- Institute of Biological Environmental and Rural Sciences, Aberystwyth University, Aberystwyth, United Kingdom
Xylitol is a low calorie sweetener that can be produced through a bioconversion approach from lignocellulosic biomass that requires pre-treatment prior to the bioconversion of xylose to xylitol. Steam explosion (SE) is an industrially scalable pre-treatment (PT) process with the potential to liberate xylose monomers, however SE-PT has not been optimized for xylose release from multiple feedstock. The effect of pressure, substrate weight, phosphoric acid loading concentration and residence time on four feedstock [wheat straw (WS), corn stover (CS), Miscanthus (M), and willow (W)] for xylose release and minimal fermentation inhibitor production [furfural and 5-hydroxymethylfurfural (HMF)] was investigated using the Taguchi methodology for design of experiment (DoE) with variation at four levels (44). An L16 orthogonal array design was utilized and all factors indicated influence on xylose release and inhibitor formation and the resulting xylose rich hydrolysate assessed for bioconversion to xylitol. The L16 DoE gave hydrolysates containing 75–95% of xylose content in the original biomass, whilst retaining cellulose and lignin components in the fiber. The level of inhibitors were within boundary limits to enable microbial fermentation of the hydrolysates to xylitol. Fine tuning of the overall evaluation criteria (OEC) model imbibing 1.5 kg feedstock in 1.2% w/v orthophosphoric acid, 12 bar(g) and 6 min residence time resulted in 90% xylose recovery and production of >1,000 L of wheat straw hydrolysate for bioconversion to xylitol. The advantages and limitations of the Taguchi OEC model and further improvements to this process are discussed in a biorefining context.
Introduction
Use of fossil fuels has impacted national energy security and global warming, leading to an increased investigation into the use of biomass as a renewable substrate to generate multiple products (Maity, 2014). Key biomass resources include lignocellulosic agricultural residues and dedicated energy crops (2G resources), neither of which impact on food security, unlike 1G plant resources which enter the food v fuel debate. Major global biomass resources include; wheat straw, corn stover, short rotation coppice willow, and Miscanthus. O'Donohue (2014) reported that within Europe, 35 Mt DM wheat straw, 15 Mt DM corn stover, 2.5–5.5 Mt DM surplus hardwood are available for use as biorefining feedstock. In addition, Miscanthus a perennial C4 grass is currently receiving much research attention for use as a dedicated energy crop, bioremediation of soils, and enhancement of grade 3–5 (marginal) land meaning that in the future this could become a widely used crop across the UK and the world. (Heaton et al., 2008; Haughton et al., 2009; O'Donohue, 2014; Rettenmaier et al., 2014; Purdy et al., 2015; Hodgson et al., 2016; Clifton-Brown et al., 2017).
Biomass has predominantly been used for the production of energy to reduce dependence on fossil fuel imports and reduce carbon output, often utilizing biomass for a single product. A European report by O'Donohue (2014) highlighted the need to couple ethanol generation from cellulose with production of multiple products from the hemicellulose and lignin components. An analysis of multiple product portfolios indicated that generation of xylitol from the hemicellulosic fraction made significant improvement to the economic returns of a lignocellulosic based process (O'Donohue, 2014).
Xylitol is a sugar alcohol estimated to achieve a market value of $1 billion by 2020 and is of interest to pharmaceutical and nutraceutical industries due to its multiple health benefits; low glycaemic index, and reduction of dental carries (Venkateswar Rao et al., 2016). The current production of this sweetener is performed via an energy intensive thermo-chemical synthesis route using corn cobs and birch wood hydrolysate. A sustainable production system through biomass pre-treatment to liberate xylan and subsequent bioconversion of xylose to xylitol has previously been considered (Mohamad et al., 2015). To the best of the author's knowledge, an optimized pre-treatment process to release xylose from multiple feedstock without undue release of fermentation inhibitors has not been carried out.
A myriad of pre-treatment techniques to facilitate deconstruction of lignocellulosic feedstock have been developed (e.g., biological, thermo-chemical, and mechanical) but few processes have been shown to be scalable (Alvira et al., 2010; Guerrero et al., 2017). Steam explosion is a simple hydrothermal, scalable, pre-treatment technology developed in 1926 that is industrially deployed to process tones of material per hour and has been used on a variety of feedstock (Mason, 1926; Hu and Ragauskas, 2012; Bryant et al., 2013; Martijn, 2015; Rabemanolontsoa and Saka, 2016). Much of the research utilizing steam explosion pre-treatment has focused on imbibing feedstock with sulfuric acid to serve as a catalyst (Eklund et al., 1995; Zimbardi et al., 2007; Sørensen et al., 2008; Martijn, 2015). Use of this harsh acid requires expensive capital costs for full-scale facilities due to the requirement of equipment to be constructed from exotic steel alloys, such as hastelloy, to withstand acid corrosion (Harmsen et al., 2010; Castro et al., 2014). Employing phosphoric acid as a catalyst enables conventional 316 stainless steel to be used in the reactor design and readily solubilizes the more labile hemicellulose fraction without releasing cellulose and further degrading the sugars to the fermentation inhibitors furfural and 5-Hydroxymethylfurfural (HMF) (Castro et al., 2014). For each of the feedstock wheat straw has had a substantial amount of SE-PT attention, as has corn stover, with few on Miscanthus or willow (Horn et al., 2011; Behera et al., 2014; Ding et al., 2018). As both the release of xylose and generation of inhibitors via SE-PT were unknown, multiple feedstock were evaluated in order to, (1) produce hydrolysates compatible for maximum xylitol yield and productivity and (2) address the issue of realistic feedstock supply for a commercial biorefinery.
To produce an economically sustainable SE-PT process, many individual process steps require optimization. Hundreds of experiments are necessary when following a full factorial design methodology leading to extensive labor input, costs, and time, particularly when performed at pilot scale. An alternative is to utilize design of experiment methodology such as the Taguchi method (Rao et al., 2008) that employs orthogonal array experimental designs, thereby reducing the number of runs required to obtain an optimum set of levels. The Taguchi method has been reviewed and critically appraised against other DoE methods such as Response Surface Methodology (RSM) and been shown to require less experiments to generate similar results to RSM (Aggarwal et al., 2008; Rao et al., 2008). Alongside RSM requiring significantly more experiments to achieve similar optimized results, the statistical design relates to number of variables not the statistical factorials that results in Taguchi DoE giving a more fully developed process (Box and Hunter, 1957; Rao et al., 2004). Selecting the key SE-PT factors and dividing these into several levels the Taguchi method provides the experimental conditions required before using statistical analysis to determine, from the results, the optimum level for each parameter to achieve the best xylose release.
The approach adopted in investigating and optimizing phosphoric acid SE-PT at pilot scale to release maximal levels of thermo-labile xylose for higher value xylitol production, differs from current research that has tended to focus on lab scale SE-PT for carbohydrate release for bioethanol production (Ruiz et al., 2008; Negro et al., 2014; Sharma et al., 2015). To deliver a successful industrial biotechnological (IB) xylitol production process at commercial scale, multiple obstacles need to be overcome within the IB process that are then optimized and validated at both pilot and demonstration scale. The data presented here address the hurdles of feedstock selection, microbial biocompatibility of the crude hydrolysate, process parameter selection and design space stability of SE-PT. Recently, O'Donohue (2014) highlighted the main issues facing the development of a xylitol IB process were the availability of hydrolysate bioconversion, along with purification and crystallization.
The aim of this study was to identify the effect of four SE-PT parameters; acid loading, substrate weight, pressure, and time, on four feedstock; wheat straw, corn stover, willow, and Miscanthus to attain an optimal set of SE-PT conditions for xylose release and subsequent bioconversion to xylitol at pilot scale. With optimized conditions more than 1,000 l of hydrolysate was produced, concentrated and stored for bio conversions. A critique of the Taguchi method as applied to this pre-treatment process is presented and future optimization scenarios are discussed.
