- 1UK Centre for Carbon Dioxide Utilisation, Chemical & Biological Engineering, Sir Robert Hadfield Building, The University of Sheffield, Sheffield, United Kingdom
- 2UK Energy Research Centre (UKERC), London, United Kingdom
We propose a hypothesis that fuels will continue to be critical elements of future energy systems. The reasons behind this are explored, such as the immense benefits conferred by fuels from their low cost of storage, transport, and handling, and especially in the management of the seasonal swing in heating demand for a country with a summer and winter season such as the UK. Empirical time-series data from Great Britain are used to examine the seasonal nature of the demand for liquid fuels, natural gas, and electricity, with the aid of a daily Shared Axis Energy Diagram. The logic of the continued need of fuels is examined, and the advantages and disadvantages of synthetic fuels are considered in comparison to fossil fuels.
Introduction
Nearly all modern energy systems are critically dependent on fossil fuels. Part of this is due to the cost and availability of fossil fuels versus other primary and secondary energy sources, but an often-overlooked reason is from the intrinsic benefit that these fuels bring. The ability to store terawatt hours (TWhs) of chemical energy economically allows the supply of primary energy to be decoupled from the demand of energy on a grand scale, both in terms of the timeframe and in terms of the location. For a country like Great Britain, with distinct summer and winter seasons, the ability to store TWhs of fuels for heating helps to balance out this major seasonal demand swing. The move toward ever-greater amounts of primary energy sourced from weather-dependent renewables (primary electricity1) brings challenges in the ongoing balance of supply and demand over different timescales, and over different distances, with the interseasonal swing in heat demand being one of the greatest.
The global deployment of weather-dependent renewable generation such as wind generation and solar PV seems set to continue, as costs decrease, and increased knowledge is gained on how to successfully integrate greater amounts of primary electricity within electrical systems (as a subset of wider energy systems). In 2014, 2015, and 2016 renewable generation (excluding large hydro) was 45.3, 51.3, and 55.3%, respectively, of the annual global electrical generation change in capacity (Frankfurt School-UNEP Centre, 2017), compared to a value of just 19.5% in 2007. This level of increase over a decade justifies that weather-dependent renewables can be considered a mainstream technology choice for many countries, which has helped to bring costs down, but like other mature forms of generation there are still several areas where technology development could bring additional cost improvements.
The major historical driver, at a time when the costs were higher for wind and solar PV generation, was for countries to encourage investment/deployment as a means to reduce their carbon emissions. This growth in markets led to manufacturing and technological advances that provided cost reductions, which itself helped greater investment and further growth. The original focus on carbon reduction is now being augmented by additional drivers such as the security of supply, diversification of primary energy sources away from fossil fuel imports, and increasingly the benefit of deploying renewable energy as an economic form of generation. In a similar vein to exploiting their fossil resources for the twin benefits of domestic economic activity and a decrease in imports, or even an increase in exports, the increase in experience and drop in generation prices allows countries to consider exploiting their renewable energy resources at greater scales than previously imagined. With countries singing up to the COP21 Paris Climate change agreement and submitting Intended Nationally Determined Contributions with lower-carbon targets, the decarbonization of the electrical system will continue to be a priority over the medium term: precisely because experience around the world shows that it is possible. In Great Britain, for example, a combination of demand reduction, increased low-carbon renewable generation, and fuel switching from coal to natural gas generation has provided an emissions reduction of 61% in 2016 from a 1990 baseline.
With the level of direct subsidies for renewable generation reducing, and capacity auctions growing increasingly common, the direct government costs of subsidizing renewable energy are becoming more controlled and understood; however, the indirect costs of accommodating higher and higher levels of primary electricity are still subject to considerable uncertainty. A major part of this is due to the increased costs of balancing electrical generation and demand in future systems that have less of a role for fuel-based generation. The ability to balance future energy systems (not just electrical energy systems) is hard to imagine without the benefit of fuels of some sort, and if fossil fuels are limited either for climate or other reasons, then there would seem to be a strong case for synthetic fuels to take their place.
The contribution of this article is to propose the critical ongoing need for fuel-based storage to overcome seasonal variations in energy demands for Great Britain, evidenced by using multi-year empirical time-series data for liquid fuels, natural gas, and electricity.
This paper is divided as follows: first, the levels of stored fuels in Great Britain are outlined, their levels discussed, and the initial case for fuels is put forward. Next, the seasonal variation in Great Britain’s energy demand for liquid fuels, natural gas, and electricity is presented with a top-level look at the synergy between solar and wind generation using empirical data. Next, the discussion and conclusion parts indicate the main findings of the paper and the consideration of these for a wider audience of policy makers and existing or potential bulk electrical storage operators.
