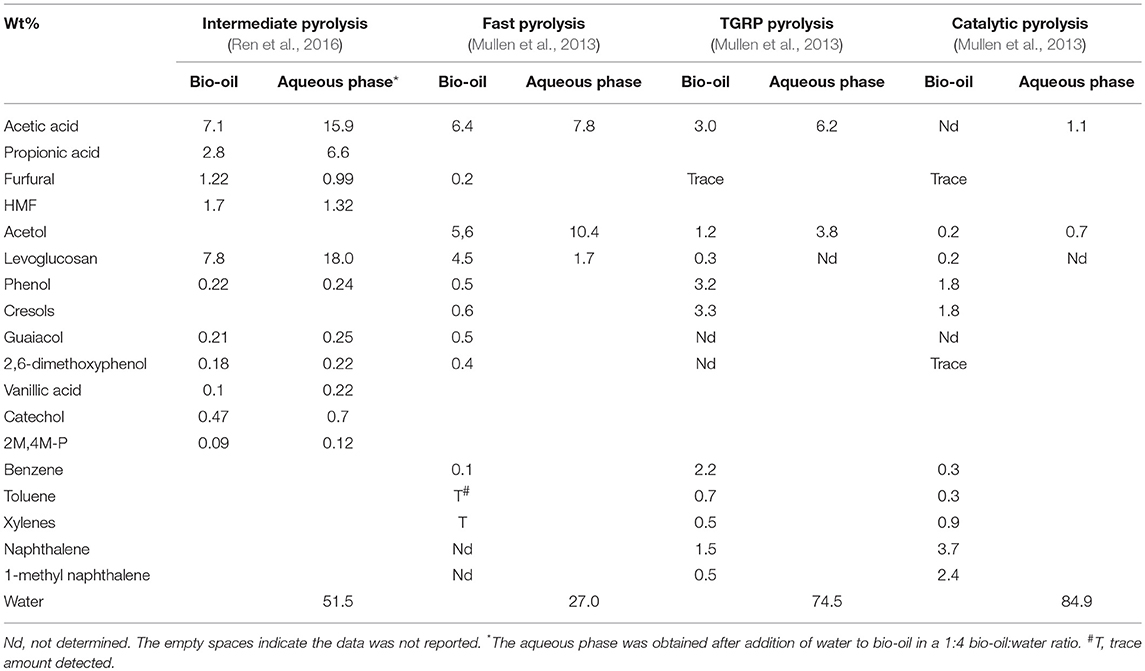
Table 2. Comparison of chemical composition of pyrolysis bio-oil and the corresponding aqueous phase (wt%) generated during pyrolysis or via subsequent separation.
Separation Schemes
In order to separate the various components in bio-oil, a number of different methods have been employed by researchers including physical separation, chemical separation, and separations using external energy, e.g., electrochemical energy. Various physicochemical upgrading methods have been investigated for bio-oil. Phase separation to separate the aqueous and organic components has been a critical initial step before further processing of bio-oil.
Studies conducted recently for the separation of BOAP via a separation scheme involving density-based separation, chemical separation via pH control and electro-chemical separation as shown in Figure 1, are further discussed below. It should be noted that these separation schemes have not yet been applied at real scale and are still in the research development stage.
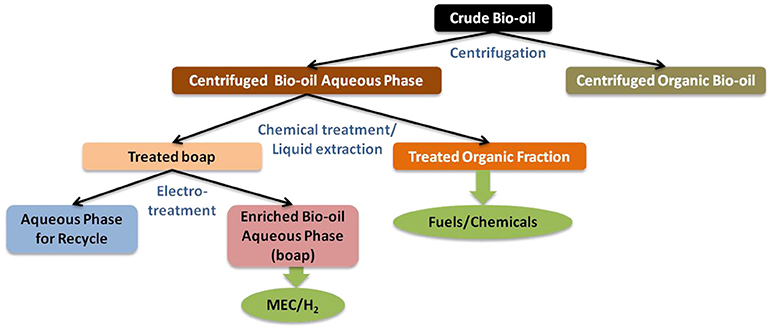
Figure 1. Separation scheme integrating physical, chemical, and electro-separations to fractionate bio-oil for production of hydrogen, fuels and chemicals. Figure reprinted with permission from (Borole, 2015).
Density-Based Separations
Bio-oil contains aqueous and organic phases, which can be separated based on their density differences. Although settling in storage tanks may be an effective separation method, it may require long residence times due to the small density difference between the two phases. Effective phase separation, even for relatively small density differences, can be achieved by continuous-flow centrifuges. One such device is the centrifugal contactor (McFarlane et al., 2010), which combines a shear regime for intimate contact between two liquid phases to facilitate mass transfer and a phase separation regime where a centrifugal force is utilized to separate the two phases. Because it combines effective mixing of two liquids and phase separation, this device has been described in the literature (Kim et al., 2017) as a process intensification device.
Chemical Separations
Chemical separations can be achieved by water addition, pH neutralization, and solvent extraction among other methods. In reality, bio-oil contains a wide range of chemicals, which are not separated individually. Instead, chemical separations are targeting the separation of chemical groups such as acids, alcohols, furans, phenols, ketones, etc.
Water Addition
Water addition forms two phases (Chan and Duff, 2010; Vitasari et al., 2011; Jacobson et al., 2013), and has been commonly employed to separate hydrophilic components (Rasrendra et al., 2011; Vitasari et al., 2011; Xu et al., 2011; Wei et al., 2014; Park et al., 2016). The solubility and polarity of various species influence their partition in the aqueous and organic phases after water addition. Organic acids (e.g., acetic and propionic acids) and levoglucosan are preferentially partitioned in water due to their high solubility (Ren et al., 2017). Furans, including furanone and furfural, have similar partition coefficients to those of acetic acid (Vitasari et al., 2011). Although phenolics including guaiacol and syringol have lower polarity, a considerable amount of such compounds can be partitioned in water (Ren et al., 2017). Song et al. investigated the influence of aqueous electrolyte solutions and solid salts on bio-oil phase separation (Song et al., 2009).
According to Park et al. (2016), water addition to aqueous bio-oil, leads to the formation of two phases, a bio-oil organic phase (BOOP) and a BOAP. Hydrophilic compounds remain in BOAP, while hydrophobic compounds partition mostly into BOOP. The partitioning of the organic compounds was quantified by Park et al. (2016) and showed that about 2/3 of the organic fraction ends up in the aqueous phase, when a 1:4 bio-oil:water ratio is used (Figure S1).
Solvent Extraction
Some investigators studied the separation of various chemical groups with organic solvents. Ren et al. (2017) separated a number of chemicals using sequentially water, hexane, chloroform, petroleum ether, and ethyl acetate. Using chloroform, they reported the highest extraction efficiency for phenolics, furans, and ketones. Ethyl acetate was employed to extract organic acids. For the same solvent-to-feed ratio, the number of chemicals extracted by solvents decreased in the following order: ethyl acetate, chloroform, petroleum, and hexane (Ren et al., 2017).
Among various solvents including dichloromethane, ethyl acetate, cyclohexane, and butyl acetate, dichloromethane demonstrated the highest extraction efficiency for such chemicals as alcohols and acids from bio-oil (Tian et al., 2011). Liquid extraction was also investigated by Wei et al. (2014) using petroleum ether, hexane, and chloroform. Chloroform was reported as the most effective in extracting guaiacols and phenols. Adding chloroform at a volume ratio of 1:1 to the BOAP (obtained after addition of water to bio-oil at a volume ratio of 1:1), Wei et al. (2014) reported extraction of 85.7 wt% of the total guaiacol and phenols. In addition, Ma and Agblevor separated bio-oil fractions after sequentially contacting bio-oil with increasing polarity solvents: hexane, toluene, chloroform, and methanol (Ma and Agblevor, 2014). They reported increasing viscosity of the fractions with increasing solvent polarity. The conclusion of these studies is that liquid extraction can be used to separate valuable chemical groups and also improve the quality of bio-oil.
A simple centrifugation of the whole bio-oil has shown that a significant portion of the organic compounds partitions into the aqueous phase, even without addition of external water. This centrifuged portion of the bio-oil is termed AqBO, which is different from BOAP, derived via water addition. Park et al. (2016) investigated solvent extraction of the aqueous portion of the bio-oil (AqBO), for the purpose of producing fuel. Specifically, they used hexadecane (C16) and octane (C8) as representatives of diesel and gasoline, respectively, for the extraction of organics from aqueous bio-oil. They reported the use of the following two approaches: (i) sequential addition of water followed by solvent extraction and (ii) combined addition of water and organic solvent. Sequential and combined extraction have been reported as shown in Figure S2. Combined extraction was reported to extract more chemicals than those separated by sequential extraction (Figure S3); however, sequential extraction leads to less solvent partitioning into the water, which may have two advantages: (i) lower mass of organic solvents lost and (ii) in the case of a subsequent microbial electrolysis process, microbes will not be exposed to potentially toxic organic solvents. The purpose of solvent extraction experiments was to enrich the solvent with organic components of the aqueous bio-oil. The mass balances, however, demonstrate that the mass gained by the solvent was very small, which means that a relatively small amount of organic compounds was partitioned into the solvent. Thus, although solvent extraction may be useful in the characterization of aqueous bio-oil, it may not be a practical separation approach for certain types of bio-oil, such as the switchgrass bio-oil.
pH Neutralization
It has been reported that pH neutralization of switchgrass aqueous-phase bio-oil leads to enhanced separation of organic compounds from a homogeneous, single-phase solution (Park et al., 2016). This behavior suggests that pH neutralization could be continuously performed in a centrifugal contactor, as shown in Figure 2, so that the rejected organic phase during pH neutralization in the shear regime of the contactor could be continuously removed from the heavier aqueous-phase bio-oil in the phase-separation regime. This process was successfully demonstrated in the literature (Park et al., 2017). In the case that the residence time in the centrifugal contactor is not long enough to allow organic precipitation to reach equilibrium, use of a static mixer was recommended to mix the two phases prior to entering the centrifugal contactor, so that organic precipitation occurs over a longer period of time. Through pH neutralization, 15-20% of the aqueous bio-oil precipitates as an organic phase, thus, facilitating the separation of phenolic compounds. The block diagram for a pH neutralization process is shown in Figure S4.
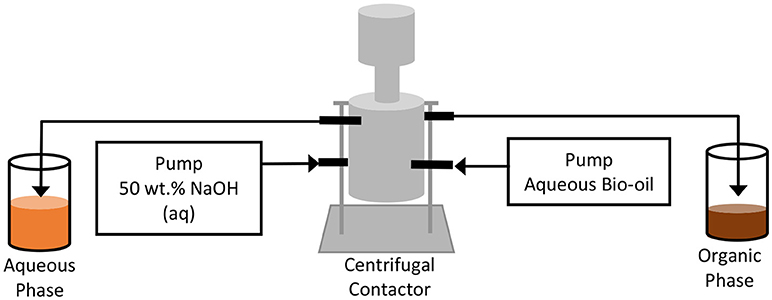
Figure 2. Continuous pH neutralization of aqueous bio-oil and phase separation of the produced aqueous and organic phases using a centrifugal contactor. Figure reprinted with permission from (Park et al., 2017). Copyright (2017) American Chemical Society.
Other Separations
Various aqueous streams contain carboxylic acids that could be utilized for production of value added products if available at higher concentration or without contaminants. Production of medium-chain fatty acids, esters, and hydrogen are some of the examples reported in the literature (Liu et al., 2005; Andersen et al., 2016). Membrane and electrochemical separations have been used to separate the short chain carboxylic acids. Anderson et al. have used electrolytic separation combining membranes and electrochemical gradient to concentrate acetate from dilute solutions (Andersen et al., 2014). Another method used recently to capture acidic compounds is capacitive deionization, which is based on charging carbon electrodes of high surface area to attract counter ions (Park et al., 2018). The separation was coupled to microbial electrolysis for hydrogen production as shown in Figure 3. Such schemes allow integrated separation-conversion enabling integration with biorefineries and generation of added value from the waste streams.