Materials and Methods
Design of Experiment and Analysis
The Taguchi approach used Qualitek 4 DOE software with process factors and levels selected combined into an L16 orthogonal array defining 16 unique experimental conditions and gave account of all process factor combinations (Table 1).The 16 experiments were applied to each of the four feedstock with the four factors being selected based on preliminary experimental procedure (data not published) and literature review (Thomsen and Schmidt, 1999; Castro et al., 2014; Guerrero et al., 2017). Data handling and analysis were performed via “bigger is better” performance characteristic utilizing Qualitek 4 DOE software (USA), Microsoft Excel (Microsoft, USA), and SPSS (IBM, USA).
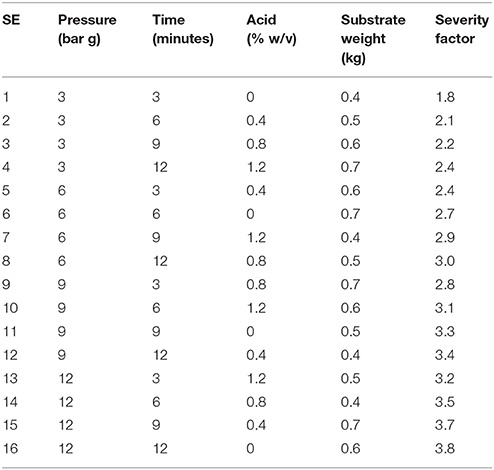
Table 1. L16 orthogonal array of designed experiments with factors, levels and associated severity factors.
Biomass Feedstock
Miscanthus × giganteus (M) was harvested at Aberystwyth University, UK, in spring 2012, and air-dried (<15% w/w MC). Corn stover (Zea Mays Spp) (CS) consisting of maize stalk and leaves was collected from Rothamstead research station, UK, oven dried to <15% moisture content. Air-dried (<15% w/w MC) baled wheat straw (Triticum) spp. (WS) was purchased from R.J. Edwards Agricultural suppliers, Aberystwyth, UK. Willow (Salix Spp.) W) var. EnDurance was harvested in January 2014 at Aberystwyth University, chipped (Jensen 540T, Germany) during harvest and immediately oven dried <10% w/w DM at 60°C to prevent fungal growth and preserve the biomass. All feedstock were milled (<40 mm) (15 kW BC, Electra, Poudenas, France) prior to pre-treatment by steam explosion.
Steam Explosion Pre-treatment
Prior to SE-PT each feedstock was impregnated with 1/5 w/v water imbibed with phosphoric acid at a range of concentrations at 50°C for 60 min, separated by draining the excess liquid through a crude sieve and fed into the Cambi pilot scale 30 L steam explosion rig (Cambi, Norway) (Figure 1). The reactor was charged to the requisite pressure with steam, maintained for the desired residence time followed by rapidly raising the pressure to 15 bar(g) prior to opening the release valve to maintain consistent explosive decompression. Following SE-PT, the material was cooled to below 50°C, water-soluble carbohydrates (WSC) were recovered from the solids following addition of 1 L water at 50°C. The resulting pulp was separation into solid and liquid fractions by filtration through a muslin cloth. The severity factor was calculated using the Overend et al. (1987) method Equation (1).
Where t is the residence time in minutes, T is the temperature of pre-treatment, 100 is the reference temperature and 14.75 is the arbitrary constant ω which is the activation energy from first order kinetics (Pedersen and Meyer, 2010).
Concentration of Steam Exploded Hydrolysate
The wet exploded material was then screw pressed (CP4 Screw press, Vincent Corporation, Tampa, Florida) at 2 bar(g) to separate solids and hydrolysate. Subsequently fiber was washed through a counter current system to extract majority of water soluble carbohydrates. The entire process was repeated until ~1,000 L of hydrolysate was available, which was subsequently concentrated up. Concentration was adapted from Murthy et al. (2005) briefly; centrifugation through disk stack (Centrimax, Cologne, Germany), before being filtered through 100 kD membrane filter (Axium Process Ltd, Swansea, UK), before finally being concentrated through reverse osmosis (Reverse osmosis plant, Axium Process Ltd, Swansea, UK). Concentration required minimal pH adjustment (NH4OH) to ensure hydrolysate remained above pH 2 to allow RO membrane to operate within bounds.
Bioconversion of Hydrolysates
SE-PT hydrolysates for L16 experiments 13, 13, 13, 14 for WS, CS, M, W respectively were preliminarily analyzed in 250 mL Erlenmeyer flasks for bioconversion to xylitol. Schefferomyces shehatae strain Y6603 was inoculated in YEP medium; 2% peptone, 1% yeast extract, 0.5% glucose, 3% xylose, 0.05% KH2PO4, 0.05% MgSO4, 0.02% MnSO4H2O, 0.02% ZnSO4. (all amounts in % w/v).After 48 h growth the inoculum was used for bioconversions at 0.04 g L−1 cell dry weight. For bioconversion a 10x concentrated above media (10 mL) was added to 90 mL of each hydrolysate to attain the % w/v levels shown above with glucose and xylose excluded. Fermentations were run for 48 h with time points taken every 24 h.
Carbohydrate Analysis
SE-PT hydrolysate was cooled to room temperature and stored until required at −20°C. Using NREL laboratory analytical procedures carbohydrate content was quantified in both the fiber and hydrolysates fractions (Sluiter et al., 2006, 2008). Briefly, 300 mg (DW) freeze dried feedstock was added to 3 mL 72% H2SO4 and mixed at 30°C for 60 min, diluted to 4% v/v H2SO4 by the addition of 84 mL deionized water, autoclaved at 121°C for 60 min, cooled to room temperature and neutralized to pH 6 with CaCO3. To quantify the monomer to polymer ratio, liquid hydrolysate from SE-PT was acid hydrolyzed by adding 34.8 μL of 72% H2SO4 to 1 mL of hydrolysate, followed by autoclaving and neutralization as described above.
High Performance Liquid Chromatography
Polymeric, oligomeric and monomeric carbohydrates, and inhibitory compound concentrations were quantified using three HPLC analytical techniques. Monomeric carbohydrates were quantified using calibrated standard curves of arabinose, galactose, glucose, xylose, mannose, fructose ranging from 100 to 0.6 μg mL−1 using a Dionex High Performance Anion-Exchange Chromatography with Pulsed Amperometer Detection (HPAE-PAD) HPLC system. Samples were centrifuged for 5 min at 14,000 rpm, the supernatant removed, diluted to within the range of the standard curve, filter sterilized through Sartorius Ministart 0.2 μm syringe filter (Fisher scientific) into 0.6 mL vials with pre-cut septum (Thermofisher) with 25 μL of each sample injected onto a CarboPac SA10 column, 250 mm long × 4 mm internal diameter and eluted with an isocratic mobile phase of 1 mM KOH at 1.5 mL min−1 (Basumallick and Rohrer, 2015).
Quantification of organic acids was performed as in Bryant et al. (2011) using a JASCO HPLC system with a Aminex HPX-87C column, 300 mm long × 7.8 mm internal diameter, connected to a refractive index detector (RID) (Shodex RI-71, Showa Denko Europe GmbH, Munich, Germany) with analytes eluted at 0.6 mL min−1 using a 5 mM H2SO4 isocratic mobile phase and 5 mM crotonic acid as an internal standard. Samples were prepared as above with 25 μL of sample quantified against calibrated standard curves formic acid and acetic acid ranging from 100 to 0.625 mg mL−1.
Furfural and 5-hydroxymethylfurfural degradation products were quantified on the JASCO HPLC as above by injecting 20 μL of sample onto Aminex HPX-87H, 300 mm long with 7.8 mm internal diameter. Furfural and HMF were run as calibrated standard curves from 5 to 0.3 mg mL−1 (Sluiter et al., 2006).
Xylitol was analyzed on HPLC using JASCO HPLC system with a Aminex HPX-87H column, 300 mm long × 7.8 mm internal diameter, connected to a refractive index detector (RID) (Shodex RI-71, Showa Denko Europe GmbH, Munich, Germany) with analytes eluted at 0.6 mL min−1 using a 5 mM H2SO4 isocratic mobile phase. Samples were quantified against calibrated standard curve for xylitol ranging from 10 to 0.625 mg mL−1.