Hypothesis
The hypothesis described in this article proposes that due to the sheer scale of fuel use that provides the interseasonal stores of energy that modern energy systems require, that the use of fuels will still be justified in future energy systems too. It is difficult to conceive of energy systems moving to a just-in-time provision of energy from non-fuel-based primary energy sources to final energy demand, as the challenges of balancing this over seasons without the benefit of fuels are insurmountable. Fuels confer immense energy system benefits due to their low cost of storage, transport, and handling and in any decarbonized future energy system, having low cost means of storing and transporting TWhs of energy will still be critical to the successful delivery of energy over an annual basis. The hypothesis is grounded in the belief that there will be a continued requirement to decouple primary energy supplies from demands at a grand scale on both a temporal scale and location. At an estimated 240 TWh of average stored energy in fossil fuels in Great Britain over the 2012–2016 period analyzed, the scale of this is compared to the seasonal nature of the demand for liquid fuels, natural gas, and electricity, with the aid of a daily Shared Axis Energy Diagram. The logic of the continued requirement of fuels is examined, and the advantages and disadvantages of synthetic fuels are considered in comparison to fossil fuels.
Average Amount of Stored Energy in Great Britain
Table 1 shows the approximate average amount of stored energy in Great Britain for the main solid (coal), gaseous (natural gas), and liquid fuels (crude oil and oil products) over the period from 2012 to 2016. There is a seasonal variation in the amount of coal and natural gas held in storage, but the total inventories of crude oil and oil products has less of a seasonal component. This is thought to be due to the underlying demand for the fuels themselves, with electricity (coal and natural gas) and heating (natural gas) having strong seasonal swings, which is less apparent with transport (crude oil and oil products); in addition, crude oil and oil products are traded internationally both import and export to a much greater degree than coal and natural gas.
At an approximate average level of 240 TWh, the levels of stored energy available in Great Britain’s fossil fuel stocks are vast. As a member of the IEA and the European Union (for the time period of the data), over half of this is in liquid fuels that is subject to some mandatory level of stored energy, the rest is not mandated and is therefore a market decision. These average levels of stored energy have provided a structurally adequate level of energy supply that has kept Great Britain away from the spectre of running out of energy. The energy supply shocks that have happened have been limited to other types of supply chain disruptions such as pay disputes by tanker drivers, rather than the stores of energy themselves being completely depleted (although March 2013 was a close call for natural gas).
As Great Britain moves to secure more and more of its primary energy needs from renewable electricity sources, there is a shift to weather dependency not only for demand but also for the supply of primary energy. This is said to bring new challenges to balance the difference between supply and demand over different timescales, which is undoubtedly true. However, energy systems have always required balancing over different timescales, so this is not a new challenge in and of itself, although the technologies and tools to do so are likely to be. Historically, fuels have allowed this decoupling of primary energy supply from demand on a grand scale at a temporal scale and location.
The question of whether this average stored level of energy at 240 TWh is optimized is explored in the next section.
Are the Levels of Stored Fuels Optimized?
All energy systems benefit from having stores of energy that act as buffers along their supply chain from the collection of primary energy to the final energy use. This is driven by a desire for a certain level of “energy security,” which itself is a challenging term to conceptualize (Kiriyama and Kajikawa, 2014; Cox, 2016). The question of how much stored energy should actually be available to an energy system such as Great Britain is an open research question, as it is not optimized at a whole system level between the electrical, natural gas, and liquid fuel networks. Even if it was, the level of stored energy of different fuels can change relatively quickly driven by the difference in demand created by switching fuels, e.g., the “dash for gas” building of natural gas generation in Great Britain (Winskel, 2002) had a major impact on the demands for coal and natural gas. In 2016, the price differential between coal and natural gas as a fuel for electrical generation, coupled with an effective carbon price, provided the market conditions for a fuel switch from coal to natural gas at an unprecedented rate (Wilson and Staffell, 2017).
The additional expense of having stores of energy is accepted and accommodated ultimately by the increase in price to end users, to provide a degree of resilience and reliability to reduce the impact of energy supply chain shocks. When supply shocks do happen to energy systems, economic activity is interrupted, with possible wider sociopolitical consequences too. For countries that have grown used to having high availability of energy systems, the lack of energy (or even the thought of the lack energy) for heating, transport, or provision of electricity looms as a major fear rather than a mere inconvenience. Depending on how long, how widespread, and how arduous an energy supply shock might be, there would be a widespread desire to hold someone to account, and from past experience in Great Britain, this is very likely to initially be the incumbent government of the day.
The amount of stored energy is influenced by market frameworks, such as the regulated market-based approaches used in Great Britain, and on the type of fuel and its cost of storage. Dependent on a complex interplay of factors, each market player will look to their own profitability for storing energy and may choose to physically store energy, e.g., coal or biomass somewhere along its supply chain, or to contractually purchase the delivery of fuel from a third party at a given timeframe, who therefore has the difficulty and expense of storing the fuel until it is required. Third party merchant facilities exist for the storage of fuels, for example, in Great Britain the natural gas grid has access to short-, medium-, and long-term (seasonal) storage facilities that all help smooth out the natural gas demand variation by having gas that is already “in-country,” and many of these are available to third parties to store natural gas.
If the individual companies’ self-interests align with the needs of the wider system, then this can provide system level benefits, but due to the costs of purchasing and storage of fuels, there are pressures to keep the stores of energy to acceptable levels, which are influenced by factors such as the anticipated change in price of the fuels. Thus, levels of stored fuels are influenced by the current and expected future costs of the fuel versus the cost of storing it, and companies may decide to have a physical hedge of fuel in the form of a stockpile to compliment or reduce the need for a financial hedge against increasing prices. There is a commercial interplay between these different costs and benefits, which is not well understood; however, the main point remains that the levels of stocks of fuels are unlikely to represent an optimized amount to provide a system level benefit.