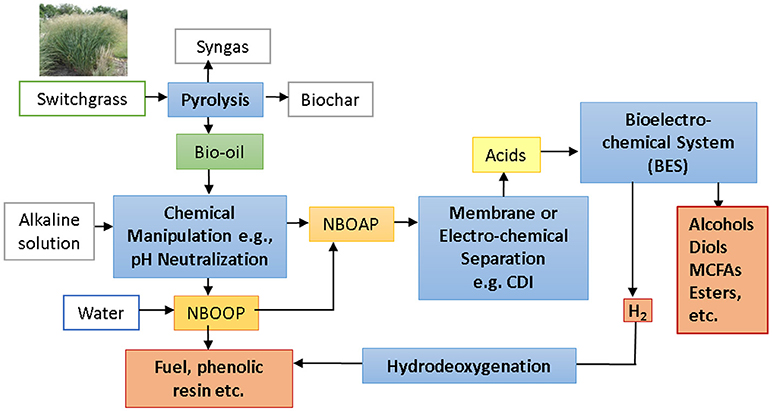
Figure 3. A flow chart for a Bio-Electro-Refinery showing production of switchgrass bio-oil, chemical manipulation including pH neutralization, followed by phase separation using centrifugal contactor, and chemical production in a BES using the acid-rich aqueous phase. (NBOOP: neutralized bio-oil organic phase, NBOAP: neutralized bio-oil aqueous phase). [Switchgrass photo: https://www.gardenia.net/rendition.slider_detail/uploads/plant/1433256670-603d263a69fa7639a/Panicum_virgatum_Cloud_Nine_2009_09.jpg].
Bioelectrochemical Platform Converting “Waste to Electrons to Products”
In the conventional biorefinery pathways, conversion of biomass to biofuels and bioproducts proceeds via chemical intermediates. The bioelectrochemical approach uses an alternate route via electrons as the key intermediates. The breakdown of biomass-derived molecules, particularly those present in waste streams culminates in production of electrons in the anode, which are used to build new molecules using additional co-substrates in the cathode. This concept of conversion of waste/biomass-derived macromolecules to electrons coupled to conversion of the electrons to products is illustrated in Figure 4. The anodic process may involve up to three biological conversion steps: hydrolysis, fermentation and exoelectrogenesis, depending on the complexity of the waste feedstock. Simple substrates such as acetate only require the last step, while simple sugars may only require latter two steps. Most waste feedstocks will contain a wide range of compounds and may require breakdown of the macromolecules and biopolymers to monomers prior to fermentation and exoelectrogenesis. Supply of different substrates into the cathode can lead to different products using suitable catalysts. This concept is the basis for a new biorefinery platform called waste biorefinery (Lu and Ren, 2016; Lewis and Borole, 2017) or bioelectrochemical biorefining (Borole, 2017a), which can potentially be more efficient than the conventional processes due to incorporation of the intrinsically efficient biocatalytic and electrocatalytic processes (Borole, 2015).
Anodic Processes
Bioelectrochemistry of Primary Reactions Leading to Electroactivity
While the ultimate goal of the anodic processes is to generate electrons, a synergy between the exoelectrogens and the fermenters and hydrolytic microbes is necessary for effective conversion of wastes. The aqueous phase from pyrolysis, BOAP, has shown to require a high level of synergy between the fermentative organisms and exoelectrogens for effective production of current in the anode (Lewis et al., 2018). This is due to the wide range of compounds present in the BOAP as shown in section Bio-Oil Components and Carbon in Aqueous Waste. Synergy between these microbial groups has been reported even in single substrate fermentative systems. Use of ethanol in the anode was shown to enrich ethanol-fermenting Acetobacterium and acetate-utilizing exoelectrogens (Parameswaran et al., 2010). In addition, hydrogen scavenging organisms were reported. Use of propionic acid as a substrate also indicated enrichment of fermenters via two pathways going through formation of acetate/formate or acetate/hydrogen, followed by exoelectrogenic conversion (Hari et al., 2016a). Use of complex substrates result in a much diverse population of fermenters and exoelectrogens. Conversion of biomass-derived streams has been studied by various groups (Thygesen et al., 2010; Wang et al., 2011; Lewis et al., 2015; Borole, 2016; Shen et al., 2016). Lalaurate et al. used a synthetic two-step process separating fermentation and exoelectrogenesis to convert cellulose into fermentation byproducts, which were then utilized in an MEC anode (Lalaurette et al., 2009). Thygessen et al., used wheat straw as feedstock and predicted the presence of xylan degrading organisms and those producing acetate and propionate based on metabolites released, but the microbial species were not identified. Similarly, Marone et al., used molasses and other hydrolysates-derived fermentation effluent streams in the anode, and reported a range of fermentation intermediates, however, did not characterize the microbial species (Marone et al., 2017). The information on complex microbial communities present in bioanode treating mixed substrate feedstocks is limited. Lewis et al. recently reported characterization of microbial population treating switchgrass-derived BOAP, which showed a dependence between the fermenters and the exoelectrogens (Lewis et al., 2018). This was one of the first detailed account of the microbial community treating complex feedstocks directly fed into anode. Open and closed-circuit experiments were conducted to show the relationship between the fermenters and the exoelectrogens. The end-products of fermentation such as acetate, form substrate for the exoelectrogens and accumulate if an electron sink is not provided such as under open circuit conditions. Thus, the syntrophy between the two groups was clearly illustrated (Lewis et al., 2018).
The relative population of the two groups can have a large impact on the overall rate of conversion. The population dynamics can reveal insights into the functioning of the community. The microbial population included Firmicutes, Bacteroidetes, and γ-Proteobacteria as the dominant families. These families were reported to use a division of labor strategy to facilitate conversion of the complex feedstock (Lewis et al., 2018). Due to the presence of various chemical groups in the BOAP, different functional groups of microbes are necessary for effective conversion of the substrate. While specific allocation of the microbial species identified in the consortium to chemical groups was not reported, a number of distinct families of fermenting organisms were identified and their potential functions discussed. These interactions are shown in Figure 5. The Firmicutes population included Clostridia, in which fermentative activity is well documented, including that in bioelectrochemical systems (Jung and Regan, 2007; Rismani-Yazdi et al., 2007). Bacteroidetes and γ-Proteobacteria have shown to persist in sugar-fed bioanode systems (Ishii et al., 2014). The exoelectrogen population included β- and δ-Proteobacteria, specifically, Geobacteraceae, Rhodocyclaceae, and Comamonadaceae. The latter two families have diverse metabolic potential and besides exoelectrogenic activity (Ginige et al., 2005; Xing et al., 2010), they also have been reported to show fermentative ability (Rismani-Yazdi et al., 2007; Borole et al., 2009a; Hesselsoe et al., 2009; Oren, 2014; Lewis et al., 2015). Thus, they were observed to potentially play both roles in the community. The exoelectrogens–fermenter synergy results from cross-feeding of intermediates (Zeng et al., 2017; Lewis et al., 2018), which has been reported by other groups illustrating the syntrophy in bioanode fed with other fermentable substrates as well (Freguia et al., 2007; Parameswaran et al., 2009; Kiely et al., 2011; Hari et al., 2016b). Use of a complex substrate such as BOAP in a bioanode results in a complex web of microbial interactions as shown in Figure 5. The two major mechanisms employed by the community in such environments are division of labor and syntrophy. The former results from the necessity to handle a wide range of substrates including acids, aldehydes, ketones, anhydrosugars, furans, phenols, etc. present in the BOAP. Secondly, the accumulation of intermediates from one group of microbes requires a different set of microbes, thus, leading to syntrophy as observed between fermenters and exoelectrogens. Additionally, the presence of inhibitory compounds such as furans and phenols are likely to create microenvironments, not ideal for certain organisms. Zeng et al. characterized the microbial community associated with conversion of key furanic and phenolic substrates in bioanode (Zeng et al., 2015), however, further work is necessary to delineate the interactions between these organisms and other fermenters and exoelectrogens in the community.
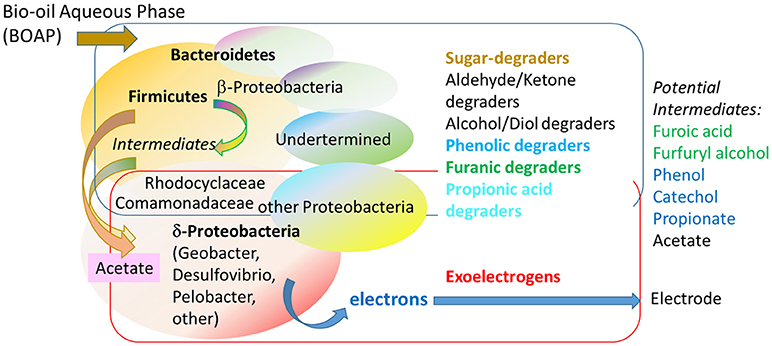
Figure 5. Biochemical interactions within anode chamber leading to electroactivity via microbial syntrophy and division of labor transforming BOAP into electrons. A fermentative population with diverse functionalities is necessary to convert the sugars and other degradation products in BOAP to intermediates, which are ultimately converted to electrons by exoelectrogens. Determination of the details of the interactions between various members of the complex consortium require further research and sophisticated techniques such as omics to be employed for identification of the role of individual members.
Conversion of Furanic and Phenolic Compounds
Furanic and phenolic compounds, generated from the degradation of sugar and lignin polymers during thermal or thermochemical pretreatment of lignocellulosic biomass for biofuel production, are widely found in the BOAP (Jones et al., 2009; Ren et al., 2017). Furanic and phenolic compounds contribute to the instability and corrosiveness of the bio-oil, as well as the BOAP (Jones et al., 2013). In addition, furanic, and phenolic compounds are among the most toxic and inhibitory components of biomass degradation products (Klinke et al., 2004; Monlau et al., 2014), making it a challenge for the waste-to-electrons conversion in the bioelectrochemical platform.
The most predominant biomass-derived furanic compounds are furfural and 5-hydroxymethyl furfural (Rasmussen et al., 2014). Phenolic compounds derived from biomass can be found in alcohol, aldehyde, and acid forms with various degrees of methoxylation (e.g., syringic, vanillic, p-hydroxy acids) (Klinke et al., 2004; Monlau et al., 2014). Depending upon the type of biomass, pretreatment method and conditions, furanic compounds are present at a total concentration up to 5 g/L, while phenolic compounds are present at a total concentration up to 3 g/L (Mills et al., 2009).
A number of studies have used furanic and phenolic compounds as primary substrates in bioanodes of bioelectrochemical systems (Table 3). In most of these studies, the removal of various parent furanic and phenolic compounds was nearly complete. Several furanic and phenolic compounds were utilized as the sole carbon source to support current generation in MFCs or MECs, including 5-hydroxymethyl furfural, furfural, syringic acid, vanillic acid, 4-hydroxybenzoic acid, 4-hydroxybenzaldehyde, 4-hydroxyacetophenone, and phenol (Borole et al., 2009b; Luo et al., 2009; Huang et al., 2011; Zeng et al., 2015). However, contradicting findings have been reported where furfural and phenol were not converted in bioanodes (Catal et al., 2008; Zeng et al., 2017). Whether or not and to what degree a furanic or phenolic compound is converted in a bioanode is related to a number of factors, such as the microbial community structure and capability, acclimation method and status, presence of alternative electron acceptors, and other MEC operating conditions, which vary from system to system (Table 3).
Biotransformation extent and pathways
There are limited studies on the biotransformation pathways of furanic and phenolic compounds in bioanodes. Based on a series of reports on two furanic (furfural, 5-hydroxymethyl furfural) and three phenolic compounds (syringic acid, vanillic acid, 4-hydroxybenzoic acid), the conversion of furanic and phenolic compounds in bioanodes occurred via two sequential processes: fermentation, followed by exoelectrogenesis (Zeng et al., 2015, 2016b, 2017). The complex parent compounds were first fermented to intermediate, simpler metabolites, among which acetate was then used as a key substrate in the subsequent exoelectrogenesis (Figure 3). Consistent with the two-step processes, presence of both fermentative and exoelectrogenic bacterial species in bioanodes was reported in several studies where furanic and phenolic compounds were used as bioanode substrates (Lewis et al., 2015; Zeng et al., 2015; Marone et al., 2016). In these bioanodes, Proteobacteria was found to be a dominant phylum, which contains putative exoelectrogens such as Geobacter spp. and various fermentative bacteria. Firmicutes and Bacteroidetes were also present in bioanodes, containing putative degraders of furanic and phenolic compounds (Lewis et al., 2015; Zeng et al., 2015). Beyond the findings based on furanic and phenolic compounds, it has been widely accepted that combination of fermentation and exoelectrogenesis is a necessity for bioanodes to process complex, fermentable substrates, which cannot be directly utilized by exoelectrogenic bacteria (Freguia et al., 2008; Kiely et al., 2011).
