- Department of Energy Engineering, ETSII, Technical University of Madrid, Madrid, Spain
The reduction and control of carbon dioxide emissions of the energy system and other industrial processes using fossil resources is one of the main concerns of our society. Natural gas may contribute to reduce such emissions as it is the fossil with the highest H/C ratio. In addition, a deep reduction of emissions requires advanced technologies such as carbon capture and sequestration (CCS) or utilization (CCU), or direct fossil decarbonization. Some practical approaches integrated in the power-to-gas scheme to the application of natural gas decarbonization are presented. The introduction of this technology in hydrogen mobility seems to be viable, providing a carbon-free production on site without hydrogen or carbon dioxide transport and storage. The integration of direct decarbonization into combined cycle plants for centralized electricity production is justified for carbon penalties above 50 €/kg.
Introduction
Natural gas plays a very important role in our society as a raw material for a great variety of industrial processes. Its utilization as primary energy source has been consolidated during the past few decades due its high hydrogen/carbon ratio, efficient combustion, and lower amount of contaminants in the exhausted gases, including lower carbon dioxide emissions. The technological shift from other fossil fuels (such as coal and oil) to natural gases has contributed to a considerable reduction of carbon footprint of energy production, penetrating in many sectors from electricity generation to heating system for houses. This smooth penetration has been produced in parallel with the development of the associated infrastructures composed of local, national, and transnational gas grids, as well as gasification plants and gas carrier ships.
Natural gas meets the requirement of a high share of the energy demand, but next phase is a challenging phase in which the concerns about global warming are motivating the energy transition to a decarbonized energy system. Natural gas may play a very important role in that energy transition. Available gas resources are far from being exhausted, thereby accounting from conventional to unconventional extraction techniques, as well as the potential reserves in the form of methane hydrates. The lower carbon dioxide emission rate in comparison with coal and oil is forcing to utilize natural gas in most of the energy transition strategies to achieve environmental targets in the long term (i.e., 2050).
Nevertheless, to continue using natural gas “as business as usual” is not enough to comply with the demands of our society, and the utilization of natural gas resources in a low-carbon economy implies the development of new technologies to achieve negligible greenhouse gas emissions. To fulfill such objective, the release of carbon compounds in the atmosphere due to the utilization of natural resources must be avoided. Three main options are possible: carbon capture and sequestration (CCS), carbon capture and utilization (CCU), and direct decarbonization (Abbasi and Abbasi, 2011). The CCS approach is facing several technological, economic and social difficulties. Some experts have concluded that “alternative emission mitigation technologies are potentially the only solution that could rescue us from the dire situation that we are heading toward by the end of this century” (Maddali et al., 2015). Hence, the development of fossil-fuel decarbonization may constitute an important alternative into the technological portfolio that could contribute to climate change mitigation, enabling the utilization of natural gas during a fast energy transition.
Natural Gas Decarbonization
Natural gas decarbonization constitutes the splitting of natural gas into its components: solid carbon and hydrogen. Methane is the main compound of the natural gas (and the basic molecule of synthetic natural gas (SNG), main vector of the Power-to-Gas scheme). Methane decomposition is described by the following general reaction:
The methane decomposition reaction is endothermic with the need to apply energy to remove the strong C-H bonds (dissociation energy: 436 kJ/mol). There are lots of work available about this reaction including extensive reviews (Abbas and Wan Daud, 2010; Amin et al., 2011) and experimental works with several types of catalysts (Botas et al., 2010; Pudukudy and Yaakob, 2015). Such energy requirements imply that temperature over 500°C is required to start methane decomposition, reaching high conversion rates over 1,100°C. Conversion rates from 10% at 500°C (Li et al., 2006) to 95% at 1,050°C (Maag et al., 2009) have been achieved with different catalysts. Catalysts have shown some difficulties during their implementation at industrial scale due to their deactivation either by coke accumulation on their active surface in metal catalysts or by the reduction of the superficial properties of the carbonaceous catalysts (Abánades et al., 2012). In particular, in the case of heterogeneous catalysts, their regeneration relies on the air/steam oxidation of the coke, producing carbon dioxide and risking sintering. The most relevant implementation of catalyzed thermal decarburization was the HYPRO process based on fluidized bed reactors (Poblenz and Scot, 1966).