Results and Discussion
Compositional Analysis
The chemical composition of each feedstock prior to SE-PT are described in Table 2 and were found to be in broad agreement with previously published data (Thomsen and Schmidt, 1999; Sun et al., 2005; Maity, 2014; Swiatek et al., 2014). Lignin was highest in Miscanthus and willow similar to previously published reports (Fahmi et al., 2008; Hu and Ragauskas, 2012) while the xylose concentration was lowest in willow due to its higher lignin content (Fahmi et al., 2008), and highest in wheat straw.
Carbohydrate Release Following SE-PT
Pressure, time, acid loading and substrate weight were all shown to alter the concentration of xylose released from each feedstock (Table 3). Due to the scale at which experiments were being investigated, duplicates were run to ascertain the variation in soluble xylose release from each feedstock following each treatment. A wide range of levels for each factor was investigated aiming to achieve minimal inhibitor production whilst still maximizing xylose release. The highest extraction of soluble xylose for W, CS, M, WS were; 133 mg g−1 DM, 265 mg g−1 DM, 197 mg g−1 DM, and 284 mg g−1 DM respectively (Figure 2). The xylose yield from corn stover and wheat straw was in agreement with the literature (Ballesteros et al., 2006; Fang et al., 2011; Pang et al., 2013), however in these reports, values were obtained using sulphuric acid to catalyze the release of carbohydrates or integrated microwave assistance, both of which increase the capital cost of processing. The variation seen across the experiments (Figures 2, 3)as well as that within duplicates indicate inherent variation of different process parameters experienced, with Auxenfans et al. (2017) finding a 3–26% variation in hemicellulose release within replicates across different combined severity factor experiments. This is exemplified in the optimal conditions of 12 bar(g), 3 min, 500 g, 1.2% w/v for experiment 13 where the error bars are smaller than many of the other high releasing experiments. For industrial scale production the process is required to be robust and consistent with (Figure 2) data highlighting the limitations for industrial use outside of the narrow operating window for SE-PT.
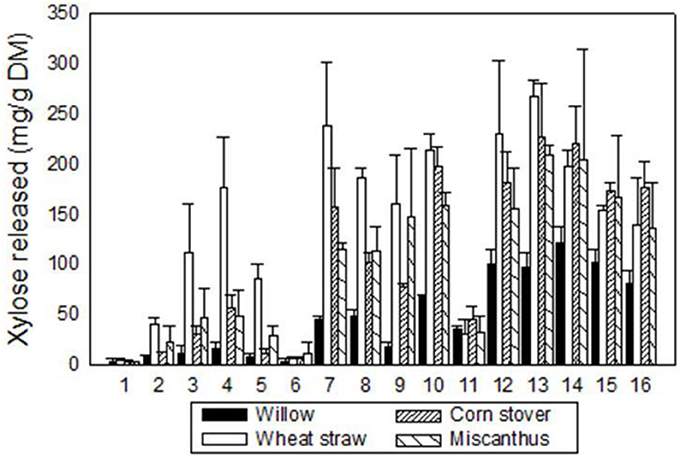
Figure 2. Concentration of xylose released (mg g−1 DM) through steam explosion pretreatment for four feedstock using Taguchi L16 DoE methodology (Black, willow; White, wheat straw; cross hatches left, corn stover; cross hatches right, Miscanthus). Vertical bars represent the standard deviation of the mean n = 2.
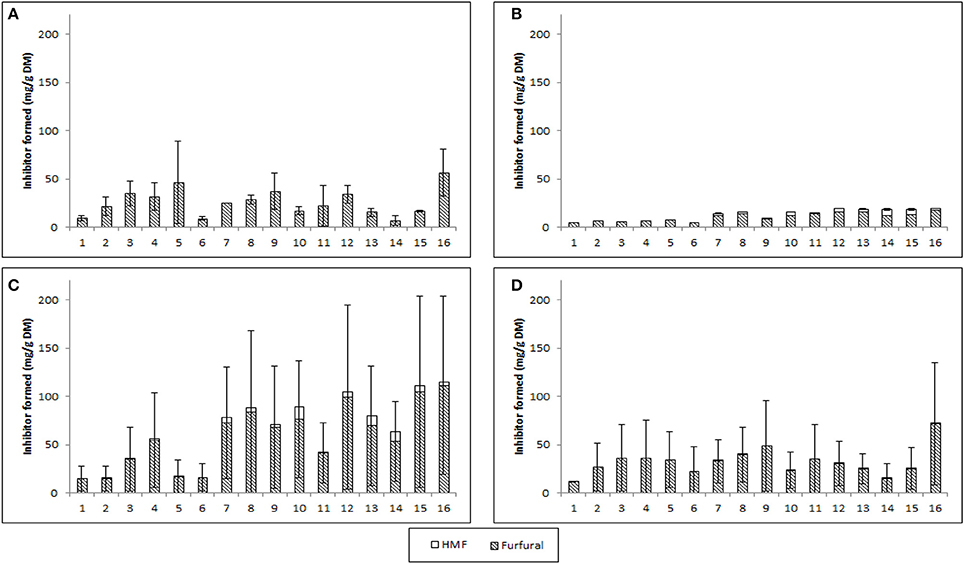
Figure 3. Furfural and 5-Hydroxymethyl-furfural produced through SE-PT with feedstock [(A) wheat straw, (B) Willow, (C) corn stover, (D) Miscanthus × giganteus]. Vertical bars represent the standard deviation of the mean n = 2.
Despite there being a wealth of research on steam explosion, the vast majority of research uses steam explosion as a pre-treatment prior to enzymatic hydrolysis to produce both C5 and C6 sugars for bioethanol production. Reports solely focussing on hemicellulose extraction into hydrolysates is not highly reported possibly due to the question of addressing the resulting cellulosic pulp. However, an abundance of literature exists on uses for the remaining fibrous material, for example as substrate for biogas production or enzymatic hydrolysis for bioethanol generation (Horn et al., 2011; López-Linares et al., 2015; Arenas-Cárdenas et al., 2017; Lizasoain et al., 2017). Production of biogas with the remaining pulp could be integrated with a commercial xylitol production plant with the advantages of having a rapid integrated energy supply as SE-PT has been shown to increase the rate of biogas production (Horn et al., 2011).
The broad range of levels resulting in a wide array of severity factors (Table 1) were chosen to assess maximum xylose release whilst decreasing the fermentation inhibitors, several publications have discussed how high severity factors of SE-PT will lead to increased inhibitor generation (Iroba et al., 2014; Guerrero et al., 2017). The data in Figure 2 indicate that low severity factors result in low xylose release. Castro et al. (2014) achieved ~66% xylose release from Eucalyptus chips utilizing 0.8% w/w phosphoric acid, 200°C for 5 minu residence time whilst Negro et al. (2014) used 1% w/w phosphoric acid at 175°C for 10 min to achieve 80% monomeric xylose extraction of olive tree pruning's. Castro et al. (2014) had high levels of inhibitors formed for high xylose release and Negro et al. (2014) generated double the furfural concentration by increasing the temperature from 175 to 195°C with only moderate xylose increases. However, both were aimed at glucose extraction for fuel ethanol production not maximum xylose release. Zhang et al. (2014) has investigated steam explosion for hemicellulose release using a screw steam explosive extruder to extract almost 90% hemicellulose from corn cobs using sulphuric acid at 9 mg g−1 dried corn cobs, 1.55 MPa (201°C) for 5.5 min. This work is informative however it used sulphuric acid which increases capital cost and requires the hydrolysate to be detoxified with activated carbon, cation and anion resins. These papers indicate a range of feedstock and the SE-PT parameters required to hydrolyze hemicellulose confirming that optimization of factor levels is required and that each feedstock is independent.