Multiple partitioning of electron equivalents takes place during the conversion of furanic and phenolic compounds to electric current in MEC bioanodes. First, in the fermentation process, the electron equivalents are distributed between fermentation metabolites and fermentative biomass (Figure 3). Based on a typical biomass true yield coefficient of 0.18 g biomass-COD/g degradable-COD for fermentative bacteria (Rittmann and McCarty, 2001), ~82% of electron equivalents are transferred to fermentation metabolites. However, depending on the extent and pathways of fermentation, only a portion of the electron equivalents may end up in acetate or H2, which can be further utilized in exoelectrogenesis; the remaining is non-exoelectrogenic end products (e.g., recalcitrant aromatic products) (Figure 6). In the subsequent exoelectrogenesis, ~14–43% of the electron equivalents are associated with exoelectrogenic biomass (Wilson and Kim, 2016), and the remaining is contributing to electric current. It is noteworthy that not all furanic and phenolic compounds were fully fermented to acetate. Instead, for some compounds, the majority of electron equivalents remained in non-exoelectrogenic end-products (Zeng et al., 2017). Zeng et al. (2018) reported that 50–72% of the electron equivalents of syringic acid, furfural and 5-hydroxymethyl furfural was accounted as current, whereas only 9–12% of electron equivalents of vanillic and 4-hydroxybenzoic acids were converted to current. Therefore, the fraction of electron equivalents used for current production is largely controlled by the extent of fermentative transformation (i.e., acetate production), and thus can vary substantially among different compounds and systems.
A few studies have investigated the biotransformation pathways of furanic and phenolic compounds in bioanodes (Zeng et al., 2015, 2017; Hedbavna et al., 2016; Marone et al., 2016). Several biotransformation metabolites, prior to the furanic or aromatic ring cleavage, were identified (Hedbavna et al., 2016; Marone et al., 2016; Zeng et al., 2017). Zeng et al. (2017) reported that biotransformation of three structurally similar phenolic compounds (syringic acid, vanillic acid, and 4-hydroxybenzoic acid) was initiated by demethylation and decarboxylation reactions common to all three compounds. However, only the aromatic ring of syringic acid was cleaved, whereas the transformation of vanillic acid and 4-hydroxybenzoic acid stopped before their aromatic rings were cleaved, producing catechol and phenol, respectively, which were persistent under the conditions of this study (Figure 7). Such difference in the biotransformation extent resulted in different levels of acetate, and correspondingly different current production (Zeng et al., 2017). Earlier studies suggested that 4.5 mol of acetate could be produced during the biotransformation of 1 mol of syringic acid under anaerobic conditions (i.e., 1.5 mol from two demethylation reactions and 3 mol from aromatic ring cleavage) (Bache and Pfennig, 1981; Brune and Schink, 1992). Thus, without aromatic ring cleavage, 1 mol of vanillic acid could produce up to 0.75 mol of acetate from its only methoxy group, and 4-hydroxybenzoic acid (without any methoxy group) could not produce any acetate (Figure 7), consistent with the findings reported by Zeng et al. (2017). It was unclear why catechol and phenol were not further degraded in the above-mentioned study. One possible reason is that under the conditions of this study, the bioanode microbial community did not possess the CoA-thioester substitution pathway for aromatic ring cleavage (Zeng et al., 2017). Nevertheless, none of the above-mentioned studies has fully identified biotransformation metabolites to establish complete pathways for furanic or phenolic compounds in bioanodes.
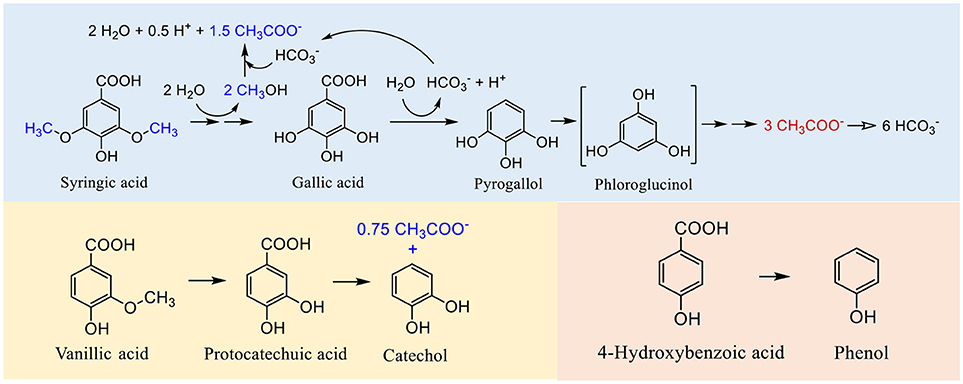
Figure 7. Biotransformation pathways of syringic acid, vanillic acid, and 4-hydroxybenzoic acid. Different amounts of acetate were produced from these compounds as shown in the acetate-producing reactions (drawn based on material presented in Zeng et al., 2017).
Inhibitory effect
Although furanic and phenolic compounds are well-known dark fermentation inhibitors, their potential inhibitory effect on bioanode processes has been scarcely reported. Catal et al. (2008) reported that syringaldehyde, trans-4-hydroxy-3-methoxy and 4-hydroxy cinnamic acids at a concentration above 20 mM, as well as benzyl alcohol and acetophenone at 0.2 mM, severely inhibited electricity generation from glucose using a MFC. Zeng et al. (2016a) reported IC50 (i.e., concentration that results in 50% current production inhibition) values for furfural, 5-hydroxymethyl furfural, syringic acid, vanillic acid, and 4-hydroxybenzoic acid for a bioanode as 2.7, 3.0, 1.9, 2.1, and 2.0 g/L, respectively. It should be pointed out that the reported inhibitory concentrations are specific to bioanode biomass concentration, biofilm thickness, and length of time the bioanode has been exposed to the compounds, and thus may not be generally applicable. Based on the study conducted by Zeng et al. (2016a), the parent compounds, as opposed to their biotransformation products, caused inhibition on exoelectrogenesis, but not on fermentation. Furthermore, a combination of these five compounds at individual, sub-inhibitory concentrations in a mixture resulted in significant, additive but not synergistic inhibition, which warrants future research on mixture effect and reversibility of furanic and phenolic compounds inhibition on bioanode processes.
Conversion of Sugars and Other Fermentable Compounds in Mixed Substrate Streams
Kadier et al., and Pant et al., have previously reviewed substrates used in bioanode for production of electrons (Pant et al., 2010; Kadier et al., 2014). Conversion parameters for the studies reporting high performance in MFC and MECs for key substrates were compiled and are shown in Table 4. We investigate three important issues related to substrate conversion in anode. First, the results obtained with fermentable compounds are compared with that obtained with acetate, the model, non-fermentable substrate for exoelectrogens. Secondly, the impact of inhibitory substrates present in mixed or complex waste streams on conversion of exoelectrogenic substrates, such as acetate as well as other non-inhibitory substrates such as glucose, is included. Thirdly, the simultaneous conversion of fermentable substrates with acetate in the bioanode is discussed. This would indicate production as well as utilization of acetate, as it has been shown to be an end product of fermentation for at least a some of the substrates typically present in biorefinery streams such as sugars and furans (Zeng et al., 2017; Lewis et al., 2018). This analysis allows one to compare the rates and efficiencies reported for real waste streams with those obtained for model systems or single substrates. The operating conditions posed by real waste streams are not always ideal for producing electrons and therefore, these conditions are also included in the table.
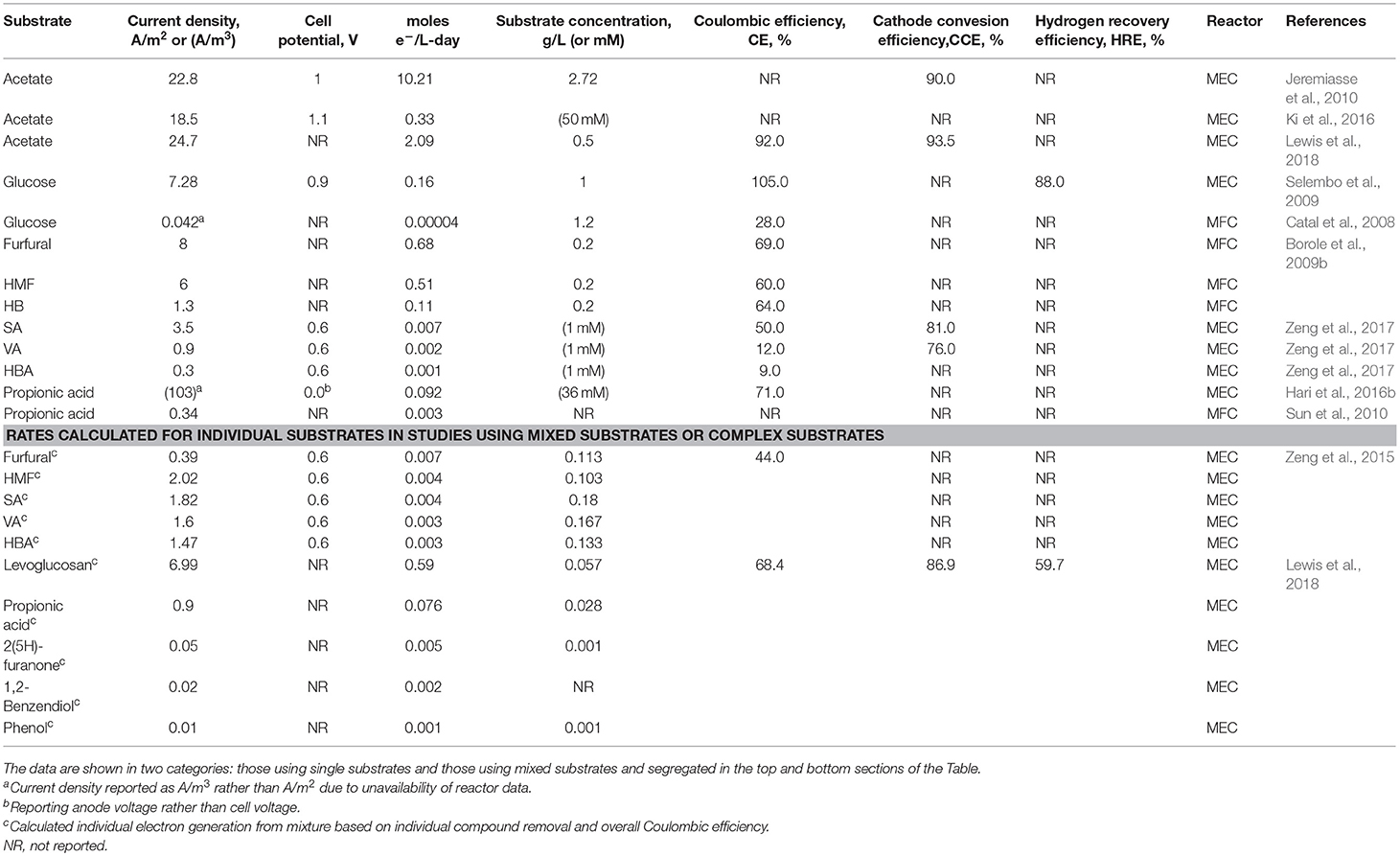
Table 4. Performance of bioelectrochemical systems with various substrates reported in the literature.