There are other technological options offering the possibility to develop natural gas decomposition. The utilization of a plasma-arc to drive methane pyrolysis has been tried at industrial level from the 90's. The main attempt was the development of the Kvaerner CB&H process (Gaudernack and Lynum, 1998). The production capacity of the Kvaerner plant was 500 kg/h of pure carbon and 2,000 Nm3/h of Hydrogen. However, the factory was shut down due to some problems with the plasma section. In fact, this process is considered as the reference industrial development for methane decomposition. Other similar practical implementations are proposed based on the production of carbonaceous aerosols with plasma technology (Muradov et al., 2009).
Direct thermal cracking can develop methane decomposition at relatively high temperatures (above 1,000°C) with reasonable conversion rates, thereby providing suitable methane residence times into the reactor (Abánades et al., 2011) or very high temperatures (more than 1,500°C, when reaction kinetics are very fast). There were even some proposals of a pilot-plant design for direct methane decomposition (Rodat et al., 2011). One of the main concerns about these methods is the extraction of carbon from the reactor, which forms very hard graphitic deposits at high temperatures. Additional technologies are under development, such as the utilization of a liquid metal reaction media (Geißler et al., 2015, 2016).
New technological developments should be put into practice to limit climate change, as pointed out by many authors (Davis et al., 2010). Finding a technological solution for continuing the utilization of fossil-fuel resources while avoiding CO2 emissions is key to achieving the climate protection targets fixed in international fori, as the Paris Agreement on Climate Change. Technology like methane decomposition could serve as a bridging solution during the transition from a fossil-fuel based economy to a more sustainable one (Weger et al., 2017), making it possible to exploit the available resources until a new system is completely implemented.
Scheme for a Low-Carbon Society
The energy transition toward a new energy model based on a CO2-emission-free society is one of the most critical challenges for our global community. It implies the integral transformation of our social and productive metabolisms at several levels such as energy production (shifting massively from fossil to renewable energy sources), energy vectors (heat, electricity, gas, liquid fuels, etc.), and final utilization (industry, transportation, housing, services, etc.). Such transformation must include new advance technologies to make possible a transition from an energy system sustained by centralized energy generation toward a distributed one, in which the clear difference between energy producers and consumers vanishes. As an example, massive energy storage is one of the critical issues either for the management of a distributed energy grid with micro-storage (as likely car batteries) or for home storage systems. The practical implementation of smart grids (electrical) or power-to-gas schemes (thermal/chemical) (Götz et al., 2016) will likely imply changes in the social habits to achieve the goal of their efficient and smooth management, the creation of new business models and opportunities, and deep changes on our society. These changes will be global, with a very important impact at international level as such energy transition should take into account the interconnections between different regions, countries, and multinational clusters, as the European Union, or the Eurasia, or MENA regions. The system, on the other hand, will be built at local level, forming small energy communities. Their interconnections will be handled by the application of big data technologies to control the energy market based on huge amount of prosumers (simultaneously producers and consumers of energy, including self-storage capabilities). This transition to a new energy model should be adapted and applied to the whole spectrum of economic, climatic, and demographic characteristics of different countries in the world.
One of the options to provide storage capability and flexibility to an evolved energy system is the power-to-gas (Blanco and Faaij, 2018) or power-to-liquid (Buttler and Spliethoff, 2018) scheme. In such scheme, intermittent energy source generation is stored into a chemical serving as energy storage, joining the sustainability and environmental benefits of a clean source and the management capability of a massive storage.
The proposals for chemical intermediate vectors are methane, ammonia, and hydrogen, or a combination of them, in stages, depending of the final use or main chemical energy storage buffer. In the power-to-gas option, wind mills and photovoltaic plants are intended to be the main primary energy input, in which intermittent and variable electricity generation is managed by a high storage capacity in the form of SNG produced by their surplus production. The existing infrastructure for the logistics of natural gas (storage sites and reservoirs, and the pipelines) are used without increasing drastically energy system costs. Additional carbon-free electricity may be produced from biomass or from concentrated solar thermal of clean fossil plants (applying technologies as CCS and CCU, as well as decarbonization). Hydraulic utilities have double role of production and storage of energy and may contribute to tune the scheme.