Inhibitor Generation
The production of the inhibitors HMF (from glucose) and furfural (from xylose) was investigated and the combined level of furfural and HMF released through steam explosion of biomass is presented in Figure 3. HMF was not detected within WS, low levels were detected in M and W (<6 mg g−1 DM), and appreciable levels within the CS hydrolysate (~15 mg g−1, ~1 g L−1). The highest accumulation of furfural and HMF was found to be 110 mg g−1 DM in CS corresponding to ~9.6 g L−1 which would be detrimental to bioconversion (van der Pol et al., 2014; Figure 3C). For WS, M and W the maximum individual inhibitor accumulation were below 4 g L−1 for HMF and furfural, which is important as up to 4 g L−1 of furfural or HMF has been shown to not inhibit microbial growth (Geddes et al., 2011; Mattam et al., 2016; Wang et al., 2016). Previous publications already mentioned have a range of inhibitors concentrations from 7.5 to 45.8 g kg−1 (Castro et al., 2014) 3.62 to 5.65 g L−1 (Negro et al., 2014) and 3.77 g Kg−1 (Zhang et al., 2014) for SE-PT biomass. Negro et al. (2014) required hydrolysate detoxification prior to fermentation, whilst Castro et al. (2014) utilized an inhibitor resistant E coli strain for bioethanol fermentation. As already mentioned above (Zhang et al., 2014) used activated carbon with cation and anion resin before xylose crystallization. Optimization of both xylose release and reduced inhibitor production is required to ensure that scale-up with reduced process steps could be achieved.
Taguchi DoE L16 Analysis
The influence of the selected factors on the pre-treatment process is given in Table 3 along with the average effect of a factor at each level. Table 3 also shows the nature of the trend for each factor across the four levels. The main effect indicates the variation in results for the shift in factor levels, which indicates the sensitivity of the factor performance, i.e., L2-L1. The larger the variation in the levels, the more the factor is influencing the process.
Through Taguchi DoE analysis, each feedstock was shown to be influenced to a differing degree (percent influence) by factors affecting xylose release (Table 4). The factor exerting the greatest influence on the process can be identified in the last column of the ANOVA table (Table 4). The indication from ANOVA is that each feedstock was influenced to a different extent. For WS, acid concentration had a much higher influence (59.8%) as an individual factor on xylose release while pressure only had a 22% influence. This is in contrast to the other three feedstock where pressure had an effect of 66.2, 70.2, and 77.1% respectively as an individual factor. The individual factors of time, acid and substrate load had minor influence on the xylose release (Table 4).
Interaction of the factors for each feedstock is shown in Table 5. The interaction between factors illustrates how in combination the factors involved can influence the pre-treatment process through either synergistic or antagonistic effects. Factor interactions can therefore be considered to have a similar effect to those of individual factors, thereby contributing positively or negatively on the net effect of the factors in the pre-treatment process. The magnitude of these interactions are calculated as a severity index (SI) percentage, which should not be confused with the severity factor used to describe pre-treatment processes. Time x substrate load and acid x substrate load were found to be most influential exerting the highest effect on the pre-treatment process for each feedstock. Pressure had high influence as an individual factor was not influential in combination with any other factor.
The quantity of lignin acting as a barrier to lignocellulose hydrolysis and the ability to disrupt the lignin structure are key factors recognized for reducing biomass recalcitrance (Behera et al., 2014; Rabemanolontsoa and Saka, 2016). Compositional analysis of each feedstock found that wheat straw had the highest levels of carbohydrates and the lowest level of lignin (Table 2). The low lignin level was likely to be a key reason why acid exerted the highest influence on xylose release from WS (Davison et al., 2006), whereas pressure played an important role in the case for CS, M and W. The degradation of the lignin matrix is also dependent upon the lignin monomeric composition with previous studies indicating that hardwoods (e.g., willow) have a higher coniferyl and sinapyl residue ratio, whereas monocot's have a greater level of p-coumaryl residue (Davison et al., 2006; Cavka, 2013; Gu, 2013). The additional pressure being applied to CS, M and W may enhance the catalytic effect by ensuring deeper pore penetration of each feedstock, however the resulting increase in temperature can also lead to sugar degradation.
There are few previous publications exclusively discussing xylose release from steam explosion for subsequent bioconversion to renewable commodities (Tomás-Pejó et al., 2008; Misra et al., 2013; Wang et al., 2013, 2015; Fan et al., 2014; Zhang et al., 2014), with all but Tomás-Pejó et al. (2008) using sulphuric acid for acid impregnation. Tomás-Pejó et al. (2008) only used water as the catalyst for SE-PT for subsequent enzymatic hydrolysis. Studies by Wang et al. (2013) most closely matched the current work in scale using 1 kg dried material and a 10 L batch reactor. These authors found soaking time of 6 h, 0.3% sulphuric acid concentration at a liquid to corncob ratio of 3:1 with SE-PT conditions of 200°C and 5 min residence time gave the optimal conditions for xylose release, however this achieved only 23.2% xylose mass recovery. Furthermore, this material required detoxification prior to bioconversion due to the level of inhibitors released through SE-PT.
Analysis of variance revealed that all four factors had a significant effect on the soluble xylose released from each feedstock (P < 0.01). ANOVA was used to determine which levels of each factor in combination will yield the highest xylose concentration and predict the xylose concentration achieved from each of these levels. All four ANOVA analyses determined that the optimum conditions were 12 bar(g), 12 min, 1.2% acid and 0.4 kg substrate (Table 6A).
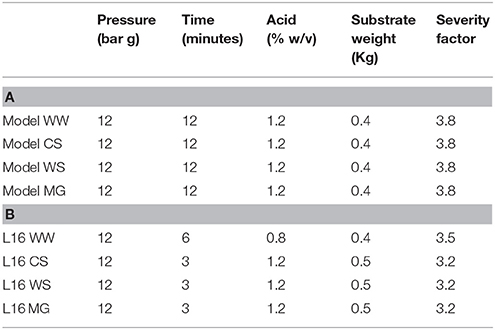
Table 6. SE Taguchi DoE model set of parameters (A) and best performing conditions from L16 experiment (B) for maximum xylose release from LCBs (WW, Willow; WS, Wheat straw; CS, Corn stover; MG, Miscanthus × giganteus).
The data presented in the L16 data set show that 1.2% w/v phosphoric acid was effective in releasing maximum xylose from all feedstock and was specified in the model (Table 6). However, compared to the L16 data the model predicted 12 min residence time with a severity factor of 3.8 as optimum. The L16 used 6, 3, 3, and 3 min residence times for maximum xylose release with associated severity factors of 3.5, 3.2, 3.2, and 3.2 for W, CS, WS and MG respectively. Some of the observed variation in xylose release would appear to be due to the inherent variation of steam explosion at pilot scale, despite attempts to minimize this by warming up the reaction vessel prior to and cleaning in between each treatment. The error bars displayed in Figure 2 highlight the variation (standard deviation) between duplicate explosions, suggesting that day to day variations of steam explosion at this scale may be the result.
Overall Evaluation Criteria Modeling
Knowing that inhibitors are present in the hydrolysate an alternative model was assessed using a combination of maximum xylose release and minimum total HMF and furfural production, an overall evaluation criteria (OEC) analysis using Qualitek 4 was performed. This analysis with a 75% weighting to maximum xylose and 25% weighting to minimum total inhibitor provided a further set of optimum explosion parameters (Table 7).
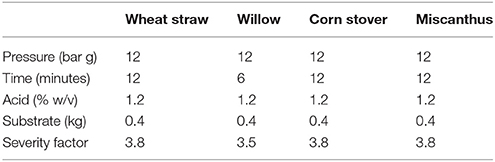
Table 7. Optimum levels of factors from OEC for maximum xylose release and minimum furfural release through SE-PT.
The OEC analysis (Table 8) indicated that the monocots; M, WS, and CS all required the same optimum conditions as for maximum xylose release (Table 6) whilst willow, an arboreal dicot, would require lower residence time to reduce the amount of furfural produced through the SE-PT. This was surprising considering the fact that W produced the lowest levels of inhibitor observed in the L16 data (Figure 3), but equally produced the lowest levels of xylose, Figure 2. The OEC model parameters were used in SE-PT of all feedstock to assess the models accuracy.