The highest hydrogen productivity reported in an MEC to date, has been 50 L/L-day, using acetate as the sole substrate by Jeremiasse et al. (2010). The current density reported in this study was 22 ± 0.1 A/m2. The current density reported by Lewis et al. using acetate as the substrate was 24.7 ± 3.4 A/m2, although the hydrogen productivity was only 27.6 ± 5.39 L/L-day (Lewis et al., 2018). This result was obtained at a substrate concentration of 0.5 gCOD/L and a poised potential of −0.2 V at the anode (equivalent cell voltage = 1.0 V), compared to 2.9 gCOD/Land an applied voltage of 1 0 V) used by Jeremiasse et al. Lewis et al. also reported results with mixed substrate streams in the presence of acetate at a lower concentration of 0.1 g COD/L, which achieved a maximum current density to 8.76 ± 1.54 A/m2 (Table 4). The maximum current density at an acetate concentration of 0.1 gCOD/L, in the absence of other substrates was 11.48 ± 2.94 A/m2. The other substrates included furans, phenols, propionic acid, etc. which were part of the real waste stream, BOAP used in the study. Catal et al. have reported adverse effects of certain furanic and phenolic compounds (2-furaldehyde, benzyl alcohol and acetophenone) on current production (Catal et al., 2008).
Simultaneous conversion of the exoelectrogenic substrates, primarily acetate, and the fermentable and/or inhibitory substrates has also been reported (Lewis et al., 2015, 2018; Zeng et al., 2016a). When BOAP was used as the substrate in MEC, the primary anhydrosugar present, levoglucosan, was reported to be converted simultaneously with acetate and other inhibitory substrates. Levoglucosan was the dominant compound present in the stream, which resulted in a highest rate of conversion among all substrates present in BOAP. Table 4 shows the rate of removal of the major fermentable compounds reported previously. The results are reported in electron equivalents of the substrate converted per liter of reactor per day. This unit is used to allow comparison of the rate of electron generation with the substrate conversion as well as product formation, such as hydrogen in MEC. The typical unit used to represent electron generation, current density is also included in Table 4, to relate the results to the conventional method of reporting. The reason for introduction of the parameters is as follows: In conventional chemical engineering and the chemical industry, the parameter used to represent conversion is moles/L-day. In the BES literature, the substrate and product (for example, carbon source and hydrogen) have been reported in moles/L-day, however, since the substrate is not converted directly into hydrogen, it is necessary to also represent the intermediate, which is electron, in this form. Therefore, the parameter: moles e−/L-day is introduced in this study. This also facilitates understanding of the bioelectrochemical conversion process, since both, substrate and product can be reported in moles e−/L-day. Of the compounds present within BOAP, levoglucosan had the highest reported electron generation rate of 0.59 moles e−/L-day, which is equivalent to 6.99 A/m2. The rate previously reported by Selembo et al. was about three times lower, despite use of a higher substrate concentration. They used glucose as a substrate, which is comparable to levoglucosan, an anhydrous form of the sugar. The rate for levoglucosan is also more than >40x greater than that of furans and phenols within BOAP, and is likely a function of furans and phenols being more recalcitrant but may also be attributed to the relative starting concentrations of different classes of compounds within BOAP, with furans and phenols existing at an order of magnitude or lower compared to levoglucosan and acetic acid. Using furan concentrations two orders of magnitude higher, Borole et al. reached electron generation rates of 0.68 and 0.51 e−/L-day for furfural and HMF (Borole et al., 2009b), similar to that reached for levoglucosan by Lewis et al., indicating initial substrate concentrations may have been a factor (Lewis et al., 2018). However, the use of higher concentrations of parent compounds does not always result in high rates of electron generation. Zeng et al. saw maximum rate of 0.007 e−/L-day for SA as a single substrate and for furfural in a mixture (Zeng et al., 2016a, 2017) with the lower output likely attributed to differences in reactor design, operation and potentially biocatalyst density. Similarly, Hari et al. reached an electron generation rate of 0.092 moles e−/L-day with pure propionic acid. Propionic acid electron generation within BOAP reached a slightly lower level of 0.076 moles e−/L-day despite the relative concentration being two orders of magnitude lower. The data on moles e−/L-day reported in the literature for various compounds is also shown in Figure S5.
Bioelectrochemical Performance Metrics
Conversion of individual substrates present in a mixed feedstock is important to understanding the biochemical processes occurring in the bioanode, however, overall conversion metrics are key to enabling application feasibility of the process. Creating value from waste streams thus requires evaluation of parameters such as overall current density, COD removal, anode efficiency, etc. for the whole stream. Studies reporting use of various complex streams including BOAP, wastewater from fermentation of molasses, cellulose, corn stover, and hydrolyzates from different biomass feedstocks are compiled in Table 5. The current density obtained from complex wastes vary widely ranging from 0.36 to 8.76 A/m2. This indicates a large variability in the performance of BESs, which introduces uncertainty regarding the application of these systems for conversion of real waste streams. The substrate concentration and applied potential are key parameters affecting current density, which are included in the Table 5. The anode Coulombic efficiency also has a large range from 15 to 78%. Similar to the individual substrates, the conversion was evaluated in terms of the electron generate rate for the complex waste streams. Using corn stover fermentation effluent, a rate of 0.49 moles e−/L-day was reached (Borole et al., 2013), which was further improved by Lewis et al. to obtain 0.74 moles e−/L-day for BOAP. Li et al. have the next highest rate for complex stream at 0.31 moles e−/L-day (Lewis et al., 2018). The substrate concentration used was however, 8x higher and a separate fermentation step was used to produce the effluent (Li et al., 2014). Additional studies in the literature without an initial fermentation step have not exceeded a rate over 0.17 moles e−/L-day. There are significant differences in the methodology used by various researchers in the development of the bioanode and process operation while testing the performance of BESs, which leads to differences in performance. Recent reviews have tried to standardize the methods used to develop the bioanode and measurement of performance by defining critical parameters. Since this is a relatively nascent field, new research teams are still entering the area, which will continue to cause variability in results, however, adoption of methods which lead to high performance being reported by reviews and cluster papers will help achieve coherence, leading to confidence in implementation of the technology for real applications. In addition, integration of findings from different subtopics within the BES area, such as mass transfer in anode, charge transfer to cathode, kinetics of cathode catalysts is important to achieve high performance, since the productivity is only as high as the slowest step in the overall process. Thus, leveraging a strategy built on focused biocatalyst development, high-performance reactor design, and optimal operating conditions is key to unlocking the potential of bioelectrochemical systems for conversion of complex waste streams, and developing applications for implementation to advance the bioeconomy.
Cathodic Processes
Conversion of waste streams into value-added products using electrons, also derived from waste can add substantial value to biorefinery operations. Direct conversion of the complex waste streams into products can be nearly impossible, therefore this route via electrons as an intermediate, may yield important benefits. In the subsections below, production of hydrogen, alcohols, diols, and medium chain fatty acids is discussed, which can improve the economics of the biorefinery process.
Production of Hydrogen and Electricity
While electricity is a useful product in the biorefinery, due to availability of alternate methods of generating electricity cheaply, as well as renewably, use of biorefinery waste streams for power generation may not be practiced, even if it is shown to be practically feasible. Therefore, most of the attention is focused on hydrogen and other products, which add greater value to the biorefinery. Hydrogen is needed for upgrading of intermediates produced in biorefinery (Kamm et al., 2008; Borole, 2011; Ferrini and Rinaldi, 2014; Abdullah et al., 2015; Heracleous et al., 2017), production of fuel additives (Gawade et al., 2016) as well as for conversion of non-lignocellulosic biopolymers such as chitin to chemicals (Kobayashi et al., 2017). Current methods of hydrogen production include those from fossil as well as renewable resources (Holladay et al., 2009; Nikolaidis and Poullikkas, 2017). Generating hydrogen from natural gas via reforming is the dominant process today, but it releases high levels of GHGs. The renewable method most advanced today, is water electrolysis. However, it is very expensive, costing over $10/kg-H2 (Personal communication, David Wick, H2 News). The MEC process has potential to be a lower cost solution, due to reduced input of electrical energy, and supplementation by chemical energy from waste. The MEC process has been shown to reach electrical efficiencies of around 150%, even when complex waste and biomass are used as feedstock (Lewis et al., 2015). Thus, it is important to consider the MEC technology as a candidate for investigating renewable hydrogen options.
The productivity of hydrogen and efficiency of production are two main parameters of interest in considering the MEC technology for hydrogen production in the biorefinery. Analysis conducted by Sleutels and others indicates the need to reach a productivity of 20 L/L-day, and a current density of 20 A/m2, to enable economic feasibility (Sleutels et al., 2012; Borole, 2017b). A few studies have reached this goal, primarily using acetate as the substrate, achieved in short term experiments. The hurdles remaining to move this technology toward commercialization include demonstration with real, complex waste streams, scale-up and demonstration of long operational stability. These issues have been investigated by a few investigators (Montpart et al., 2015; Lewis et al., 2018) leading to some improvements in using complex feedstocks (Marcus et al., 2011; Ruiz et al., 2015; Borole and Lewis, 2017), assessment of long term stability (Rago et al., 2015) and initial attempts at scale-up (Cusick et al., 2011; Escapa et al., 2015). A continued effort can potentially enable achievement of the targets for commercialization of the MEC technology in the near future.
Production of Alcohols and Diols
One of the most significant challenges of bio-based refining is economic feasibility of producing biofuels. It has been recognized that production of high value chemicals can alleviate the high costs of processing biomass for biofuel production. Conversion of low value intermediates and waste stream components to chemical building blocks for advanced refining or replacement of petroleum derived intermediates can support the high volume, low value biofuel pathways. Recently, the use of bioelectrochemical routes for production of alcohols and diols has received increasing attention. Production of butanol from glucose, ethanol from acetic acid, 1,3-propanediol from glycerol has been reported in recent years (Moscoviz et al., 2016; Schievano et al., 2016). Pyrolysis-derived aqueous streams already contain a range of alcohols and diols, however, their concentrations are not high enough to allow their separation. The aqueous phase also has many oxygenated compounds, which could serve as substrates for production of alcohols or diols, making it worthwhile to separate specific products or class of compounds, if high titers can be reached. In this section, we discuss bioelectrochemically-controlled processes for production of value-added chemicals, which can improve the economics of the biorefinery, using waste or biomass-derived streams as the feed.
Production of butanol has received much attention in the last decade due to its many advantages as a fuel, however, it is also used as a chemical reagent having an annual demand of 4 million tons. It is used in production of resins, solvents, paints, coatings, perfumes, adhesives, inks, plastics, lubricants, synthetic rubber, etc. (Dürre, 2008). The traditional method of production via acetone-butanol-ethanol fermentation route can be improved via a new approach called electro-fermentation resulting in high titers, enabling easier recovery of the alcohol. Electro-fermentation is a process where the oxidation-reduction potential of a fermentation process is altered extracellularly via exchange of electrons by microbes with an electrode, resulting in a gain or loss of energy. He et al. reported 12.2% higher butanol concentration when an electrical voltage was applied in the presence of the mediator neutral red, during a Clostridium beijerinckii fermentation (He et al., 2016). They measured the concentration of NADH and reported higher levels in presence of mediator and applied electricity. Choi et al. reported increased production of butyric acid using a different Clostridium (Clostridium tyrobutyricum) under the influence of electric potential using methyl viologen as the mediator (Choi et al., 2012). Later, the same group identified electron uptake via direct electron transfer in Clostridium pasteurianum, leading to increased levels of butanol (Choi et al., 2014). Mostafazadeh et al. increased the butanol yield from glucose up to 13.3 g/L using C. pasteurianum at an applied voltage of 1.32 V, a 60% increase over unpoised fermentation (Mostafazadeh et al., 2016). Production of ethanol has also been reported via cathodic reduction of acetate (Steinbusch et al., 2010). In several of these studies, supply of electrons via the cathode is needed to boost the intracellular NADH levels. These electrons can be sourced from waste streams as discussed in previous section. A review paper recently identified potential of this approach for improving industrial fermentations (Schievano et al., 2016).