Methane decarbonization may be a key technology applied near the energy end-user on power-to-gas option. The energy stored in the form of SNG is delivered to the consumers through the gas pipes. The consumers include industrial factories, service utilities, or households. Natural decarbonization will enable the direct carbon capture by the transformation of natural gas into hydrogen, making possible the application of fuel cell technologies or direct H2 oxidation. The development of a scalable and modular process to split methane into pure carbon and hydrogen may be very applicable for sites requiring a few kW of power, such as households, to several MW for industrial applications. Obviously, it will depend on the technological possibility for hydrogen utilization, being more applicable in medium and high power facilities, as it is the case of hydrogen stations for vehicles or ammonia production, or as a technique for carbon capture in gas facilities, where the economy of scale and the need for hydrogen or carbon capture processes are very relevant. Figure 1 illustrates the application of methane decarbonization near the end–user in power-to-gas option. Next, the case of the application of this technology into a hydrogen station and a natural gas combined cycle will be analyzed.
Case Studies for Methane Decarbonization Into Power-to-Gas
In this paper, two cases illustrate the potential application of decarbonization into the power-to-gas scheme. Two business models for the implementation of methane decomposition are considered: A medium-sized facility producing of the order of some units of MW consisting of a hydrogen station and an industrial installation integrated into a natural gas combined cycle as a tool for carbon capture. Notice that both cases receive natural gas from external gas pipes and transform it locally into hydrogen, capturing carbon, and eliminating the need for hydrogen transport and storage, as natural gas is the energy carrier vector.
The economic viability of the hydrogen station is determined by two well-known parameters such as the net present value (NPV) and the internal return rate (IRR), which are defined in function of the cash flow (CF), the weighted average cost of capital (r), and the number of years (n) as:
IRR corresponds to the worth of r to set NPV = 0. CAPEX is the initial capital cost invested at the beginning of the operational life of the station.
In the case of application to a natural gas combined cycle (NGCC), the key parameter for the evaluation of the economic viability of the plant is the levelized cost of electricity (LCOE) that has been estimated by the following expression:
CAPEX is the capital cost of the plant spent at the beginning of its lifespan. &M is the operation and maintenance (O&M) cost at the year t, Ctfuel is the cost of the natural gas at the year t, CtCO2 is the carbon taxes at the year t, and r is the weighted average cost of capital (WACC). N is the number of years of the analysis. Et is the energy produced in the year t. The following expression represents the levelized OPEX (LOPEX) including O&M with fuel consumption and carbon taxes:
Costs are evaluated based on its worth at the initial year (t = 0) (, , ) and the inflation rate i for the year t, according to:
Hydrogen Station
The deployment of the hydrogen economy in the mobility sector implies an increasing number of hydrogen delivery stations for vehicles in parallel with the evolution of the number of hydrogen-driven engines on the roads. One of the most important issues regarding hydrogen mobility is how to get hydrogen in the filling stations. The cheaper production of hydrogen in centralized facilities is one option, but the technical difficulties and the cost of hydrogen storage and its transport to the filling stations penalize this alternative. On the contrary, the solution of transporting natural gas to the stations and producing the hydrogen locally to charge the vehicles seems to be a viable option. Most of the projects with duty hydrogen vehicle fleets are based on the local hydrogen generation by methane steam reforming on the site. Nevertheless, their integration into a low-carbon economy must include any kind of carbon sequestration technology, which reduces their economic competitiveness.
A hydrogen station based on methane decarbonization is depicted in Figure 2. A conventional pipe of the gas network in the area provides natural gas to the station. The natural gas infrastructure will depend on the total power delivered by the station, mainly the number of hydrogen nozzles. Natural gas is then processed in the decomposition system, which comprises a cracking reactor and a separation stage for the extraction of the hydrogen from the rest of the components of the reactor outlet (mostly unreacted methane). Membrane separation technology is proposed for the extraction of pure hydrogen stream that follows a set of compression stages to reach the 350/700 bar for its injection into the vehicle.