There may be more opportunity of a system level benefit when there is a monopoly provider of the energy system to dictate the levels of storage, e.g., electricity in a state-owned monopoly, but again, the reason for the amounts of stored energy in fuels are not well understood. Even if there is logic to the level of stored energy on one of the energy systems in a country, such as the electrical system, it is highly unlikely that this will take account of the level of stored energy required in the natural gas or liquid fuels systems too. This lack of a whole systems appreciation of the amount of stored energy across all energy vectors in any country is endemic, but there are positive signs that this whole systems approach is gaining more interest at a policy level (Scottish Government Energy Consultation, 2017; http://www.gov.scot/Publications/2017/01/3414). As more primary energy is harvested using primary electricity, and parts of heating and transport demands are transferred over to the electrical system, this provides policy makers and regulators an opportunity to reconsider energy systems in a more holistic or whole systems manner; meaning that the different primary energy sources, energy vectors, and final energy demands are considered together.
Regardless of the ownership or operation of an energy system, energy stores can be mandated as a matter of political will, typically driven by the overall imperative to reduce the impact of supply chain shocks. A major example of these are the level of inventories of crude oil held by members of the International Energy Agency, equivalent to at least 90 days of their net oil imports. Joining the organization as a member places a legal requirement to hold a minimum inventory of crude oil, which will have a cost. Another example is the level of stocks of petroleum products under European Union legislation, which is based on the average daily consumption of the previous year (Bielecki, 2002). To the authors’ knowledge, there is no similar widespread legal obligation to hold a certain level of solid or gaseous fuels; liquid fuels have therefore attracted more political interest than solid or gaseous fuels, as the inventories of these are left up to the market, i.e., it is a market decision.
The Continued Need for Fuels
It is of little surprise that countries including Great Britain continue to be utterly dependent on fuels for their stored energy requirements; however, a question arises what it would use in the future if not fossil fuels? Large reductions in coal generation have already happened, falling below 10% of all electrical generation in 2016; the first time in Great Britain’s electrical history. Data suggest that even less electricity will be produced from coal in 2017, as the first quarter of 2017 shows coal generation to be 11% of the total, down from 16% of the total in the first quarter of 2016. This reduction, mainly due to an effective carbon price, is strengthened by a stated aim of the UK Government to remove unabated coal from Great Britain’s electrical system by 2025. If carbon capture and storage (CCS) does not provide coal generation with a lower-carbon future, then the stockpiles of coal, and the energy that they contained, will no longer be available to the system. This means the average of 80 TWh of energy contained in coal stocks between 2012 and 2016 will have gone; a large reduction has already been recorded for the first quarter of 2017 with an average level of below 40 TWh of stored chemical energy in coal (BEIS, 2017). As the first quarter is typically when stocks of coal are at their lowest, it remains to be seen how the levels will rise over the summer and autumn period in 2017.
Assuming that the stored energy of coal is therefore unavailable in Great Britain’s future energy systems (without CCS), the same might be argued for natural gas too, as this will come under increasing pressure to move off the system in a deeply decarbonized future. There is a large difference between the two fuels, with natural gas providing 80% of the heating demand of Great Britain as well as providing primary energy for the electrical system. Coal in comparison provides very little heating supply and is primarily used in the electrical and industrial sectors. Moving away from natural gas therefore brings the grand challenge of how to decarbonize the heating sector, as well as part of the electrical sector.
If coal and natural gas are no longer available to Great Britain, it is an open research question how it would balance its energy demand over the year. Due to costs or resource constraints, it is difficult to imagine how tens or hundreds of TWhs of energy can be stored in any energy form that is not a fuel. Non-fuel methods of storing energy are orders of magnitude more expensive than storing fuels, and although they will have vital roles to play in terms of balancing shorter and medium term supply and demand (within day, and days to weeks), they are ill suited to provide the TWh levels of seasonal balancing required by heating demand in particular.
The comparison of the average amount of stored energy of 240 TWh is compared to the daily energy demands of Great Britain in the next section.
Daily Shared Axis Energy Diagram for Great Britain
Figure 1 shows the different energy vectors that Great Britain used on a daily basis from 2014 to early 2017. The background and evolution of this daily Shared Axis Energy Diagram is described in Wilson (2016).