Besides alcohols, diols have also been produced via the bioelectrochemical approach. Several studies have been reported generation of 1,3-propanediol via a redox-controlled fermentation. Pure as well as mixed cultures have been used to generate 1,3-propanediol from glycerol bioelectrochemically. Clostridium pasteurianum was shown to be capable of reducing glycerol as well as facilitate the electron transfer without a mediator at a potential of 0.045 vs. standard hydrogen electrode (SHE) (Choi et al., 2014). Similar to the studies aimed at butanol production, the NADH/NAD+ levels were shown to be higher during the course of the 1,3-propanediol fermentation. The metabolic pathway and the electron transfer pathway are not always present in same organism, so some studies have used mixed cultures to demonstrate 1,3-propanediol production with success. Optimization of operating conditions is critical for microbiota-based fermentations to achieve the desired product. While redox potential is an important control parameters, the range of potentials under which the fermentation was shown to be possible was quite large, ranging from −0.8 to −1.44 V vs. SHE (Dennis et al., 2013; Zhou et al., 2013, 2015). Further insights are needed to better understand these mixed culture fermentations.
Production of Medium Chain Fatty Acids and Esters
Biorefinery waste streams contain a high proportion of volatile fatty acids and residual sugars or anhydrosugars as a percent of the total organic content. However, these compounds are present at relatively low concentration in the waste streams, therefore their recovery is difficult. Similarly, waste streams from the food and other industries consistently contain similar compounds. Conversion of these compounds to medium chain fatty acids has been reported recently as a means of valorizing these streams (Angenent et al., 2016). This upgrading requires reducing equivalents, which are usually provided in the form of hydrogen or ethanol (Kucek et al., 2016). The use of electrode to deliver the needed reducing equivalents as electrons either directly or via hydrogen has been investigated as a means of electrochemical upgrading (Van Eerten-Jansen et al., 2013). The simultaneous availability of the substrate (volatile fatty acids) and excess electrons derived from waste thus leads to the possibility of production of MCFAs and esters in the biorefinery (Andersen et al., 2014).
Bio-oil aqueous phase contains acetic acid at around 10% of the total organic carbon, thus, its use for production of MCFAs could be a valuable proposition, if separation of the MCFAs can also be achieved simultaneously. This has been shown to be possible using fermentation wastewater (Andersen et al., 2016; Kucek et al., 2016). In addition to production of electrons from the BOAP stream, concentration of the substrates for MCFA production, i.e., acetic acid and other VFAs can also be increased using the same bioanode. Production of acetic as well as propionic acid has been demonstrated in a bioanode under unpoised conditions from BOAP (Lewis et al., 2018), which can be coupled to a biocathode capable of chain elongation leading to formation of MCFAs. These reactors can be self-sufficient to create the necessary VFAs and the reducing equivalents from a single biorefinery waste stream, leading to production of value-added chemicals in the biorefinery.
Electro-Fermentation Process Control
One observation that has been made in fermentations conducted under poised potential conditions is that the electrons needed for the fermentation need not come from the electrode alone. Studies have found that the moles of electrons in the reduced products did not correlate with the current flowing from the cathode. Instead, large fraction of the electrons actually were derived from the substrate itself. The difference in electron balance vs. the unpoised control was a shift in the metabolism toward reduced products vs. a balanced product slate observed in typical fermentations. The poised potential apparently results in a change in the intracellular NADH /NAD+ levels, and directs the electrons toward the reduced product. Moscoviz et al. reported a new parameter called electro-fermentation coefficient (ηEF), which is the ratio of the electrons donated by the electrode vs. those present in the product, to quantify the altered electron stoichiometry (Moscoviz et al., 2016). If the productivity of the desired product is decoupled from the rate of electron flow from the electrode, i.e., the current density, this could mean a significant advancement and a diversion from typical bioelectrochemical processing. The number of studies to date, however, have been limited, thus more work is needed to understand the electro-fermentation process completely. An additional question that arises in context of the electrochemical control is the quantification of the productivities with respect to the redox potential. If current is not a determinant of the rate of production of the reduced products, what is the relationship between applied potential and the productivity or the product distribution? It is possible that the current density may still influence the product titer and productivity, increasing the yield and productivity beyond that possible via the use of the primary substrate alone. The supply of waste-derived electrons may, therefore, still be important and improve the performance of the electro-fermentation systems beyond that possible with redox control alone. Additionally, the use of waste streams as a feed to cathode itself during electro-fermentation may lead to formation of new chemicals, for example from furanic and phenolic compounds. This is a completely new area for development of novel products and exploratory research in this area may be quite productive. Thus, the impact of electro-fermentation approach can be quite significant in production of reduced products such as biofuels and biochemicals in the biorefinery, while reducing waste disposal costs.
Path Forward
Conversion of complex wastes such as BOAP into value-added products is possible via integrated separation-conversion processes. Due to the vast range of compounds present in BOAP, separation processes have limitations in yielding purified compounds, often resulting in mixtures of compounds of similar or related groups. Production of high value, pure compounds is however, possible, via bioelectrochemical approach which generates chemicals through the channel of electrons. The sections above elaborated the various components of such a process illustrating the feasibility of the anodic and cathodic conversions. In order to advance this path further into a real application, developments are needed in biocatalyst optimization to balance the rate of fermentation and electrogenesis (Lewis et al., 2018). While high current densities were obtained using BOAP, sustained production of electrons will be key for stable long term operation. Additionally, improvements in reactor design are needed to alleviate charge transfer limitations experienced during the balance of electrochemical reactions in the anode and cathode. In the simple case of hydrogen production in an MEC from BOAP, it was reported that proton transfer can be the limiting factor when high electron generation rates are achieved in the anode (Borole and Lewis, 2017). Since the anode process is the same for production of other chemicals at the cathode, finding a solution to the proton transfer issue will be key in advancing these processes as well. Alternate configurations of membranes or separators between anode and cathode are needed to facilitate proton transfer, while keeping costs low. Thus, continued improvements in the anode kinetics as well as materials and reactor design will be necessary to realize the path to commercialization.
Conclusions
Bio-oil generated via pyrolysis can form two phases, one containing hydrophilic compounds, and the other containing hydrophobic compounds. The aqueous component of bio-oil is a complex stream constituting a large fraction of the product. It is usually treated as a waste stream, which can represent a loss of carbon and energy. Thus, valorization of the BOAP is important. The complexity of the aqueous phase makes it very challenging to separate the components into individual, pure compounds. Solvent extraction, as well as chemical manipulation, can be employed to separate the various compounds into useful groups of chemicals. A two-pronged approach consisting of separation schemes coupled with bioelectrochemical conversion is presented to generate value from the waste stream. Utilization of the remaining mixture after separation of possible fractions to produce valuable chemicals or products is discussed. The bioelectrochemical route transforms the complex mixture into electrons, which are then used to generate value-added products via cathodic reactions. The anode uses microbial consortia to break down the complex mixture producing electrons, while the cathode builds chemical molecules using anode-generated electrons via electrochemical catalysis. Deconstruction of the BOAP requires ability to manage inhibitory effects of furanic and phenolic compounds present in the bio-oil. The open literature is reviewed to integrate studies from multiple disciplines including biology, electrochemistry, and engineering with the objective to develop efficient and productive systems to generate value from waste. Diverse, electroactive microbial catalysts capable of producing current densities above 10 A/m2 are identified to enable application feasibility; however, further developments in reactor design and materials are necessary to move this technology toward commercial consideration. The cathodic processes capable of generating electricity, hydrogen, alcohols, diols, medium chain fatty acids, etc. are documented to illustrate potential of the bioelectrochemical platform for improving biorefinery economics. Generation of these products from thermochemical, as well as biochemical, process wastes in biorefineries makes this platform versatile, allowing development of new routes for supporting a circular economy, essential for the twenty-first century.
Author Contributions
AB created an outline of the manuscript and coordinated content with all co-authors. He wrote the main body of the section Introduction, Bio-oil Components and Carbon in Aqueous Waste, Path Forward, and part of section Bioelectrochemical Platform Converting Waste to Electrons to Products. CT, SY, and LP contributed to the section on separations. AL compiled the data for tables related to bioelectrochemical conversion and wrote the related text. SP and XZ wrote the sections on the anodic bioconversion and inhibitory effect of model furanic and phenolic compounds.
Conflict of Interest Statement
The authors declare that the research was conducted in the absence of any commercial or financial relationships that could be construed as a potential conflict of interest.
Acknowledgments
This manuscript has been authored by UT-Battelle, LLC under Contract No. DE-AC05-00OR22725 with the U.S. Department of Energy (DOE). This article is based upon work supported by the Bioenergy Technologies Office (BETO) within the DOE Office of Energy Efficiency and Renewable Energy (EERE) under the Carbon, Hydrogen, and Separations Efficiency for Bio-oil Pathways (CHASE) program. Funding from the Oak Ridge National Laboratory Seed Money Program is also acknowledged. AL was partially supported by the Bredesen Center for Interdisciplinary Research and Education, the University of Tennessee, Knoxville.
Supplementary Material
The Supplementary Material for this article can be found online at: https://www.frontiersin.org/articles/10.3389/fenrg.2018.00094/full#supplementary-material
References
Abdullah, Z., Chadwell, B., Taha, R., Hindin, B., and Ralston, K. (2015). Upgrading of Bio-Oil Produced by Pyrolysis. Battelle Memorial Institute, Report DE-EE0004391, Columbus, OH.
Andersen, S. J., Berton, J. K., Naert, P., Gildemyn, S., Rabaey, K., and Stevens, C. V. (2016). Extraction and esterification of low-titer short-chain volatile fatty acids from anaerobic fermentation with ionic liquids. ChemSusChem 9, 2059–2063. doi: 10.1002/cssc.201600473
Andersen, S. J., Hennebel, T., Gildemyn, S., Coma, M., Desloover, J., Berton, J., et al. (2014). Electrolytic membrane extraction enables production of fine chemicals from biorefinery sidestreams. Environ. Sci. Technol. 48, 7135–7142. doi: 10.1021/es500483w
Angenent, L. T., Richter, H., Buckel, W., Spirito, C. M., Steinbusch, K. J., Plugge, C. M., et al. (2016). Chain elongation with reactor microbiomes: open-culture biotechnology to produce biochemicals. Environ. Sci. Technol. 50, 2796–2810. doi: 10.1021/acs.est.5b04847
ARPA-E (2014). Electrofuels. Available online at: https://arpa-e.energy.gov/?q=arpa-e-programs/electrofuels
Bache, R., and Pfennig, N. (1981). Selective isolation of Acetobacterium woodii on methoxylated aromatic acids and determination of growth yields. Arch. Microbiol. 130, 255–261. doi: 10.1007/BF00459530
Borole, A. (2015). “Renewable hydrogen production from biomass pyrolysis aqueous phase,” in BETO Annual Review, Vol. CHASE Project Review (Alexandria, VA: DOE EERE). Available online at: https://cbes.ornl.gov/files/2017/12/WorkShop2015BorolePres.pdf
Borole, A. P. (2011). Improving energy efficiency and enabling water recycle in biorefineries using bioelectrochemical cells. Biofuels Bioprod. Biorefin. 5, 28–36. doi: 10.1002/bbb.265
Borole, A. P. (2015). Microbial fuel cells and microbial electrolyzers. Electrochem. Soc. Interface 24, 55–59. doi: 10.1149/2.F02153if
Borole, A. P. (2016). “Microbial electrochemical cells and biorefinery energy efficiency,” in Biotechnology for Biofuel Production and Optimization, 1st Edn, eds T. Cong, C. Eckert (New York, NY: Elsevier), 449–472. doi: 10.1016/B978-0-444-63475-7.00017-0
Borole, A. P. (2017a). “Bioelectrochemical biorefining, a chapter in the book,” in Bioelectrochemical Biorefining, a Chapter in the Book: Biofuels and Bioenergy, ed O. Konur (Boca Raton, FL: CRC Press), 435–462.
Borole, A. P. (2017b). “Renewable hydrogen production from biomass pyrolysis aqueous phase,” in BETO Annual Review, Vol. CHASE Project Review (Denver, CO: DOE EERE). Available online at: https://energy.gov/sites/prod/files/2017/05/f34/thermochem_borole_2.5.5.403.pdf
Borole, A. P., Hamilton, C., and Schell, D. (2013). Conversion of residual organics in corn stover-derived biorefinery stream to bioenergy via microbial fuel cells. Environ. Sci. Technol. 47, 642–648. doi: 10.1021/es3023495
Borole, A. P., Hamilton, C. Y., Vishnivetskaya, T. A., Leak, D., and Andras, C. (2009a). Improving power production from acetate-fed microbial fuel cells via enrichment of exoelectrogenic organisms in continuous flow systems. Biochem. Eng. J. 48, 71–80.