A cost analysis has been done for a reference hydrogen station delivering 150 kg/h of hydrogen working at full capacity 16 h/day equivalent to a capacity factor of 0.66. The total amount of carbon produced is 572 kg/h, which should be managed as a waste or as a product. As main hypothesis for the economic analysis, carbon worth is set to null. The capital cost (CAPEX) of the installation according to our analysis is evaluated as 1.1 €/W, what imply a total cost for our station of 5.5 M€. In that figure, the decarbonization process equipment, the separation membrane based on Pd-Cu (60/40), as well as the hydrogen compression/buffering equipment are included.
We evaluated the operational costs (OPEX) of the facility, being of the order of 4.1 €/kgH, including manpower and substitution of spare parts during operation. The energy for the compression stage corresponds to approximately 25% of the total cost. Such analysis has been done for European cost of natural gas (11 €/GJ as upper limit including taxes 2nd Sem. 2016; Eurostat, 2018). This cost is higher to that of the natural gas in international markets as it is applied to a medium consumer. The total levelized cost of hydrogen for a typical 20 year lifetime is 5.19 €/kgH2.
This figures has to be compared with the market cost of hydrogen paid by the vehicle owners, which has been estimated as 8 €/kgH2 (+VAT). Compression costs are very relevant. There may be reduced developing hydrogen storage at lower pressure, as it is in the case of metal hydrates.
With such economic values, the economic analysis of a hydrogen station based on natural gas decarbonization shows an internal return rate (IRR) of 14% and a NPV of 2.8 M€ in 20 years.
Natural Gas Combined Cycle (NGCC)
The reduction of carbon dioxide emissions from centralized power plants is one of the key technological alternatives to integrate the utilization of fossil resources in a low-carbon society. Coal plants must implement carbon capture sections to apply CCS or CCU as the main path to reduce its carbon footprint. Hydrocarbon-based plants, such as NGCC, using a fuel with a very high H/C ratio can integrate fossil decarbonization in addition to carbon capture systems. The reduction of CO2 generation in a NGCC will depend on the fraction of feeding natural gas being converted into hydrogen. In the case of a full conversion into hydrogen and carbon, the hypothetical plant eliminates CO2 process emissions, substituting the management of gaseous carbon dioxide by solid carbon, and avoids carbon taxes.
The direct application of natural gas decarbonization to carbon capture in a natural gas combined cycle is shown in Figure 3. Feeding natural gas enters a pyrolysis reactor operating at 1,200°C achieving a molar conversion of methane into hydrogen close to 80%. The gas output from the reactor is a mixture of hydrogen and natural gas, which is almost 50/50 in weight. In this analysis, for a total power of 460 MWe, the pyrolysis section processes 27 kg/s of natural gas, extracting 16.26 kg/s of carbon and producing a flow of 8.5 kg/s of gas fuel (4.21 kg/s gas, 4.29 kg/s H2) that is injected into the combustion chamber of the gas turbine.
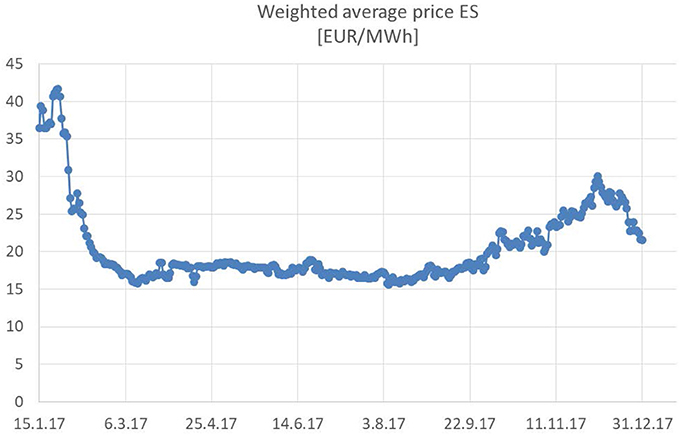
Figure 3. Average market natural gas price into the Iberian market (MIBGAS, 2017).