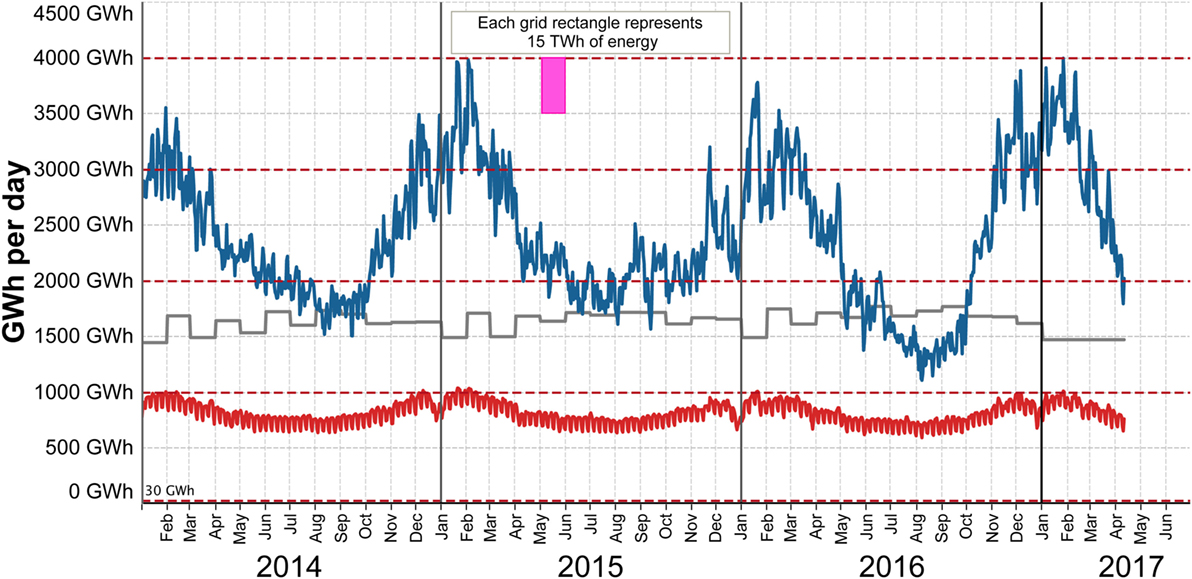
Figure 1. Daily Shared Axis Energy Diagram for Great Britain 2014–2016. Data sources, National Grid, Elexon, and BEIS. Data sources are described in Wilson et al. (2014).
The dark blue line is the natural gas demand in Great Britain less the amount exported through interconnectors to mainland Europe. The blocky gray line is the total of motor spirit (petrol), DERV (diesel), and aviation fuel. These are reported on a monthly basis by the department of Business, Energy and Industrial Strategy and are then calculated on a daily basis to be comparable with other data in the diagram; the flat end to this line over 2017 is an extension of the January values into February and March (as the reporting of liquid fuels has a time lag). The red line is the amount of electrical demand in Great Britain, which is calculated as the total of INDO (Initial National Demand Out-turn) and the amount of embedded wind and solar generation estimated by National Grid.
The red dotted line, close to the x-axis and marked as 30 GWh, is an approximation of the amount of energy that could be stored each day in Great Britain’s four pumped storage schemes in total (Wilson et al., 2010), and the magenta box is the size of one grid box in the figure and represents 15 TWh of energy.
Figure 2 replicates Figure 1 with the magenta box now showing the average amount of stored energy in Great Britain in fossil fuels. From Table 1, the value for coal is 80 TWh, natural gas is 30 TWh, and crude oil and oil products are 130 TWh, which total the 240 TWh shown.
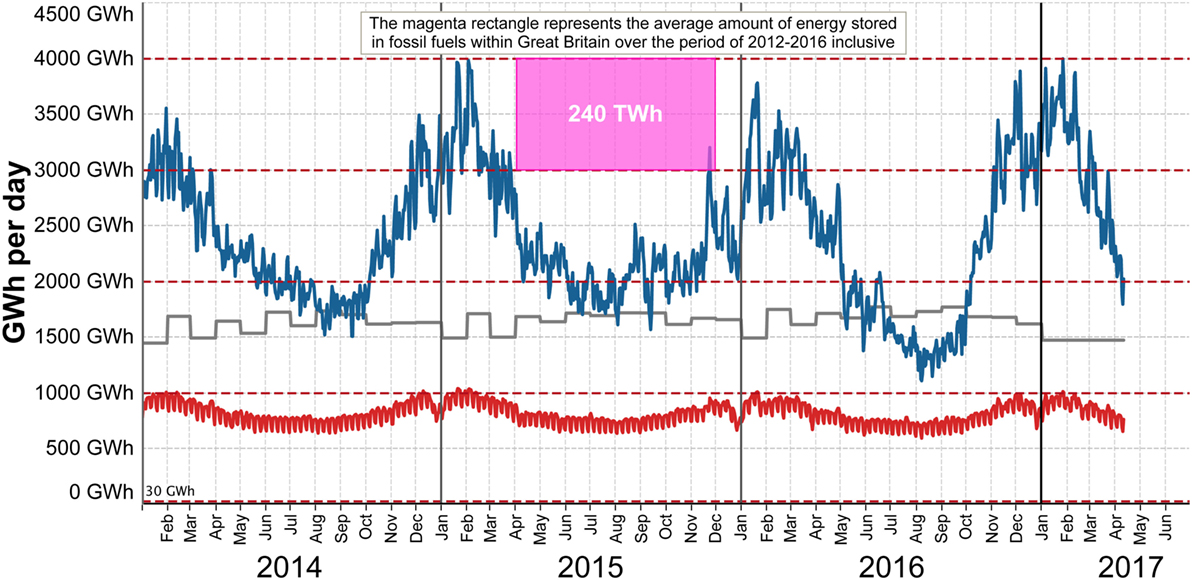
Figure 2. This figure shows the average amount of stored energy in fossil fuels within Great Britain over the period 2012–2016.