Borole, A. P., and Lewis, A. J. (2017). Proton transfer in microbial electrolysis cells. Sust. Energy Fuels 1, 725–736. doi: 10.1039/C7SE00034K
Borole, A. P., Mielenz, J., Vishnivetskaya, T. A., and Hamilton, C. Y. (2009b). Controlling accumulation of fermentation inhibitors in biorefinery water recycle using microbial fuel cells. Biotechnol. Biofuels 2:7. doi: 10.1186/1754-6834-2-7
Brune, A., and Schink, B. (1992). Phloroglucinol pathway in the strictly anaerobic Pelobacter acidigallici: fermentation of trihydroxybenzenes to acetate via triacetic acid. Archiv. Microb. 157, 417–424. doi: 10.1007/BF00249098
Campanario, F., and Ortiz, F. G. (2017). Fischer-tropsch biofuels production from syngas obtained by supercritical water reforming of the bio-oil aqueous phase. Energy Conv. Manage. 150, 599–613. doi: 10.1016/j.enconman.2017.08.053
Catal, T., Fan, Y. Z., Li, K. C., Bermek, H., and Liu, H. (2008). Effects of furan derivatives and phenolic compounds on electricity generation in microbial fuel cells. J. Power Sour. 180, 162–166. doi: 10.1016/j.jpowsour.2008.02.052
Chan, J. K., and Duff, S. J. (2010). Methods for mitigation of bio-oil extract toxicity. Bioresour. Technol. 101, 3755–3759. doi: 10.1016/j.biortech.2009.12.054
Choi, O., Kim, T., Woo, H. M., and Um, Y. (2014). Electricity-driven metabolic shift through direct electron uptake by electroactive heterotroph Clostridium pasteurianum. Sci. Rep. 4:6961. doi: 10.1038/srep06961
Choi, O., Um, Y., and Sang, B. I. (2012). Butyrate production enhancement by Clostridium tyrobutyricum using electron mediators and a cathodic electron donor. BioTechnol. Bioengineer. 109, 2494–2502. doi: 10.1002/bit.24520
Cusick, R. D., Bryan, B., Parker, D. S., Merrill, M. D., Mehanna, M., Kiely, P. D., et al. (2011). Performance of a pilot-scale continuous flow microbial electrolysis cell fed winery wastewater. Appl. Microb. BioTechnol. 89, 2053–2063. doi: 10.1007/s00253-011-3130-9
Dennis, P. G., Harnisch, F., Yeoh, Y. K., Tyson, G. W., and Rabaey, K. (2013). Dynamics of cathode-associated microbial communities and metabolite profiles in a glycerol-fed bioelectrochemical system. Appl. Env. Microb. 79, 4008–4014. doi: 10.1128/AEM.00569-13
Desloover, J., Arends, J. B., Hennebel, T., and Rabaey, K. (2012). Operational and technical considerations for microbial electrosynthesis. Biochem. Soc. Transac. 40, 1233–1238. doi: 10.1042/BST20120111
Dürre, P. (2008). Fermentative butanol production. Ann. N. Y. Acad. Sci. 1125, 353–362. doi: 10.1196/annals.1419.009
Elkasabi, Y., Mullen, C. A., and Boateng, A. A. (2015). Aqueous extractive upgrading of bio-oils created by tail-gas reactive pyrolysis to produce pure hydrocarbons and phenols. ACS Sust. Chem. Engineer. 3, 2809–2816. doi: 10.1021/acssuschemeng.5b00730
Elliott, D. C., Hart, T. R., Neuenschwander, G. G., Rotness, L. J., and Zacher, A. H. (2009). Catalytic hydroprocessing of biomass fast pyrolysis bio-oil to produce hydrocarbon products. Environ. Progr. Sust. Energy 28, 441–449. doi: 10.1002/ep.10384
Escapa, A., San-Martín, M. I., Mateos, R., and Moran, A. (2015). Scaling-up of membraneless microbial electrolysis cells (MECs) for domestic wastewater treatment: Bottlenecks and limitations. Bioresour. Technol. 180, 72–78. doi: 10.1016/j.biortech.2014.12.096
Ferrini, P., and Rinaldi, R. (2014). Catalytic biorefining of plant biomass to non-pyrolytic lignin bio-oil and carbohydrates through hydrogen transfer reactions. Angew. Chem. Int. Edn. 53, 8634–8639. doi: 10.1002/anie.201403747
Freguia, S., Rabaey, K., Yuan, Z. G., and Keller, J. (2007). Electron and carbon balances in microbial fuel cells reveal temporary bacterial storage behavior during electricity generation. Environ. Sci. Technol. 41, 2915–2921. doi: 10.1021/es062611i
Freguia, S., Rabaey, K., Yuan, Z. G., and Keller, J. (2008). Syntrophic processes drive the conversion of glucose in microbial fuel cell anodes. Environ. Sci. Technol. 42, 7937–7943. doi: 10.1021/es800482e
Friman, H., Schechter, A., Ioffe, Y., Nitzan, Y., and Cahan, R. (2013). Current production in a microbial fuel cell using a pure culture of Cupriavidus basilensis growing in acetate or phenol as a carbon source. Microb. Biotechnol. 6, 425–434. doi: 10.1111/1751-7915.12026
Fu, J., Hakim, S. H., and Shanks, B. H. (2012). Aqueous-phase processing of bio-oil model compounds over Pt-Re supported on carbon. Top. Cataly. 55, 140–147. doi: 10.1007/s11244-012-9784-4
Gawade, A. B., Tiwari, M. S., and Yadav, G. D. (2016). Biobased green process: selective hydrogenation of 5-hydroxymethylfurfural to 2, 5-dimethyl furan under mild conditions using Pd-Cs2. 5H0. 5PW12O40/K-10 clay. ACS Sust. Chem. Engineer. 4, 4113–4123. doi: 10.1021/acssuschemeng.6b00426
Ginige, M. P., Keller, J., and Blackall, L. L. (2005). Investigation of an acetate-fed denitrifying microbial community by stable isotope probing, full-cycle rRNA analysis, and fluorescent in situ hybridization-microautoradiography. Appl. Environ. Microbiol. 71, 8683–8691. doi: 10.1128/AEM.71.12.8683-8691.2005
Hari, A. R., Katuri, K. P., Gorron, E., Logan, B. E., and Saikaly, P. E. (2016a). Multiple paths of electron flow to current in microbial electrolysis cells fed with low and high concentrations of propionate. Appl. Microbiol. BioTechnol. 100, 5999–6011. doi: 10.1007/s00253-016-7402-2
Hari, A. R., Katuri, K. P., Logan, B. E., and Saikaly, P. E. (2016b). Set anode potentials affect the electron fluxes and microbial community structure in propionate-fed microbial electrolysis cells. Sci. Rep. 6:38690. doi: 10.1038/srep38690
He, A.-Y., Yin, C.-Y., Xu, H., Kong, X.-P., Xue, J.-W., Zhu, J., et al. (2016). Enhanced butanol production in a microbial electrolysis cell by Clostridium beijerinckii IB4. Bioproc. Biosyst. Engineer. 39, 245–254. doi: 10.1007/s00449-015-1508-2
He, P., and Song, H. (2014). Catalytic conversion of biomass by natural gas for oil quality upgrading. Indust. Engineer. Chem. Res. 53, 15862–15870. doi: 10.1021/ie502272j
Hedbavna, P., Rolfe, S. A., Huang, W. E., and Thornton, S. F. (2016). Biodegradation of phenolic compounds and their metabolites in contaminated groundwater using microbial fuel cells. Bioresour. Technol. 200, 426–434. doi: 10.1016/j.biortech.2015.09.092
Heracleous, E., Lappas, A., and Serrano, D. (2017). Special thematic issue in “Biomass Conversion and Biorefinery” “Advances in catalytic biomass fast pyrolysis and bio-oil upgrading”. Biomass Convers. Biorefinery 7, 275–276. doi: 10.1007/s13399-017-0284-4
Hesselsoe, M., Füreder, S., Schloter, M., Bodrossy, L., Iversen, N., Roslev, P., et al. (2009). Isotope array analysis of Rhodocyclales uncovers functional redundancy and versatility in an activated sludge. ISME J. 3, 1349–1364. doi: 10.1038/ismej.2009.78
Holladay, J. D., Hu, J., King, D. L., and Wang, Y. (2009). An overview of hydrogen production technologies. Cataly. Today 139, 244–260. doi: 10.1016/j.cattod.2008.08.039
Huang, D.-Y., Zhou, S.-G., Chen, Q., Zhao, B., Yuan, Y., and Zhuang, L. (2011). Enhanced anaerobic degradation of organic pollutants in a soil microbial fuel cell. Chem. Engin. J. 172, 647–653. doi: 10.1016/j.cej.2011.06.024
Huber, G. W., Cortright, R. D., and Dumesic, J. A. (2004). Renewable alkanes by aqueous-phase reforming of biomass-derived oxygenates. Angew. Chem. Int. Edn. 43, 1549–1551. doi: 10.1002/anie.200353050
Humbird, D., David, R., Tao, L., Kinchin, C., Hsu, D., Aden, A., et al. (2011). Process Design and Economics for Biochemical Conversion of Lignocellulosic Biomass to Ethanol - Dilute-Acid Pretreatment and Enzymatic Hydrolysis of Corn Stover. National Renewable Energy Laboratory (NREL). NREL/TP-5100-47764
Ishii, S. I., Suzuki, S., Norden-Krichmar, T. M., Phan, T., Wanger, G., Nealson, K. H., et al. (2014). Microbial population and functional dynamics associated with surface potential and carbon metabolism. ISME J. 8, 963–978. doi: 10.1038/ismej.2013.217
Jacobson, K., Maheria, K. C., and Dalai, A. K. (2013). Bio-oil valorization: a review. Renew. Sust. Energy Rev. 23, 91–106. doi: 10.1016/j.rser.2013.02.036
Jeremiasse, A. W., Hamelers, H. V. M., Saakes, M., and Buisman, C. J. N. (2010). Ni foam cathode enables high volumetric H-2 production in a microbial electrolysis cell. Int. J. Hydrogen Energy 35, 12716–12723. doi: 10.1016/j.ijhydene.2010.08.131
Jones, S., Meyer, P., Snowden-Swan, L., Padmaperuma, A., Tan, E., Dutta, A., et al. (2013). Process Design and Economics for Conversion of Lignocellulosic Biomass to Hydrocarbon Fuels. PNNL. PNNL-23053, NREL/TP-5100-61178.
Jones, S., Valkenburg, C., Walton, C., Elliot, D., Holladay, J., STevens, D., et al. (2009). Production of Gasoline and Diesel from Biomass via Fast Pyrolysis, Hydrotreating and Hydrocracking: A Design Case. Pacific Northwest National Laboratory. ONNL-18284 Rev.1.
Jung, S., and Regan, J. M. (2007). Comparison of anode bacterial communities and performance in microbial fuel cells with different electron donors. Appl. Microbiol. Biotechnol. 77, 393–402. doi: 10.1007/s00253-007-1162-y
Kadier, A., Simayi, Y., Kalil, M. S., Abdeshahian, P., and Hamid, A. A. (2014). A review of the substrates used in microbial electrolysis cells (MECs) for producing sustainable and clean hydrogen gas. Renew. Energy 71, 466–472. doi: 10.1016/j.renene.2014.05.052
Kamm, B., Gruber, P. R., and Kamm, M. (2008). Biorefineries-Industrial Processes and Products. Weinheim: Wiley InterScience.