Around 15% of the gas is used to drive the endothermic reaction (1.1 kg/s gas, 1.2 kg/s H2). Taking into account the efficiency of the transformation of natural gas into hydrogen, of the order of 53% and the efficiency of the combined cycle of 63%, the overall efficiency of the plant with direct carbon capture is 34%. The CO2 emission rate is reduced from 348 kg/MWh in the conventional combined cycle plant to 115 kg/MWh with the application of decarbonization burning a 50/50 H2/CH4 fuel mixture.
The reduction in efficiency of a natural gas combined cycle is produced because the carbon capture contains a high fraction of energy of the natural gas feed. Almost 40% of the initial energy content of natural gas is discarded by its decarbonization. Nevertheless, such carbon is not producing CO2 and not releasing the energy of its combustion but avoiding the energy required for its further sequestration or reduction for CO2 utilization.
From the definition of the process depicted in Figure 4, a cost analysis has been done. The components for the evaluation of the LCOE are depicted in Table 1. Natural gas price is the main input. The NGCC case is located in Spain; hence, natural gas cost has been taken according to the Iberian market. Such daily average price is shown in Figure 3 for 2017. On the analysis, natural gas has been fixed to 20 €/MWh. The cost estimates for the process resulted in a LCOE (Levelized Cost of Electricity) in Spain is 121.8 €/MWh, vs. the average LCOE for NGCC of 78.8 €/MWh. This scheme is only viable if the taxes for CO2 emissions are above 105 €/tonCO2 to compensate the plant efficiency reduction and the increase in fuel consumption. In world regions with lower natural gas prices, as it is the case of United States (around 11 €/MWh), CO2 penalties above 50 €/tonCO2 make viable utilization of direct decarbonization.
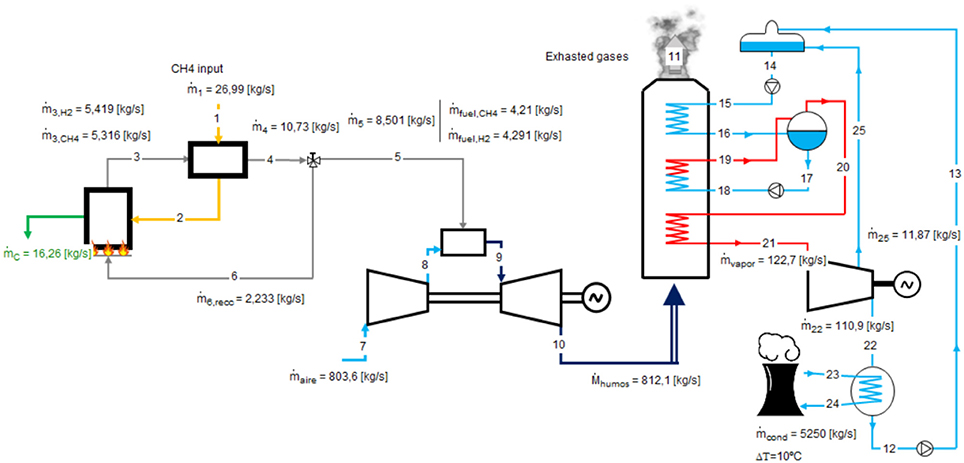
Figure 4. Model for the evaluation of the application of natural gas decarbonization to a combined cycle.
Additional income from the production of graphitic carbon can be considered. Nevertheless, the massive amount of carbon will imply a negligible impact on the economics of the application of decarbonization to this scheme in comparison with the uncertainties of the cost evaluation at this stage of development. On the other hand, it may have an impact on future technology development in the field of carbonaceous materials or the steel industry, as cheap carbon raw materials will be available.
Conclusion
Decarbonization is a technological option under development as an alternative to other techniques such as carbon capture sequestration and utilization (CCS and CCU), which are proposed for the deep reduction of greenhouse gas emissions in the consumption of natural gases. On the other hand, the energy sector is facing paramount challenges that may imply a deep transformation of the energy market and risks for the oil and gas companies, unless they put in place efficient low-carbon technologies. A brief description of natural gas decomposition has been done, explaining its integration into a power-to-gas scheme as a main grid for thermal/chemical energy management in the future. Natural gas decarbonization would enable the utilization of hydrogen in the system without the need of massive hydrogen storage and transport, keeping SNG as the main energy carrier using existing gas logistic infrastructures. The basic economic analysis of the application of decarbonization to a distributed typical application, such as a hydrogen station, and a centralized facility, such as a natural gas combined cycle, has been reported.