It is clear from both figures that the average amount of energy contained in fossil fuels over the 2012–2016 time period is large enough to provide seasonal levels of balancing, whereas the scale of pumped storage at 30 GWh is simply too small to operate over seasonal timeframes. Scaling this up to a global level by considering that in 2015 the primary energy demand of Great Britain was only ca. 1.5% of global primary energy demand (BP, 2017), the amount of stored energy in fossil fuels at a global level stored within countries is likely to be in the multi petawatt hour scale (as an order of magnitude estimate). If one were to consider the fossil fuels that are in situ in working reserves at the very start of their fossil fuel supply chains, then the stored energy at a global level could be into the exawatt hour range. However, as discussed by McGlade and Ekins (2015), many of these underground stores of energy are likely to come under increasing pressure not to be exploited and remain unused and underground.
The next section examines an amount of storage for the non-fuel sources of primary energy.
Daily Cumulative Difference Diagram for Renewable Electrical Generation
Figure 3 shows the cumulative difference of the storage required to firm up renewable generation of solar, wind, and solar + wind over the years from 2010 to 2016. The energy generated on each day is averaged over a year per technology, and this annual average is then subtracted from the daily generation value. If the daily output is below average then this gives a negative daily difference value, conversely if the daily output is above average then this will be a positive daily difference value. The annual charts in Figure 3 are the cumulative total of this daily difference, which starts and finishes the year at a zero amount. Conceptually, the maximum and minimum values for the running total are the amount of storage required to provide a flat average output over the year, given a 100% efficient electrical storage device. Although this top-level assessment is instructive, it is also unrealistic for a number of reasons: the calculation supposes an energy storage device with no efficiency losses, the calculation supposes an energy system that would want to have a flat output over a year from a renewable source. Nonetheless, the calculation shows the benefits of a combination of solar and wind that considerably reduces the need for an amount of storage for either generation type alone. The negative correlation of wind and solar therefore has a positive impact on the amount of storage required to provide a flat annual output and is indicative of the benefits of a diversified electrical generation portfolio.
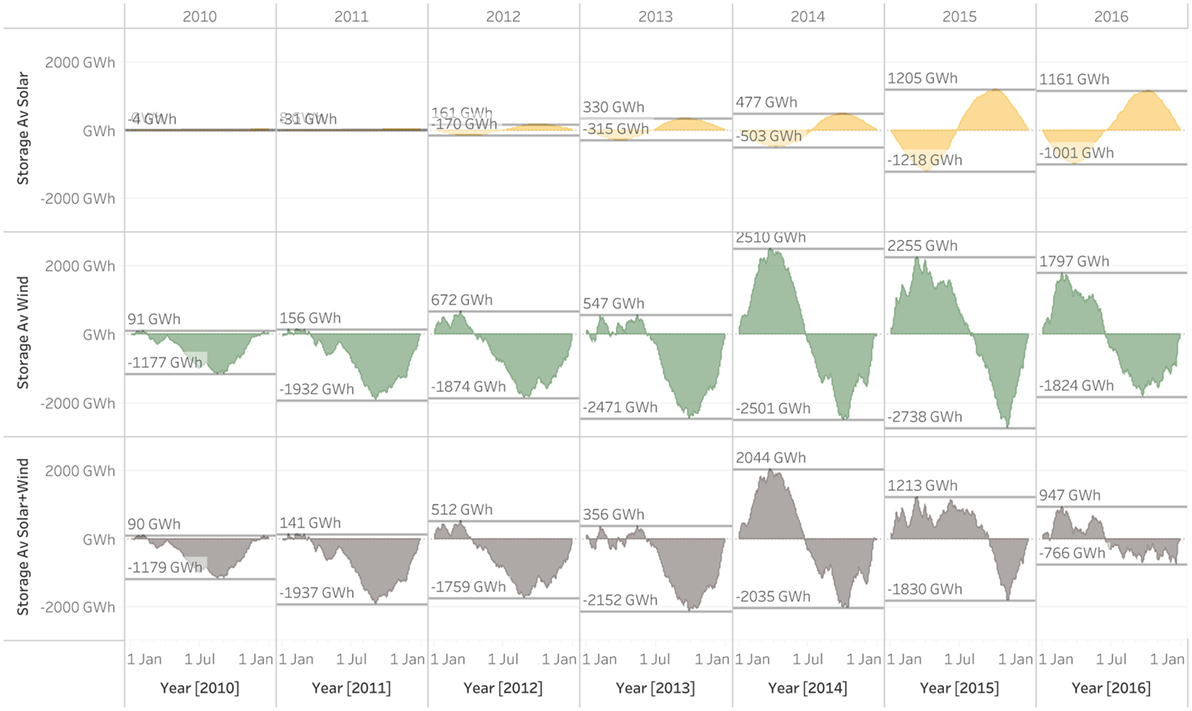
Figure 3. Cumulative difference between Great Britain daily and average annual output from solar, wind, and solar + wind.