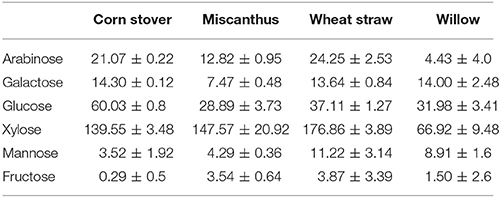
Table 8. Taguchi model validation for maximum xylose release (mg g−1 DM) of four feedstock, Model, predicted levels for factors from Taguchi OEC model n = 3.
Taguchi Validation Experiments
The concentration of monomeric carbohydrates, released into the hydrolysate during steam explosion, revealed that the predicted model parameters gave lower values of all carbohydrates compared to the L16 best performing SE-PT (Table 8 and Figure 2). A maximum xylose release (up to 94%) achieved within L16 experimental conditions outperforms that observed in the OEC model conditions, with considerable losses apparent from each feedstock, up to 50% lower xylose release than L16. The indication here is that the increase in severity factor from the L16 to the model has resulted in a decrease in xylose released. Table 6 illustrates the increase in severity factor from L16 to model with the lower severity experiments yielding greater xylose levels than higher severities. Previous authors have noted that increased severity factors led to reduction in carbohydrate concentrations and greater inhibitor levels (Castro et al., 2014; Iroba et al., 2014; Sharma et al., 2015). It may be unsurprising that the model prediction of a 4-fold increase in residence time for CS, M and WS resulted in lowering xylose recovery as the increased length of exposure of labile pentose monomers to acid at high temperature is known to result in degradation to furfural and subsequent decomposition to formic acid (Larsson et al., 1999). Indeed taking together the xylose released and inhibitor formed from WS, 65% of xylose was recovered and almost 300% more inhibitor was produced when compared to run 13 of the L16 experiment (Figures 2–4). In isolation, time was shown to exert a minor influence on xylose recovery from all feedstock (Table 4). However, the severity index of interacting factors revealed that time x substrate weight applied an influence of between 80 and 96.4% on the generation of xylose for each feedstock (Table 5) demonstrating a strong interaction with biomass load. Zhao et al. (2013) also noticed an interactive influence in assessment of sewage sludge for solid fuel production utilizing an L16 orthogonal array with steam explosion. The orthogonal array could not account for the interaction of factors; pressure and residence time, and the fluctuation in moisture content for a 15 s change in residence time was likely due to the interaction of these factors. In light of these findings the OEC model was modified by reducing the residence time to 6 min and increasing the biomass loading to 1.5 kg of WS, resulting in 90% recovery of the original xylose in the hydrolysate.
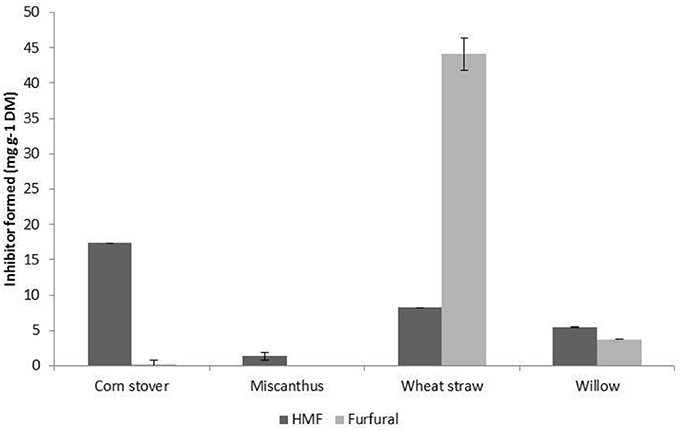
Figure 4. Inhibitor formation (mg g−1 DM) following Taguchi OEC model validation SE-PT of four feedstock. Vertical bars represent the standard deviation of the mean n = 2.
The lower xylose release from all feedstock is attributed to the increased residence time offered by the OEC model and the lower levels of inhibitors in CS and M suggest that the increase in residence time has led to further decomposition of furfural and HMF to levulinic acid and/or formic acid and that this decomposition occurred in a feedstock dependent manner (Mussatto and Roberto, 2004; van der Pol et al., 2014). The inhibitor data for wheat straw supported those of xylose release in that the SE-PT severity was too harsh resulting in degradation into inhibitory compounds. Specifically this appears to have resulted from the increased residence time and reduction in biomass loading as dictated by the Taguchi OEC model.
The Taguchi methodology has enabled 256 experiments, if performed through full factorial assessment, to be reduced down to 16 experiments. Using this methodology each of the four feedstock gave high xylose yields (>75% total xylose), all of which successfully had preliminary single flask assessments for bioconversion to produce xylitol (Figure 5). However, for process optimization the wide range of levels on each factor resulted in a broad range of xylose release ~10 mg g−1 DM to ≥197 mg g−1 DM for all monocots, Figure 2. The xylose release from willow was substantial ~75% (Figure 2) however, the amount in the initial material was much lower at 15.7% (Table 2) than found in monocots, consequently further assessment of willow was discounted. For the three monocots the furfural and HMF produced also showed considerable variation (Figure 3) from ~5 mg g−1 DM to ~110 mg g−1 DM. The extensive range of both xylose and inhibitor levels suggest that the factor levels were too broad affecting the reliability of the Taguchi OEC model.
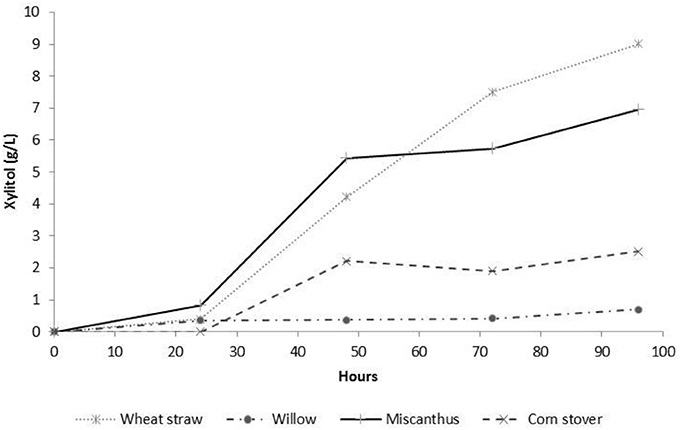
Figure 5. Flask bioconversion of L16 SE-PT feedstock hydrolysate without detoxification for xylitol production utilizing Schefferomyces shehatae Y6603 strain n = 1.
Taguchi DoE requires a systematic approach to understanding: measuring inputs and outputs, identifying and understanding the noise factors within the system, choosing appropriate control factors and levels, and selection of a suitable design (Rao et al., 2008). Options to improve the current approach would be to reduce the range of levels for each factor and to narrow the parameter range, increase the number of replicates to decrease the variation within the experiments, or to conduct a fine tuning Taguchi methodology model. Previous research has indicated that utilizing an L16 to do an initial optimization of methane production can be further improved by fine tuning these results via an L4 orthogonal array (Adu-Gyamfi et al., 2012). Teng and Xu (2008) utilized Taguchi for initial optimization with RSM used subsequently to further enhance lipase yield. Had the current investigation been carried out via RSM many more experiments would have been required, for example 4 factors at 3 levels requires 27 experiments, an increase of 50% on Taguchi DoE. Both of these examples indicate the importance that Taguchi methodology can have for enhancing production whilst reducing experiments required. In future a L4 Taguchi orthogonal array could be performed to finalize maximal xylose release from WS whilst minimizing formation of fermentation inhibitors. However, the increase in yield is anticipated to be modest compared to that presented here.