The integration of natural gas decomposition in the hydrogen economy and mobility is viable according to the analysis with an IRR of 14%. Regarding its integration in a combined cycle plant, even if the hydrogen produced is cheaper due to the higher scale, the efficiency plant reduction to a 34% is only compensated by carbon taxes between 51 and 105 €/ton CO2.
Additional improvements in the economic viability of those processes may be achieved by carbon selling, as natural gas decomposition produces graphitic carbon. As the carbon market is much smaller than the energy market, such revenues are not considered realistic if natural gas decomposition is applied at industrial scale.
Author Contributions
This work is part of the effort that the author has done for the integration of natural gas decarbonization into our Society, and, in particular in the context of the Power-to-Gas scheme. The work has been done on his position as staff of the Technical University of Madrid.
Conflict of Interest Statement
The author declares that the research was conducted in the absence of any commercial or financial relationships that could be construed as a potential conflict of interest.
Acknowledgments
The author has to acknowledged the discussion about this topic with his department colleages and his students as Alejandro Gallego and Luis Fernández-Vittorio.
References
Abánades, A., Rubbia, C., and Salmieri, D. (2012). Technological challenges for industrial development of hydrogen production based on methane cracking. Energy 46, 359–363. doi: 10.1016/j.energy.2012.08.015
Abánades, A., Ruiz, E., Ferruelo, E. M., Hernández, F., Cabanillas, A., Martínez-Val, J. M., et al. (2011). Experimental analysis of direct thermal methane cracking. Int. J. Hyd. Energ 36, 12877–12886. doi: 10.1016/j.ijhydene.2011.07.081
Abbas, H. F., and Wan Daud, W. M. A. (2010). Hydrogen production by methane decomposition: a review. Int. J. Hyd. Energ 35, 1160–1190. doi: 10.1016/j.ijhydene.2009.11.036
Abbasi, T., and Abbasi, S. A. (2011). Decarbonization of fossil fuels as a strategy to control global warming. Renew. Sustain. Energ. Rev. 15, 1828–1834. doi: 10.1016/j.rser.2010.11.049
Amin, A. M., Croiset, E., and Epling, W. (2011). Review of methane catalytic cracking for hydrogen production. Int. J. Hyd. Energ. 36, 2904–2935. doi: 10.1016/j.ijhydene.2010.11.035
Blanco, H., and Faaij, A. (2018). A review at the role of storage in energy systems with a focus on power to gas and long-term storage. Renew. Sustain. Energ. Rev. 81, 1049–1086. doi: 10.1016/j.rser.2017.07.062
Botas, J. A., Serrano, D. P., Guil-López, R., Pizarro, P., and Gómez, G. (2010). Methane catalytic decomposition over ordered mesoporous carbons: a promising route for hydrogen production. Int. J. Hyd. Energ. 35, 9788–9794. doi: 10.1016/j.ijhydene.2009.10.031
Buttler, A., and Spliethoff, H. (2018). Current status of water electrolysis for energy storage, grid balancing and sector coupling via power-to-gas and power-to-liquids: a review. Renew. Sustain. Energ. Rev. 82, 2440–2454. doi: 10.1016/j.rser.2017.09.003
Davis, S. J., Caldeira, K., and Matthews, H. D. (2010). Supporting online material for future co 2 emissions and climate change from existing energy infrastructure. Methods 1330, 1330–1333. doi: 10.1126/science.1188566
Eurostat (2018). Eurostat Database on Energy. Available online at: http://ec.europa.eu/eurostat/web/energy/data/database.