This exercise also displays the differing range of outputs of weather-dependent renewable generation, with wind having many days considerably above average (high positive slopes in Figure 3), and concentrated in the winter and spring periods. As renewable generation capacity continues to be deployed, it brings with it an increased risk of times where curtailment is used to protect the electrical network itself, and the electrical equipment connected to it. The alternating current frequency of the network requires to be kept within strict limits, and curtailment of renewable energy is a method that is used by the system operator to manage the system by turning down or switching off the output from renewable generators. Historical balancing of electrical networks using thermal generation allowed the chemical energy in fuels to be kept in the fuel (by not combusting it) until it was required. This is not possible with wind generation, as the only ability to store the energy of the wind is after it is harvested as electricity. It is simply not feasible to store the wind as wind. The same is true of solar PV generation, as sunlight cannot feasibly be stored as sunlight, but there are technological avenues such as artificial photosynthesis (AP) that provide a route to stored energy without an electrical vector. The global solar PV capacity at the end of 2016 was 303 GW (IEA, 2017); however, this was on a steep trajectory being only 3.7 GW 10 years previously. Wind capacity at the end of 2016 was 486.8 GW (GWEC, 2016). Strictly speaking, AP does not use solar PV but chromophores within the AP system to directly harvest and use the photons. AP converts photons directly to products in a multistage process that mimics natural photosynthesis. This can be categorized into two main phases: hydrogen generation through water splitting (the light cycle) and the photocatalytic reduction of carbon dioxide using that hydrogen (the dark cycle, analogous to the Calvin cycle). If we decouple the two processes to say that the hydrogen is produced from any weather-dependent source (such as wind), then the term solar fuels is also used. Natural photosynthesis is highly inefficient, producing only enough of a particular product to maintain its survival, growth, and reproduction. This is typically around 1% solar efficiency for most plants. However, if we aim to produce fuels, this efficiency must increase significantly and the product must suit our energy needs. AP systems have been shown to operate an order of magnitude higher than a natural system. If wind power is used, then AP efficiency can be at least doubled due to the higher conversion efficiency of wind over solar PV. There are many studies and reviews of AP, and a recent European Commission study has highlighted the potential for further exploitation. While the water splitting reactions are well developed, effort is now focusing on the CO2 reduction step using catalytic and photocatalytic systems.
The deployment of AP, and solar fuels in general, will not only depend on the availability of the energy source but also on the availability of other resources necessary to the process. The message is that when designing any process, we must consider “location, location, location” (Styring, 2016). Solar PV and solar thermal energy is more suited to southern Europe, for example, while wind power is more suited to northern latitudes. It has also been suggested that due to the particularly high solar flux in the Sahara Desert, this would provide an ideal location. However, AP also needs water, a scarce commodity in such an environment; it also requires a source of carbon dioxide and again a desert is not an industrialized area. This would need water and CO2 to be piped in, at high cost, or in the case of CO2, direct air capture to be employed.
Use of Storage and Cost
If one wishes to store the output of weather-dependent electrical generation at scale to allow for seasonal levels of storage in the TWh range, then this is challenging for non-fuel systems of storage in terms of cost.
First, if one considers the target price from the US Department of Energy for batteries of $100 per kWh, then the cost for 1 TWh of batteries would be $100 billion. Second, the nature of seasonal storage is such that it might only be charged and discharged a handful of times a year. Due to the upfront cost of the batteries, there would be an understandable desire to use them more often than the handful of times a year, to reduce the per unit storage cost of a unit of electrical energy. As a broad example, $100 per kWh, 100% efficiency, and an estimated lifespan of 5,000 charge/discharge cycles would provide a per kWh unit of energy storage cost of 100/5,000 = 2 cents per kWh, in essence, the more batteries or for that matter any other storage device that has a high capital cost are utilized, the more favorable it is for the eventual unit price of stored energy.
Different forms of energy storage are better suited to storing energy over different timeframes. Electrochemical forms of storage are rapidly developing in terms of cost, and these are highly suited to storing electrical energy over a cycle that may last anywhere from minutes to hours to days up to weeks. However, as the previous paragraph makes clear—they are ill suited to cycles that last from weeks to months to seasons, precisely because the capital cost makes it desirable to utilize them often to reduce the unit cost of energy that is stored.
This desire to increase the charge/discharge cycles of a storage technology is incompatible with the characteristics required for seasonal levels of storage, which may need the energy to be held over months until it is required. This challenge is however ideally suited to fuels, as these are orders of magnitude cheaper to store on a unit price of stored energy. This is the reason that they have historically been used as seasonal stores of energy and is one of the main foundations of the hypothesis that fuels will continue to have a critical role to play in future energy systems.
If this basis of the hypothesis is accepted, then there is still the question of which types of fuels might be suited to this in the long term.
Synthetic Fuels Versus Fossil Fuels
The overriding benefit of fossil fuels versus synthetic fuels is due to their cost advantage on an energy basis. Fossil fuels are the most traded physical commodities in the world, leading to a highly competitive environment. Technology advancements in the oil, natural gas, and coal sectors have allowed new deposits to be exploited, e.g., tight oil/gas and shale oil/gas, and the competitive nature of these hydrocarbon markets brings constant pressures to supply chains to limit cost increases or drive further cost savings.