Based upon these data and subsequent modification of the OEC model an optimized scalable process was developed that enabled 90% xylose recovery from WS using the parameters of 1.5 kg DM, 1.2% w/v H3PO4, 12 bar(g) and a residence time of 6 min. All four feedstock generated hydrolysates indicated bioconversion of xylose to xylitol (data not shown) however to assess a pilot scale bioconversion system a single feedstock was chosen; CS was discounted due to the high inhibitor loadings seen in Figure 3. While Miscanthus performed well in terms of xylose release and inhibitor production, the low availability of this feedstock globally precluded use in subsequent studies. Table 6 illustrates that the monocotyledonous material all required the same SE-PT parameters to yield the highest concentration of xylose, indicating that a biorefinery processing plant could equally utilize any of these biomass for SE-PT toward xylitol bioconversion. These results enable a broader spectrum of locals for a biorefinery to be situated for supply of enough biomass for production. SE-PT was performed on 10's of kg of wheat straw to produce a total yield of ~1,000 L of hydrolysate for scale-up assessment of a bioconversion system for xylitol production up to 170 L fermentation.
Conclusion
This work helps fill in the knowledge void in the production of xylose following pre-treatment of lignocellulosic material at pilot scale. Agricultural residues, wheat straw, corn stover, and dedicated energy crops, Miscanthus, willow, could all be used as biomass resources for xylose release for xylitol production. Using a steam explosion pre-treatment of 12 bar(g), 3 min, 1.2% phosphoric acid, and 500 g substrate attained a yield of up to 94% release of initial xylose into hydrolysates. The use of Taguchi method DoE reduced the number of explosions from 256 per feedstock to 16 and gave a high yielding set of parameters. Steam explosion pre-treatment of wheat straw for xylose release gave high yields with low levels of fermentation inhibitors and subsequent fermentation assessment of the hydrolysates has proved these are non-toxic and allow for xylitol generation (Figure 5). However, the OEC Taguchi model predicted optimum conditions that were not confirmed by experimental data, yielding lower xylose levels than those seen via L16 experiments. The utilization of Taguchi methodology DoE is based upon knowledge of the system being investigated. Methods to improve model outputs include narrow levels for factors via in-depth knowledge or preliminary investigation and use of multiple orthogonal arrays to initially limit the levels and factors before fine tuning the model with a tapered orthogonal array and reduced levels.
The results presented indicated that the Taguchi orthogonal experimental design did reduce experimental numbers, costs and time and that modification of the OEC model produced a scalable SE-PT for recovering 90% of the original xylose in a fermentable form.
Author Contributions
DB, JG, SR, and AW conceived the project. SR and DB designed the experiments. DW performed the experiments. DW, SR, and DB performed analysis and data interpretation. DW and AS wrote the manuscript with all authors contributing to manuscript editing and revisions.
Conflict of Interest Statement
The authors declare that the research was conducted in the absence of any commercial or financial relationships that could be construed as a potential conflict of interest.
Acknowledgments
The authors acknowledge EIT Climate-KIC for funding the project ADMIT BIOSUCCINOVATE, BBSRC (BB/J00442/1), and thank staff at the BEACON Biorefining Centre of Excellence Facility (c80851), IBERS, Aberystwyth University for sharing their expertise and steam explosion facilities.
References
Adu-Gyamfi, N., Ravella, S. R., and Hobbs, P. J. (2012). Optimizing anaerobic digestion by selection of the immobilizing surface for enhanced methane production. Bioresour. Technol. 120, 248–255. doi: 10.1016/j.biortech.2012.06.042
Aggarwal, A., Singh, H., Kumar, P., and Singh, M. (2008). Optimizing power consumption for CNC turned parts using response surface methodology and Taguchi's technique—a comparative analysis. J. Mater. Process. Technol. 200, 373–384. doi: 10.1016/J.JMATPROTEC.2007.09.041
Alvira, P., Tomás-Pejó, E., Ballesteros, M., and Negro, M. J. (2010). Pretreatment technologies for an efficient bioethanol production process based on enzymatic hydrolysis: a review. Bioresour. Technol. 101, 4851–4861. doi: 10.1016/j.biortech.2009.11.093
Arenas-Cárdenas, P., López-López, A., Moeller-Chávez, G. E., and León-Becerril, E. (2017). Current pretreatments of lignocellulosic residues in the production of bioethanol. Waste Biomass Valorization 8, 161–181. doi: 10.1007/s12649-016-9559-4
Auxenfans, T., Crônier, D., Chabbert, B., and Paës, G. (2017). Understanding the structural and chemical changes of plant biomass following steam explosion pretreatment. Biotech. Biofuels 10:36. doi: 10.1186/s13068-017-0718-z
Ballesteros, I., Negro, M. J., Oliva, J. M., Cabañas, A., Manzanares, P., and Ballesteros, M. (2006). Ethanol production from steam-explosion pretreated wheat straw. Appl. Biochem. Biotech. 129–132, 496–508. doi: 10.1385/ABAB:130:1:496
Basumallick, L., and Rohrer, J. (2015). Determination of Carbohydrates in Acid Hydrolysates of Wood. Available online at: https://appslab.thermofisher.com/App/3799/determination-wood-carbohydrates
Behera, S., Arora, R., Nandhagopal, N., and Kumar, S. (2014). Importance of chemical pretreatment for bioconversion of lignocellulosic biomass. Renew. Sustain. Energy Rev. 36, 91–106. doi: 10.1016/j.rser.2014.04.047
Box, G. E. P., and Hunter, J. S. (1957). Multi-factor experimental designs for exploring response surfaces. Ann. Math. Stat. 28, 195–241. doi: 10.1214/aoms/1177707047
Bryant, D. N., Firth, E., Kaderbhai, N., Taylor, S., Morris, S. M., Logan, D., et al. (2013). Monitoring real-time enzymatic hydrolysis of Distillers Dried Grains with Solubles (DDGS) by dielectric spectroscopy following hydrothermal pre-treatment by steam explosion. Bioresour. Technol. 128, 765–768. doi: 10.1016/j.biortech.2012.09.021
Bryant, D. N., Morris, S. M., Leemans, D., Fish, S. A., Taylor, S., Carvell, J., et al. (2011). Modelling real-time simultaneous saccharification and fermentation of lignocellulosic biomass and organic acid accumulation using dielectric spectroscopy. Bioresour. Technol. 102, 9675–9682. doi: 10.1016/j.biortech.2011.07.084
Castro, E., Nieves, I. U., Mullinnix, M. T., Sagues, W. J., Hoffman, R. W., Fernández-Sandoval, M. T., et al. (2014). Optimization of dilute-phosphoric-acid steam pretreatment of Eucalyptus benthamii for biofuel production. Appl. Energy 125, 76–83. doi: 10.1016/j.apenergy.2014.03.047
Cavka, A. (2013). Biorefining of Lignocellulose: Detoxification of Inhibitory Hydrolysates and Potential Utilization of Residual Streams for Production of Enzymes. Umeå University. Available online at: http://www.diva-portal.org/smash/record.jsf?pid=diva2%3A661377&dswid=5386
Clifton-Brown, J., Hastings, A., Mos, M., McCalmont, J. P., Ashman, C., Awty-Carroll, D., et al. (2017). Progress in upscaling Miscanthus biomass production for the European bio-economy with seed-based hybrids. GCB Bioenergy 9, 6–17. doi: 10.1111/gcbb.12357
Davison, B. H., Drescher, S. R., Tuskan, G. A., Davis, M. F., and Nghiem, N. P. (2006). “Variation of S/G ratio and lignin content in a populus family influences the release of xylose by dilute acid hydrolysis,” in Twenty-Seventh Symposium on Biotechnology for Fuels and Chemicals, ABAB Symposium, eds J. D. McMillan, W. S. Adney, J. R. Mielenz, and K. T. Klasson (Totowa, NJ: Humana Press), 427–435.