Kechagiopoulos, P. N., Voutetakis, S. S., Lemonidou, A. A., and Vasalos, I. A. (2006). Hydrogen production via steam reforming of the aqueous phase of bio-oil in a fixed bed reactor. Energy Fuels 20, 2155–2163. doi: 10.1021/ef060083q
Ki, D., Popat, S. C., and Torres, C. I. (2016). Reduced overpotentials in microbial electrolysis cells through improved design, operation, and electrochemical characterization. Chem. Engineer. J. 287, 181–188. doi: 10.1016/j.cej.2015.11.022
Kiely, P. D., Regan, J. M., and Logan, B. E. (2011). The electric picnic: synergistic requirements for exoelectrogenic microbial communities. Curr. Opin. BioTechnol. 22, 378–385. doi: 10.1016/j.copbio.2011.03.003
Kim, Y.-H., Park, L. K., Yiacoumi, S., and Tsouris, C. (2017). Modular chemical process intensification: a review. Ann. Rev. Chem. Biomol. Engineer. 8, 359–380. doi: 10.1146/annurev-chembioeng-060816-101354
Klinke, H. B., Thomsen, A. B., and Ahring, B. K. (2004). Inhibition of ethanol-producing yeast and bacteria by degradation products produced during pre-treatment of biomass. Appl. Microbiol. BioTechnol. 66, 10–26. doi: 10.1007/s00253-004-1642-2
Kobayashi, H., Techikawara, K., and Fukuoka, A. (2017). Hydrolytic hydrogenation of chitin to amino sugar alcohol. Green Chem. 19, 3350–3356. doi: 10.1039/C7GC01063J
Kucek, L. A., Spirito, C. M., and Angenent, L. T. (2016). High n-caprylate productivities and specificities from dilute ethanol and acetate: chain elongation with microbiomes to upgrade products from syngas fermentation. Energy Environ. Sci. 9, 3482–3494. doi: 10.1039/C6EE01487A
Lalaurette, E., Thammannagowda, S., Mohagheghi, A., Maness, P. C., and Logan, B. E. (2009). Hydrogen production from cellulose in a two-stage process combining fermentation and electrohydrogenesis. Int. J. Hydrogen Energy 34, 6201–6210. doi: 10.1016/j.ijhydene.2009.05.112
Lewis, A. J., and Borole, A. P. (2017). “Bio-electro-refinery: conversion, sustainability and policy,” in Microbial Electrochemical Technology: Platform for Fuels, Chemicals and Remediation, eds S. Venkata Mohan, S. Varjani, A. Pandey (Amsterdam: Elsevier).
Lewis, A. J., Campa, M., Hazen, T. C., and Borole, A. P. (2018). Unravelling biocomplexity of electroactive biofilms for producing hydrogen from biomass. Microb. BioTechnol. 11, 84–97. doi: 10.1111/1751-7915.12756
Lewis, A. J., Ren, S., Ye, X., Kim, P., Labbe, N., and Borole, A. P. (2015). Hydrogen production from switchgrass via a hybrid pyrolysis-microbial electrolysis process. Bior. Technol. 195, 231–241. doi: 10.1016/j.biortech.2015.06.085
Li, X.-H., Liang, D.-W., Bai, Y.-X., Fan, Y.-T., and Hou, H.-W. (2014). Enhanced H-2 production from corn stalk by integrating dark fermentation and single chamber microbial electrolysis cells with double anode arrangement. Int. J. Hydrogen Energy 39, 8977–8982. doi: 10.1016/j.ijhydene.2014.03.065
Liu, H., Grot, S., and Logan, B. E. (2005). Electrochemically assisted microbial production of hydrogen from acetate. Environ. Sci. Technol. 39, 4317–4320. doi: 10.1021/es050244p
Liu, H., Hu, H., Chignell, J., and Fan, Y. (2010). Microbial electrolysis: novel technology for hydrogenb production from biomass. Biofuels 1, 129–142. doi: 10.4155/bfs.09.9
Logan, B. E., Call, D., Cheng, S., Hamelers, H. V. M., Sleutels, T., Jeremiasse, A. W., et al. (2008). Microbial electrolysis cells for high yield hydrogen gas production from organic matter. Environ. Sci. Technol. 42, 8630–8640. doi: 10.1021/es801553z
Logan, B. E., and Rabaey, K. (2012). Conversion of wastes into bioelectricity and chemicals by using microbial electrochemical technologies. Science 337, 686–690. doi: 10.1126/science.1217412
Logan, B. E., and Regan, J. M. (2006). Microbial fuel cells, challenges and applications. Environ. Sci. Technol. 40, 5172–5180. doi: 10.1021/es0627592
Lu, L., Ren, N. Q., Xing, D. F., and Logan, B. E. (2009). Hydrogen production with effluent from an ethanol-H-2-coproducing fermentation reactor using a single-chamber microbial electrolysis cell. Biosens. Bioelectron. 24, 3055–3060. doi: 10.1016/j.bios.2009.03.024
Lu, L., and Ren, Z. J. (2016). Microbial electrolysis cells for waste biorefinery: a state of the art review. Bioresour. Technol. 215, 254–264. doi: 10.1016/j.biortech.2016.03.034
Luo, H., Liu, G., Zhang, R., and Jin, S. (2009). Phenol degradation in microbial fuel cells. Chem. Engineer. J. 147, 259–264. doi: 10.1016/j.cej.2008.07.011
Ma, B., and Agblevor, F. A. (2014). Polarity-based separation and chemical characterization of fast pyrolysis bio-oil from poultry litter. Biomass Bioenergy 64, 337–347. doi: 10.1016/j.biombioe.2014.03.003
Mahmoud, M., Parameswaran, P., Torres, C. I., and Rittmann, B. E. (2016). Relieving the fermentation inhibition enables high electron recovery from landfill leachate in a microbial electrolysis cell. RSC Adv. 6, 6658–6664. doi: 10.1039/C5RA25918E
Marcus, A. K., Torres, C. I., and Rittmann, B. E. (2011). Analysis of a microbial electrochemical cell using the proton condition in biofilm (PCBIOFILM) model. Bioresource Technol. 102, 253–262. doi: 10.1016/j.biortech.2010.03.100
Marone, A., Ayala-Campos, O. R., Trably, E., Carmona-Martinez, A. A., Moscoviz, R., Latrille, E., et al. (2017). Coupling dark fermentation and microbial electrolysis to enhance bio-hydrogen production from agro-industrial wastewaters and by-products in a bio-refinery framework. Int. J. Hydrogen Energy 42, 1609–1621. doi: 10.1016/j.ijhydene.2016.09.166
Marone, A., Carmona-Martínez, A. A., Sire, Y., Meudec, E., Steyer, J. P., Bernet, N., et al. (2016). Bioelectrochemical treatment of table olive brine processing wastewater for biogas production and phenolic compounds removal. Water Res. 100, 316–325. doi: 10.1016/j.watres.2016.05.008
McFarlane, J., Tsouris, C., Birdwell, J. F. Jr., Schuh, D. L., Jennings, H. L., Palmer Boitrago, A. M., et al. (2010). Production of biodiesel at the kinetic limit in a centrifugal reactor/separator. Indust. Engineer. Chem. Res. 49, 3160–3169. doi: 10.1021/ie901229x
Mills, T. Y., Sandoval, N. R., and Gill, R. T. (2009). Cellulosic hydrolysate toxicity and tolerance mechanisms in Escherichia coli. BioTechnol. Biofuels 2, 1–11. doi: 10.1186/1754-6834-2-26
Monlau, F., Sambusiti, C., Barakat, A., Quéméneur, M., Trably, E., Steyer, J. P., et al. (2014). Do furanic and phenolic compounds of lignocellulosic and algae biomass hydrolyzate inhibit anaerobic mixed cultures? A comprehensive review. BioTechnol. Adv. 32, 934–951. doi: 10.1016/j.biotechadv.2014.04.007
Montpart, N., Rago, L., Baeza, J. A., and Guisasola, A. (2015). Hydrogen production in single chamber microbial electrolysis cells with different complex substrates. Water Res. 68, 601–615. doi: 10.1016/j.watres.2014.10.026
Moscoviz, R., Toledo-Alarcón, J., Trably, E., and Bernet, N. (2016). Electro-fermentation: how to drive fermentation using electrochemical systems. Trends BioTechnol. 34, 856–865. doi: 10.1016/j.tibtech.2016.04.009
Mostafazadeh, A. K., Drogui, P., Brar, S. K., Tyagi, R. D., Le Bihan, Y., Buelna, G., et al. (2016). Enhancement of biobutanol production by electromicrobial glucose conversion in a dual chamber fermentation cell using C. pasteurianum. Energy Conv. Manage. 130, 165–175. doi: 10.1016/j.enconman.2016.10.050
Mukarakate, C., Evans, R. J., Deutch, S., Evans, T., Starace, A. K., ten Dam, J., et al. (2017). Reforming biomass derived pyrolysis bio-oil aqueous phase to fuels. Energy Fuels 31, 1600–1607. doi: 10.1021/acs.energyfuels.6b02463
Mullen, C. A., Boateng, A. A., and Goldberg, N. M. (2013). Production of deoxygenated biomass fast pyrolysis oils via product gas recycling. Energy Fuels 27, 3867–3874. doi: 10.1021/ef400739u
Nevin, K. P., Hensley, S. A., Franks, A. E., Summers, Z. M., Ou, J. H., Woodard, T. L., et al. (2011). Electrosynthesis of organic compounds from carbon dioxide is catalyzed by a diversity of acetogenic microorganisms. Appl. Environ. Microbiol. 77, 2882–2886. doi: 10.1128/AEM.02642-10
Nikolaidis, P., and Poullikkas, A. (2017). A comparative overview of hydrogen production processes. Renew. Sust. Energy Rev. 67, 597–611. doi: 10.1016/j.rser.2016.09.044
Oren, A. (2014). “The family rhodocyclaceae,” in The Prokaryotes: Alphaproteobacteria and Betaproteobacteria, eds E. F. DeLong, S. Lory, E. Stackebrandt, and F. L. Thompson (Berlin, Heidelberg: Springer).