Gaudernack, B., and Lynum, S. (1998). Hydrogen from natural gas without release of CO2 to the atmosphere. Int. J. Hyd. Energ. 23, 1087–1093. doi: 10.1016/S0360-3199(98)00004-4
Geißler, T., Abánades, A., Heinzel, A., Mehravaran, K., Müller, G., Rathnam, R., et al. (2016). Hydrogen production via methane pyrolysis in a liquid metal bubble column reactor with a packed bed. Chem. Eng. J. 299, 192–200. doi: 10.1016/j.cej.2016.04.066
Geißler, T., Plevan, M., Abánades, A., Heinzel, A., Mehravaran, K., Rathnam, R., et al. (2015). Experimental investigation and thermo-chemical modeling of methane pyrolysis in a liquid metal bubble column reactor with a packed bed. Int. J. Hyd. Energ. 40, 14134–14146. doi: 10.1016/j.ijhydene.2015.08.102
Götz, M., Lefebvre, J., Mörs, F., Koch, A. M., Graf, F., Bajohr, S., et al. (2016). Renewable power-to-gas: a technological and economic review. Renew. Energ. 85, 1371–1390. doi: 10.1016/j.renene.2015.07.066
Li, Y., Zhang, B., Xie, X., Liu, J., Xu, Y., and Shen, W. (2006). Novel Ni catalysts for methane decomposition to hydrogen and carbon nanofibers. J. Catal. 238, 412–424. doi: 10.1016/j.jcat.2005.12.027
Maag, G., Zanganeh, G., and Steinfeld, A. (2009). Solar thermal cracking of methane in a particle-flow reactor for the co-production of hydrogen and carbon. Int. J. Hyd. Energ. 34, 7676–7685. doi: 10.1016/j.ijhydene.2009.07.037
Maddali, V., Tularam, G. A., and Glynn, P. (2015). Economic and time-sensitive issues surrounding ccs: a policy analysis. Environ. Sci. Technol. 49, 8959–8968. doi: 10.1021/acs.est.5b00839
MIBGAS (2017). MIBGAS, Mercado Ibérico Del Gas. MIBGAS Web Page. Available online at: http://www.mibgas.es/aplicaciones/datosftpGAS/datosftp.jsp?path=//datos_GAS//AGNO_2017//XLS/
Muradov, N., Smith, F., Bockerman, G., and Scammon, K. (2009). Thermocatalytic decomposition of natural gas over plasma-generated carbon aerosols for sustainable production of hydrogen and carbon. Appl. Catal. A General 365, 292–300. doi: 10.1016/j.apcata.2009.06.031
Poblenz, J. B., and Scott, N. H. (1966). Method for Hydrogen Production by Catalytic Decomposition of a Gaseous Hydrocarbon Stream. Patent No: US3284161A. Universal Oil Products Co.
Pudukudy, M., and Yaakob, Z. (2015). Methane decomposition over ni, co and fe based monometallic catalysts supported on sol gel derived sio2 microflakes. Chem. Eng. J. 262, 1009–1021. doi: 10.1016/j.cej.2014.10.077
Rodat, S., Abanades, S., and Flamant, G. (2011). Co-production of hydrogen and carbon black from solar thermal methane splitting in a tubular reactor prototype. Solar Energ. 85, 645–652. doi: 10.1016/j.solener.2010.02.016
Keywords: decarbonization, natural gas, low-carbon economy, hydrogen economy, carbon capture, power-to-gas
Citation: Abánades A (2018) Natural Gas Decarbonization as Tool for Greenhouse Gases Emission Control. Front. Energy Res. 6:47. doi: 10.3389/fenrg.2018.00047
Received: 23 March 2018; Accepted: 14 May 2018;
Published: 19 June 2018.
Edited by:
Fernando Bimbela, Universidad Pública de Navarra, SpainReviewed by:
Consuelo Alvarez-Galvan, Consejo Superior de Investigaciones Científicas (CSIC), SpainMuhammad Aziz, Tokyo Institute of Technology, Japan
Copyright © 2018 Abánades. This is an open-access article distributed under the terms of the Creative Commons Attribution License (CC BY). The use, distribution or reproduction in other forums is permitted, provided the original author(s) and the copyright owner are credited and that the original publication in this journal is cited, in accordance with accepted academic practice. No use, distribution or reproduction is permitted which does not comply with these terms.
*Correspondence: Alberto Abánades, YWJhbmFkZXNAZXRzaWkudXBtLmVz