Ding, D., Zhou, X., You, T., Zhang, X. X., Zhang, X., and Xu, F. (2018). Exploring the mechanism of high degree of delignification inhibits cellulose conversion efficiency. Carbohydr. Polym. 181, 931–938. doi: 10.1016/j.carbpol.2017.11.057
Eklund, R., Galbe, M., and Zacchi, G. (1995). The influence of SO2 and H2SO4 impregnation of willow prior to steam pretreatment. Bioresour. Technol. 52, 225–229. doi: 10.1016/0960-8524(95)00042-D
Fahmi, R., Bridgwater, A. V., Donnison, I., Yates, N., and Jones, J. M. (2008). The effect of lignin and inorganic species in biomass on pyrolysis oil yields, quality and stability. Fuel 87, 1230–1240. doi: 10.1016/j.fuel.2007.07.026
Fan, X., Cheng, G., Zhang, H., Li, M., Wang, S., and Yuan, Q. (2014). Effects of acid impregnated steam explosion process on xylose recovery and enzymatic conversion of cellulose in corncob. Carbohydr. Polym. 114, 21–26. doi: 10.1016/j.carbpol.2014.07.051
Fang, H., Deng, J., and Zhang, X. (2011). Continuous steam explosion of wheat straw by high pressure mechanical refining system to produce sugars for bioconversion. BioResources 6, 4468–4480. doi: 10.15376/biores.6.4.4468-4480
Geddes, C. C., Mullinnix, M. T., Nieves, I. U., Peterson, J. J., Hoffman, R. W., York, S. W., et al. (2011). Simplified process for ethanol production from sugarcane bagasse using hydrolysate-resistant Escherichia coli strain MM160. Bioresour. Technol. 102, 2702–2711. doi: 10.1016/j.biortech.2010.10.143
Guerrero, A. B., Ballesteros, I., and Ballesteros, M. (2017). Optimal conditions of acid-catalysed steam explosion pretreatment of banana lignocellulosic biomass for fermentable sugar production. J. Chem. Tech. Biotech. 92, 2351–2359. doi: 10.1002/jctb.5239
Harmsen, P., Huijgen, W., Bermudez, L., and Bakker, R. (2010). Literature Review of Physical and Chemical Pretreatment Processes for Lignocellulosic Biomass. Available online at: http://www.ecn.nl/docs/library/report/2010/e10013.pdf
Haughton, A. J., Bond, A. J., Lovett, A. A., Dockerty, T., Sünnenberg, G., Clark, S. J., et al. (2009). A novel, integrated approach to assessing social, economic and environmental implications of changing rural land-use: a case study of perennial biomass crops. J. Appl. Ecol. 46, 315–322. doi: 10.1111/j.1365-2664.2009.01623.x
Heaton, E. A., Dohleman, F. G., and Long, S. P. (2008). Meeting US biofuel goals with less land: the potential of Miscanthus. Global Change Biol. 14, 2000–2014. doi: 10.1111/j.1365-2486.2008.01662.x
Hodgson, E., Lewys-James, A., Rao Ravella, S., Thomas-Jones, S., Perkins, W., and Gallagher, J. (2016). Optimisation of slow-pyrolysis process conditions to maximise char yield and heavy metal adsorption of biochar produced from different feedstocks. Bioresour. Technol. 214, 574–581. doi: 10.1016/j.biortech.2016.05.009
Horn, S. J., Estevez, M. M., Nielsen, H. K., Linjordet, R., and Eijsink, V. G. (2011). Biogas production and saccharification of Salix pretreated at different steam explosion conditions. Bioresour. Technol 102, 7932–7936. doi: 10.1016/j.biortech.2011.06.042
Hu, F., and Ragauskas, A. (2012). Pretreatment and Lignocellulosic Chemistry. Bioenergy Res. 5, 1043–1066. doi: 10.1007/s12155-012-9208-0
Iroba, K. L., Tabil, L. G., Sokhansanj, S., and Dumonceaux, T. (2014). Pretreatment and fractionation of barley straw using steam explosion at low severity factor. Biomass Bioenergy 66, 286–300. doi: 10.1016/j.biombioe.2014.02.002
Larsson, S., Palmqvist, E., Hahn-Hägerdal, B., Tengborg, C., Stenberg, K., Zacchi, G., et al. (1999). The generation of fermentation inhibitors during dilute acid hydrolysis of softwood. Enzyme Microb. Technol. 24, 151–159. doi: 10.1016/S0141-0229(98)00101-X
Lizasoain, J., Trulea, A., Gittinger, J., Kral, I., Piringer, G., Schedl, A., et al. (2017). Corn stover for biogas production: effect of steam explosion pretreatment on the gas yields and on the biodegradation kinetics of the primary structural compounds. Bioresour. Technol. 244, 949–956. doi: 10.1016/j.biortech.2017.08.042
López-Linares, J. C., Ballesteros, I., Tourán, J., Cara, C., Castro, E., Ballesteros, M., et al. (2015). Optimization of uncatalyzed steam explosion pretreatment of rapeseed straw for biofuel production. Bioresour. Technol. 190, 97–105. doi: 10.1016/j.biortech.2015.04.066
Maity, S. K. (2014). Opportunities, recent trends and challenges of integrated biorefinery: Part I. Renew. Sustain. Energy Rev. 43, 1427–1445. doi: 10.1016/j.rser.2014.11.092
Martijn, V. (2015). A Method for Standardized Biomass Characterization and Minimal Biomass Quality Requirements for Each Biomass Conversion Technology. Enschede. Available online at: http://s2biom.alterra.wur.nl/doc/S2BIOM_D2.1_method_standardized_biomass_characterisation__quality_requirements_for_conversion_technologies.pdf
Mason, W. H. (1926). Process and apparatus for disintegration of wood and the like US Patent. 1, 578–609.
Mattam, A. J., Kuila, A., Suralikerimath, N., Choudary, N., Rao, P. V., and Velankar, H. R. (2016). Cellulolytic enzyme expression and simultaneous conversion of lignocellulosic sugars into ethanol and xylitol by a new Candida tropicalis strain. Biotech. Biofuels 9:157. doi: 10.1186/s13068-016-0575-1
Misra, S., Raghuwanshi, S., and Saxena, R. K. (2013). Evaluation of corncob hemicellulosic hydrolysate for xylitol production by adapted strain of Candida tropicalis. Carbohydr. Polym. 92, 1596–1601. doi: 10.1016/j.carbpol.2012.11.033
Mohamad, N. L., Mustapa Kamal, S. M., and Mokhtar, M. N. (2015). Xylitol biological production: a review of recent studies. Food Rev. Int. 31, 74–89. doi: 10.1080/87559129.2014.961077
Murthy, G. S., Sridhar, S., Shyam Sunder, M., Shankaraiah, B., and Ramakrishna, M. (2005). Concentration of xylose reaction liquor by nanofiltration for the production of xylitol sugar alcohol. Sep. Purif. Technol. 44, 221–228. doi: 10.1016/j.seppur.2005.01.009
Mussatto, S. I., and Roberto, I. C. (2004). Alternatives for detoxification of diluted-acid lignocellulosic hydrolyzates for use in fermentative processes: a review. Bioresour. Technol. 93, 1–10. doi: 10.1016/j.biortech.2003.10.005
Negro, M. J., Alvarez, C., Ballesteros, I., Romero, I., Ballesteros, M., Castro, E., et al. (2014). Ethanol production from glucose and xylose obtained from steam exploded water-extracted olive tree pruning using phosphoric acid as catalyst. Bioresour. Technol. 153, 101–107. doi: 10.1016/j.biortech.2013.11.079
O'Donohue, M. (2014). BIOCORE Final Publishable Summary Report. Toulouse. Available online at: http://www.biocore-europe.org/file/BIOCORE%20final%20report(1).pdf
Overend, R. P., Chornet, E., and Gascoigne, J. A. (1987). Fractionation of lignocellulosics by steam-aqueous pretreatments [and Discussion]. Philos. Trans. R. Soc. A Math. Phys. Eng. Sci. 321, 523–536. doi: 10.1098/rsta.1987.0029
Pang, F., Xue, S., Yu, S., Zhang, C., Li, B., and Kang, Y. (2013). Effects of combination of steam explosion and microwave irradiation (SE–MI) pretreatment on enzymatic hydrolysis, sugar yields and structural properties of corn stover. Indus. Crops Products 42, 402–408. doi: 10.1016/j.indcrop.2012.06.016