Paasikallio, V., Kihlman, J., Sánchez, C. A. S., Simell, P., Solantausta, Y., and Lehtonen, J. (2015). Steam reforming of pyrolysis oil aqueous fraction obtained by one-step fractional condensation. Int. J. Hydrogen Energy 40, 3149–3157. doi: 10.1016/j.ijhydene.2015.01.025
Pant, D., Van Bogaert, G., Diels, L., and Vanbroekhoven, K. (2010). A review of the substrates used in microbial fuel cells (MFCs) for sustainable energy production. Bioresour. Technol. 101, 1533–1543. doi: 10.1016/j.biortech.2009.10.017
Parameswaran, P., Torres, C. I., Lee, H. S., Krajmalnik-Brown, R., and Rittmann, B. E. (2009). Syntrophic interactions among anode respiring bacteria (ARB) and Non-ARB in a biofilm anode: electron balances. BioTechnol. Bioengineer. 103, 513–523. doi: 10.1002/bit.22267
Parameswaran, P., Zhang, H. S., Torres, C. I., Rittmann, B. E., and Krajmalnik-Brown, R. (2010). Microbial community structure in a biofilm anode fed with a fermentable substrate: the significance of hydrogen scavengers. BioTechnol. Bioengineer. 105, 69–78. doi: 10.1002/bit.22508
Park, L. K., Ren, S., Yiacoumi, S., Ye, P., Borole, A. P., and Tsouris, C. (2016). Separation of switchgrass bio-oil by water/organic solvent addition and pH adjustment. Energy Fuels 30, 2164–2173. doi: 10.1021/acs.energyfuels.5b02537
Park, L. K.-E., Ren, S., Yiacoumi, S., Ye, X. P., Borole, A. P., and Tsouris, C. (2017). pH neutralization of aqueous bio-oil from switchgrass intermediate pyrolysis using process intensification devices. Energy Fuels 31, 9455–9464. doi: 10.1021/acs.energyfuels.7b00854
Park, L. K.-E., Satinover, S. J., Yiacoumi, S., Mayes, R. T., Borole, A. P., and Tsouris, C. (2018). Electrosorption of organic acids from aqueous bio-oil and conversion into hydrogen via microbial electrolysis cells. Renew. Energy 125, 21–31. doi: 10.1016/j.renene.2018.02.076
Pirwitz, K., Rihko-Struckmann, L., and Sundmacher, K. (2016). Valorization of the aqueous phase obtained from hydrothermally treated Dunaliella salina remnant biomass. Bioresour. Technol. 219, 64–71. doi: 10.1016/j.biortech.2016.06.095
Rabaey, K., Girguis, P., and Nielsen, L. K. (2011). Metabolic and practical considerations on microbial electrosynthesis. Curr. Opin. BioTechnol. 22, 371–377. doi: 10.1016/j.copbio.2011.01.010
Rabaey, K., and Rozendal, R. (2010). Microbial electrosynthesis - revisiting the electrical route for microbial production. Nat. Rev. Microbiol. 8, 706–716. doi: 10.1038/nrmicro2422
Rago, L., Ruiz, Y., Baeza, J. A., Guisasola, A., and Cortes, P. (2015). Microbial community analysis in a long-term membrane-less microbial electrolysis cell with hydrogen and methane production. Bioelectrochemistry 106, 359–368. doi: 10.1016/j.bioelechem.2015.06.003
Rasmussen, H., Sørensen, H. R., and Meyer, A. S. (2014). Formation of degradation compounds from lignocellulosic biomass in the biorefinery: sugar reaction mechanisms. Carbohyd. Res. 385, 45–57. doi: 10.1016/j.carres.2013.08.029
Rasrendra, C., Girisuta, B., Van de Bovenkamp, H., Winkelman, J., Leijenhorst, E., Venderbosch, R., et al. (2011). Recovery of acetic acid from an aqueous pyrolysis oil phase by reactive extraction using tri-n-octylamine. Chem. Engineer. J. 176, 244–252. doi: 10.1016/j.cej.2011.08.082
Ren, S., Ye, P., Borole, A. P., Kim, P., and Labbe, N. (2016). Analysis of switchgrass-derived bio-oil and associated aqueous phase generated in a pilot-scale auger pyrolyzer. J. Appl. and Anal. Pyrolys. 119, 97–103. doi: 10.1016/j.jaap.2016.03.013
Ren, S., Ye, X. P., and Borole, A. P. (2017). Separation of chemical groups from bio-oil water-extract via sequential organic solvent extraction. J. Analy. Appl. Pyrolys. 123, 30–39. doi: 10.1016/j.jaap.2017.01.004
Rismani-Yazdi, H., Christy, A. D., Dehority, B. A., Morrison, M., Yu, Z., and Tuovinen, O. H. (2007). Electricity generation from cellulose by rumen microorganisms in microbial fuel cells. Biotechnol Bioeng, 97, 1398–1407. doi: 10.1002/bit.21366
Rittmann, B. E., and McCarty, P. L. (2001). Environmental Biotechnology: Principles and Applications. New York, NY: McGraw-Hill.
Ruiz, Y., Baeza, J. A., and Guisasola, A. (2015). Enhanced performance of bioelectrochemical hydrogen production using a pH control strategy. Chemsuschem 8, 389–397. doi: 10.1002/cssc.201403083
Schievano, A., Pepé Sciarria, T., Vanbroekhoven, K., De Wever, H., Puig, S., Andersen, S. J., et al. (2016). Electro-fermentation–merging electrochemistry with fermentation in industrial applications. Trends BioTechnol. 34, 866–878. doi: 10.1016/j.tibtech.2016.04.007
Selembo, P. A., Perez, J. M., Lloyd, W. A., and Logan, B. E. (2009). High hydrogen production from glycerol or glucose by electrohydrogenesis using microbial electrolysis cells. Int. J. Hydrogen Energy 34, 5373–5381. doi: 10.1016/j.ijhydene.2009.05.002
Shen, R., Liu, Z., He, Y., Zhang, Y., Lu, J., Zhu, Z., et al. (2016). Microbial electrolysis cell to treat hydrothermal liquefied wastewater from cornstalk and recover hydrogen: Degradation of organic compounds and characterization of microbial community. Int. J. Hydrogen Energy 41, 4132–4142. doi: 10.1016/j.ijhydene.2016.01.032
Sleutels, T., Ter Heijne, A., Buisman, C. J. N., and Hamelers, H. V. M. (2012). Bioelectrochemical systems: an outlook for practical applications. Chemsuschem 5, 1012–1019. doi: 10.1002/cssc.201100732
Song, Q.-H., Nie, J.-Q., Ren, M.-G., and Guo, Q.-X. (2009). Effective phase separation of biomass pyrolysis oils by adding aqueous salt solutions. Energy Fuels 23, 3307–3312. doi: 10.1021/ef900143u
Song, T.-S., Wu, X. Y., and Zhou, C. C. (2014). Effect of different acclimation methods on the performance of microbial fuel cells using phenol as substrate. Bioproc. Biosyst. Engineer. 37, 133–138. doi: 10.1007/s00449-013-0975-6
Steinbusch, K. J., Hamelers, H. V. M., Schaap, J. D., Kampman, C., and Buisman, C. J. N. (2010). Bioelectrochemical ethanol production through mediated acetate reduction by mixed cultures. Environ. Sci. and Technol. 44, 513–517. doi: 10.1021/es902371e
Sun, M., Mu, Z. X., Sheng, G. P., Shen, N., Tong, Z. H., Wang, H. L., et al. (2010). Hydrogen production from propionate in a biocatalyzed system with in-situ utilization of the electricity generated from a microbial fuel cell. Int. Biodeter. Biodegr. 64, 378–382. doi: 10.1016/j.ibiod.2010.04.004
Thygesen, A., Marzorati, M., Boon, N., Thomsen, A. B., and Verstraete, W. (2011). Upgrading of straw hydrolysate for production of hydrogen and phenols in a microbial electrolysis cell (MEC). Appl. Microbiol. BioTechnol. 89, 855–865. doi: 10.1007/s00253-010-3068-3
Thygesen, A., Thomsen, A. B., Possemiers, S., and Verstraete, W. (2010). Integration of microbial electrolysis cells (MECs) in the biorefinery for production of ethanol, H2 and phenolics. Waste Biomass Valoriz. 1, 9–20. doi: 10.1007/s12649-009-9007-9
Tian, Q. K., He, L., Zhang, H., Lv, Z. L., and Li, R. (2011). Study on solvent extraction of fast pyrolysis bio-oil. Adv. Mater. Res. Trans Tech Publ. 306–307, 1532–1536. doi: 10.4028/www.scientific.net/AMR306-307.1532
Van Eerten-Jansen, M. C., Ter Heijne, A., Grootscholten, T. I., Steinbusch, K. J., Sleutels, T. H., Hamelers, H. V., et al. (2013). Bioelectrochemical production of caproate and caprylate from acetate by mixed cultures. Acs Sust. Chem. Engin. 1, 513–518. doi: 10.1021/sc300168z
Vitasari, C. R., Meindersma, G. W., and De Haan, A. B. (2011). Water extraction of pyrolysis oil: The first step for the recovery of renewable chemicals. Bioresour. Technol. 102, 7204–7210. doi: 10.1016/j.biortech.2011.04.079
Wang, A., Sun, D., Cao, G., Wang, H., Ren, N., Wu, W.-M., et al. (2011). Integrated hydrogen production process from cellulose by combining dark fermentation, microbial fuel cells, and a microbial electrolysis cell. Bioresour. Technol. 102, 4137–4143. doi: 10.1016/j.biortech.2010.10.137
Wang, Y., Guo, W.-Q., Xing, D.-F., Chang, J.-S., and Ren, N.-Q. (2014). Hydrogen production using biocathode single-chamber microbial electrolysis cells fed by molasses wastewater at low temperature. Int. J. Hydrogen Energy 39, 19369–19375. doi: 10.1016/j.ijhydene.2014.07.071
Wei, Y., Lei, H., Wang, L., Zhu, L., Zhang, X., Liu, Y., et al. (2014). Liquid–liquid extraction of biomass pyrolysis bio-oil. Energy Fuels 28, 1207–1212. doi: 10.1021/ef402490s
Wilson, E. L., and Kim, Y. (2016). The yield and decay coefficients of exoelectrogenic bacteria in bioelectrochemical systems. Water Res. 94, 233–239. doi: 10.1016/j.watres.2016.02.054
Xafenias, N., Anunobi, M. O., and Mapelli, V. (2015). Electrochemical startup increases 1,3-propanediol titers in mixed-culture glycerol fermentations. Proc. Biochem. 50, 1499–1508. doi: 10.1016/j.procbio.2015.06.020
Xing, D. F., Cheng, S. A., Logan, B. E., and Regan, J. M. (2010). Isolation of the exoelectrogenic denitrifying bacterium Comamonas denitrificans based on dilution to extinction. Appl. Microb. BioTechnol. 85, 1575–1587. doi: 10.1007/s00253-009-2240-0
Xu, R., Ferrante, L., Briens, C., and Berruti, F. (2011). Bio-oil production by flash pyrolysis of sugarcane residues and post treatments of the aqueous phase. J Analyt. Appl. Pyrolys. 91, 263–272. doi: 10.1016/j.jaap.2011.03.001
Zeng, X., Borole, A. P., and Pavlostathis, S. G. (2015). Biotransformation of furanic and phenolic compounds with hydrogen gas production in a microbial electrolysis cell. Environ. Sci. and Technol. 49, 13667–13675. doi: 10.1021/acs.est.5b02313
Zeng, X., Borole, A. P., and Pavlostathis, S. G. (2016a). Inhibitory effect of furanic and phenolic compounds on exoelectrogenesis in a microbial electrolysis cell bioanode. Environ. Sci. and Technol. 50, 11357–11365.
Zeng, X., Borole, A. P., and Pavlostathis, S. G. (2016b). Performance evaluation of a continuous-flow bioanode microbial electrolysis cell fed with furanic and phenolic compounds. RSC Adv. 6, 65563–65571. doi: 10.1039/C6RA13735K
Zeng, X., Borole, A. P., and Pavlostathis, S. G. (2018). Processes and electron flow in a microbial electrolysis cell bioanode fed with furanic and phenolic compounds. Environ. Sci. Poll. Res. doi: 10.1007/s11356-018-1747-2. [Epub ahead of print].
Zeng, X., Collins, M. A., Borole, A. P., and Pavlostathis, S. G. (2017). The extent of fermentative transformation of phenolic compounds in the bioanode controls exoelectrogenic activity in a microbial electrolysis cell. Wat. Res. 109, 299–309. doi: 10.1016/j.watres.2016.11.057
Zhou, M., Chen, J., Freguia, S., Rabaey, K., and Keller, J. R. (2013). Carbon and electron fluxes during the electricity driven 1, 3-propanediol biosynthesis from glycerol. Environ. Sci. and Technol. 47, 11199–11205. doi: 10.1021/es402132r
Keywords: microbial electrolysis, bioelectrochemical, electro-fermentation, bio-oil, pyrolysis, renewable hydrogen, bio-electro-refinery, biorefinery
Citation: Borole AP, Tsouris C, Pavlostathis SG, Yiacoumi S, Lewis AJ, Zeng X and Park L (2018) Efficient Conversion of Aqueous-Waste-Carbon Compounds Into Electrons, Hydrogen, and Chemicals via Separations and Microbial Electrocatalysis. Front. Energy Res. 6:94. doi: 10.3389/fenrg.2018.00094
Received: 26 April 2018; Accepted: 27 August 2018;
Published: 19 September 2018.
Edited by:
Abudukeremu Kadier, National University of Malaysia, MalaysiaReviewed by:
Pascal E. Saikaly, King Abdullah University of Science and Technology, Saudi ArabiaHyungKuk Ju, Commonwealth Scientific and Industrial Research Organisation (CSIRO), Australia
G. Venkata Subhash, Reliance Industries, India
Copyright © 2018 Borole, Tsouris, Pavlostathis, Yiacoumi, Lewis, Zeng and Park. This is an open-access article distributed under the terms of the Creative Commons Attribution License (CC BY). The use, distribution or reproduction in other forums is permitted, provided the original author(s) and the copyright owner(s) are credited and that the original publication in this journal is cited, in accordance with accepted academic practice. No use, distribution or reproduction is permitted which does not comply with these terms.
*Correspondence: Abhijeet P. Borole, YWJvcm9sZUB1dGsuZWR1