After many decades and trillions of US dollars of investment in supply chain improvements and generation of knowledge, it is nearly impossible for synthetic fuels to compete economically with fossil fuels purely on a cost per unit of energy basis (Pérez-Fortes et al., 2016). It can be argued that with significant levels of investment to encourage synthetic fuel supply chains to develop and innovate, that synthetic fuels can seek to close this cost gap to fossil fuels. However, the perennial cost challenge is not only due to the maturity and scale of supply chains but also and maybe more importantly due to where the actual energy stored in the fuels comes from in the first place.
The main environmental problem with fossil fuels is with their unabated combustion that releases fossil carbon back into the atmosphere, which will become increasingly expensive in a carbon-constrained world. Fossil fuels by statistical convention are classed as primary energy, when in fact, they could be classed as secondary energy carriers, as the original energy is due to sunlight that created the original biogenic material that subsequently became a fossil fuel. The overall conversion for this is extremely low, from the photon to eventual energy contained in the fossil fuel, but this is of little concern, as the reserves of fossil energy reserve are available in a concentrated form that can be harvested with the expenditure of some energy. For most fossil fuels the energy returned on energy invested (EROI) is high, as one only needs to expend a small part of the energy contained in the fossil fuel to actually harvest the fossil fuel itself. In a comprehensive meta-analysis on EROI for various fuels and energy harvesting (Hall et al., 2014), coal is reported to have an EROI of 46:1, oil and gas at 20:1, nuclear at 13:1, hydroelectric power generation at 84:1, wind power 18:1, and solar power 10:1. These reported values are subject to a range of uncertainties but give an indication of the relative EROI of different energy sources. Interestingly, the EROI of fossil fuels and nuclear, the mainstay of most electrical systems, is reducing over time as the easier won deposits (the high EROI reserves of these fuels and mineral ores) continue to be depleted, leaving lower EROI reserves to take their place. This contrasts with the technology and supply chain improvements in renewables such as wind and solar generation, where the EROI for these technologies has steadily improved, albeit from a low starting point.
The EROI from the harvesting of fossil fuels is the fundamental basis of the energy leverage that has driven economic and population growth since the industrial revolution. All societies at any level of advancement are utterly dependent on their access to energy, without it, their societies collapse (Hall et al., 2009). So, this period of human history leading to unprecedented increases in population is directly linked to this return on energy invested (McKevitt and Ryan, 2014).
Synthetic fuels, on the other hand, have much lower EROIs if they are derived from biomass, and in the case of power-to-fuels have EROIs that are less than one. All routes to creating synthetic fuels have process efficiency losses, which means that the amount of energy put into synthetic fuels to create them is always going to be less than the energy eventually stored in the fuels. This, above all else, means that power-to-fuels have a cost disadvantage compared to harvested fuels such as fossil fuels with higher EROIs. In short, power-to-fuels will always have an EROI below 1, whereas fossil fuels will continue to have EROIs well above 1. Having an EROI below 1 combined with a renewable energy source provides a combined generation and storage EROI above 1, which in the long-run is the basis of a sustainable energy system.
There are a wide range of fuels that can be synthesized using power (electricity) to provide the energy to be stored in the fuels. The creation of hydrogen from electrolytic splitting of water is a typical starting point for power-to-fuels and can itself be an energy vector. The challenge with hydrogen however lies with its storage, with hydrocarbon fuels proving much easier to store due to their physical characteristics. The concept of transporting hydrogen through natural gas infrastructure has undergone a renaissance in Great Britain in 2016, as evidenced by the interest at a policy and industry level generated around the Leeds Citygate H21 project (Sadler, 2016). Prior to a changeover to using natural gas from the North Sea, the gas networks of Great Britain used to supply town gas (also known as synthesis gas) as a mixture of hydrogen and carbon monoxide produced from the gasification of coal. The Citygate H21 project considers whether a repurposing of the natural gas network to transport 100% hydrogen would be feasible, and the challenges involved in undertaking this transition. One of the major challenges of the identified is in the manufacture of approximately 6 TWhs of hydrogen on an annual basis. This is felt to require the mature process of producing hydrogen at scale, the steam reformation of natural gas to strip the hydrogen from natural gas to leave CO2. If this CO2 from the steam methane reforming process were not sequestered, there would be a carbon increase for the final energy delivered, so the concept is critically dependent on having a CCS infrastructure available. However, there is room for the growth of hydrogen from electrolysis, but the sheer volumes of energy needed and existing costs of electrolysis are thought to preclude this pathway to provide significant levels of hydrogen in the short term. The project is interesting as it contains many of the considerations for the continued use of fuels, be they natural gas itself, or hydrogen derived from this natural gas (with CCS) or from hydrogen created by electrolysis using low-carbon electricity.
The owners and operators of natural gas infrastructure clearly have a vested interest in the continued use of their assets but are grappling with the challenges of meeting deep decarbonization targets with the continued use of unabated natural gas.
Over the long term, the use of synthetic fuels versus the creation of low-carbon fuels from fossil fuels using CCS will be determined by price, which will itself be determined by policy. One area where synthetic fuels have an advantage is being able to help balance the electrical grid, which as mentioned earlier is likely to suffer from the ever-greater risks of the excess supply of electrical energy. This is a complex area to understand in detail due to the interaction between future demands and the portfolio of future electrical generation, but the principle is that power-to-gas can provide flexibility to the electrical system as a highly controllable demand, and in addition provide a source of storable fuels for the electrical, gaseous, or even liquid fuels systems. This is one of the reasons that power-to-gas is being actively investigated to gain a better understanding of the potential of the sector to be a key element in the flexibility of future energy systems.