Pedersen, M., and Meyer, A. S. (2010). Lignocellulose pretreatment severity - relating pH to biomatrix opening. New Biotech. 27, 739–750. doi: 10.1016/j.nbt.2010.05.003
Purdy, S. J., Cunniff, J., Maddison, A. L., Jones, L. E., Barraclough, T., Castle, M., et al. (2015). Seasonal carbohydrate dynamics and climatic regulation of senescence in the perennial grass, Miscanthus. BioEnergy Res. 8, 28–41. doi: 10.1007/s12155-014-9500-2
Rabemanolontsoa, H., and Saka, S. (2016). Various pretreatments of lignocellulosics. Bioresour. Technol. 199, 83–91. doi: 10.1016/j.biortech.2015.08.029
Rao, R. S., Kumar, C. G., Prakasham, R. S., and Hobbs, P. J. (2008). The Taguchi methodology as a statistical tool for biotechnological applications: a critical appraisal. Biotech. J. 3, 510–523. doi: 10.1002/biot.200700201
Rao, R. S., Prakasham, R. S., Prasad, K. K., Rajesham, S., Sarma, P. N., and Rao, L. V. (2004). Xylitol production by Candida sp.: parameter optimization using Taguchi approach. Process Biochem. 39, 951–956. doi: 10.1016/S0032-9592(03)00207-3
Rettenmaier, N., Harter, R., Himmler, H., Keller, H., Kretschmer, W., Pajula, T., et al. (2014). Environmental Sustainability Assessment of the BIOCORE Biorefinery Concept. Heidelberg. Available online at: http://www.biocore-europe.org/file/BIOCORE_D7_5_Environmental%20assessment_2014-05-15.pdf
Ruiz, E., Cara, C., Manzanares, P., Ballesteros, M., and Castro, E. (2008). Evaluation of steam explosion pre-treatment for enzymatic hydrolysis of sunflower stalks. Enzyme Microb. Tech. 42, 160–166. doi: 10.1016/j.enzmictec.2007.09.002
Sharma, S., Kumar, R., Gaur, R., Agrawal, R., Gupta, R. P., Tuli, D. K., et al. (2015). Pilot scale study on steam explosion and mass balance for higher sugar recovery from rice straw. Bioresour. Technol. 175, 350–357. doi: 10.1016/j.biortech.2014.10.112
Sluiter, A., Hames, B., Ruiz, R. O., Scarlata, C., Sluiter, J., Templeton, D., et al. (2008). Determination of structural carbohydrates and lignin in biomass. Lab. Anal. 2011, 1–14. Available online at: https://www.nrel.gov/docs/gen/fy13/42618.pdf
Sluiter, A., Hames, B., Ruiz, R., and Scarlata, C. (2006). Determination of Sugars, Byproducts, and Degradation Products in Liquid Fraction Process Samples. Golden, CO: National Renewable Energy Laboratory. Available online at: http://www.nrel.gov/docs/gen/fy08/42623.pdf
Sørensen, A., Teller, P. J., Hilstrøm, T., and Ahring, B. K. (2008). Hydrolysis of Miscanthus for bioethanol production using dilute acid presoaking combined with wet explosion pre-treatment and enzymatic treatment. Bioresour. Technol. 99, 6602–6607. doi: 10.1016/j.biortech.2007.09.091
Sun, X. F., Xu, F., Zhao, H., Sun, R. C., Fowler, P., and Baird, M. S. (2005). Physicochemical characterisation of residual hemicelluloses isolated with cyanamide-activated hydrogen peroxide from organosolv pre-treated wheat straw. Bioresour. Technol. 96, 1342–1349. doi: 10.1016/j.biortech.2004.11.018
Swiatek, K., Lewandowska, M., Swiatek, M., Bednarski, W., and Brzozowski, B. (2014). The improvement of enzymatic hydrolysis efficiency of rape straw and Miscanthus giganteus polysaccharides. Bioresour. Technol. 151, 323–331. doi: 10.1016/j.biortech.2013.10.090
Teng, Y., and Xu, Y. (2008). Culture condition improvement for whole-cell lipase production in submerged fermentation by Rhizopus chinensis using statistical method. Bioresour. Technol. 99, 3900–3907. doi: 10.1016/j.biortech.2007.07.057
Thomsen, A. B., and Schmidt, A. S. (1999). Further Development of Chemical and Biological Processes for Production of Bioethanol : Optimisation of Pre-treatment Processes and Characterisation of Products, 1–74. Available online at: http://orbit.dtu.dk/files/7729741/ris_r_1110.pdf
Tomás-Pejó, E., Oliva, J. M., Ballesteros, M., and Olsson, L. (2008). Comparison of SHF and SSF processes from steam-exploded wheat straw for ethanol production by xylose-fermenting and robust glucose-fermenting Saccharomyces cerevisiae strains. Biotech. Bioeng. 100, 1122–1131. doi: 10.1002/bit.21849
van der Pol, E. C., Bakker, R. R., Baets, P., and Eggink, G. (2014). By-products resulting from lignocellulose pretreatment and their inhibitory effect on fermentations for (bio)chemicals and fuels. Appl. Microbiol. Biotech. 98, 9579–9593. doi: 10.1007/s00253-014-6158-9
Venkateswar Rao, L., Goli, J. K., Gentela, J., and Koti, S. (2016). Bioconversion of lignocellulosic biomass to xylitol: an overview. Bioresour. Technol. 213, 299–310. doi: 10.1016/j.biortech.2016.04.092
Wang, L., Fan, X., Tang, P., and Yuan, Q. (2013). Xylitol fermentation using hemicellulose hydrolysate prepared by acid pre-impregnated steam explosion of corncob. J. Chem. Technol. Biotechnol. 88, 2067–2074. doi: 10.1002/jctb.4070
Wang, S., He, Z., and Yuan, Q. (2016). Xylose enhances furfural tolerance in Candida tropicalis by improving NADH recycle. Chem. Eng. Sci. 158, 37–40. doi: 10.1016/j.ces.2016.09.026
Wang, W., Ling, H., and Zhao, H. (2015). Steam explosion pretreatment of corn straw on xylose recovery and xylitol production using hydrolysate without detoxification. Process Biochem. 50, 1623–1628. doi: 10.1016/j.procbio.2015.06.001
Zhang, H. J., Fan, X. G., Qiu, X. L., Zhang, Q. X., Wang, W. Y., Li, S. X., et al. (2014). A novel cleaning process for industrial production of xylose in pilot scale from corncob by using screw-steam-explosive extruder. Bioprocess Biosyst. Eng. 37, 2425–2436. doi: 10.1007/s00449-014-1219-0
Zhao, P., Ge, S., and Yoshikawa, K. (2013). An orthogonal experimental study on solid fuel production from sewage sludge by employing steam explosion. Appl. Energy 112, 1213–1221. doi: 10.1016/j.apenergy.2013.02.026
Keywords: biorefining, industrial biotechnology, xylitol, steam explosion, Taguchi, xylose
Citation: Walker DJ, Gallagher J, Winters A, Somani A, Ravella SR and Bryant DN (2018) Process Optimization of Steam Explosion Parameters on Multiple Lignocellulosic Biomass Using Taguchi Method—A Critical Appraisal. Front. Energy Res. 6:46. doi: 10.3389/fenrg.2018.00046
Received: 20 December 2017; Accepted: 14 May 2018;
Published: 01 June 2018.
Edited by:
Jalel Labidi, University of the Basque Country (UPV/EHU), SpainReviewed by:
Vivekanand Vivekanand, Malaviya National Institute of Technology, Jaipur, IndiaFei Shen, Sichuan Agricultural University, China
Copyright © 2018 Walker, Gallagher, Winters, Somani, Ravella and Bryant. This is an open-access article distributed under the terms of the Creative Commons Attribution License (CC BY). The use, distribution or reproduction in other forums is permitted, provided the original author(s) and the copyright owner are credited and that the original publication in this journal is cited, in accordance with accepted academic practice. No use, distribution or reproduction is permitted which does not comply with these terms.
*Correspondence: Sreenivas R. Ravella, cnNyQGFiZXIuYWMudWs=