CO2 Fuels
The existing infrastructure to handle, transfer, and store fuels is primarily built for hydrocarbon fuels, which have carbon atoms bonded to hydrogen. Given this, there is a deep experience and knowledge base of the costs and the risks of these fuels in markets and in the regulatory and safety frameworks that surround them. Building this knowledge using fuels other than hydrocarbons takes time, and the costs of developing infrastructure for non-hydrocarbon fuels is likely to be costly, with valid questions arising around path dependency for future energy systems. As one of the main problems with fossil fuels is due to their unabated combustion and therefore the release of carbon dioxide into the atmosphere, which increases greenhouse gas concentration, several potential solutions have been proposed and developed. The main solution is CCS, where the carbon dioxide is captured by one of several techniques, and then stored back underground in a suitable geological repository. Depending on the technique used, this can capture upwards of 90% of the carbon that otherwise would have been released to the atmosphere without CCS. Due to the economy of scale benefits of CCS, these are suited to larger scale point source emitters of CO2 such as industrial and power sector plants.
A related concept that is garnering greater interest is the technique of using captured carbon to produce synthetic hydrocarbons or other forms of CO2 fuels that are easier to store than hydrogen. This is not a replacement for CCS, as the fuels created via this route will likely be combusted and the CO2 released to the atmosphere. Synthetic methane and methanol are of particular interest, with demonstration projects in power-to-methane using hydrogen produced by electrolysis and carbon from CO2 to create methane that is injected into existing natural gas infrastructure. There are disadvantages to this approach in terms of additional efficiency losses introduced by the extra step of adding carbon to hydrogen, as opposed to the creation of hydrogen itself. However, the concept is of interest as it allows the continued use of the natural gas infrastructure, with all the benefits in terms of handling, transport, and storage.
Even though there may be clear benefits for the use of synthetic fuels in terms of an extended lifetime of existing hydrocarbon fuel infrastructure, and potentially a security of supply benefit of creating fuels within a country’s national borders, the climate benefits of synthetic fuels are challenging to analyze. A full life cycle analysis needs to be undertaken for each synthetic fuel, which takes account of the source and therefore the carbon footprint of the energy inputs; which is complex, as the carbon footprint of grid electricity is constantly changing due to the underlying mix of generation plant at any moment in time. The other important consideration is where the carbon itself came from, and how this is accounted for. There should instinctively be a difference if the carbon came from a biogenic source and therefore was already above ground when it was captured by the biomass (thus reducing atmospheric CO2), as opposed to carbon that was captured from a fossil fuel-based power plant, where the carbon has come from underground. If the fuel is eventually combusted without the carbon being captured again, then the carbon from the biomass can be argued to be rereleased back to the atmosphere without the addition of a further carbon atom. In comparison, if the carbon atom was originally from a fossil fuel source, if the synthetic hydrocarbon is eventually combusted without the carbon being captured again, then this will provide an additional carbon atom into the atmosphere. There is an ongoing debate around the climate benefits of biomass itself (Brack, 2017), and also when a carbon atom in the atmosphere stops being fossil based, and is merely considered just another carbon atom in the mixture of atmospheric CO2. In short, there are likely to be a range of climate benefits to synthetic fuels (especially, if one considers the potential reduction in the use of fossil fuels), but these are particularly sensitive to a range of input conditions.
Conclusion
The hypothesis of this article proposes that the benefits to energy systems from the stores of fossil fuel-based energy will not be able to be replaced without some other form of fuel-based energy storage; the question should therefore be what type of fuel-based storage, not whether it is required. All energy systems (not just electrical energy systems) have benefited from the TWhs of stored chemical energy intrinsic in fossil fuels that has allowed a decoupling of primary energy supplies from the final use demand on a grand scale. The hypothesis is that this will continue to be the case.
As an example, Great Britain’s primary energy demand was explored using daily data, and as a northern European country with a range of inefficient building stock, its primary energy demand can be seen to be highly seasonal due to the demand for heating through the winter period; this is shown in Figure 1. With effective policies and investment to increase the efficiency of building envelopes, the seasonal variation of the heat demand for primary energy supplies should reduce between the summer and the winter, but even so, there will still be a significant difference between the primary energy required on a daily basis in the winter versus the summer. In the past, fuels have provided the TWh buffers of energy required to provide seasonal levels of stored energy, and it is highly likely that in the future Great Britain will continue to require TWhs of storage to provide security of supply buffers of energy to provide system resilience. Widening this, it seems clear that all energy systems of any country will still require some part of their TWh levels of stored energy into the long-term, what is less clear is the form that these might take.
This scale and use of seasonal levels of storage at a national scale that are well into the TWh region points to the use of fuels rather than other forms of storage, due to the unit cost of storing energy that is only stored and utilized a handful of times a year.
Given the cost disadvantages of synthetic fuels, if policy makers wish to encourage them to grow in market share, then they could consider providing protected markets for them to compete within, provide subsidies, or by costing fossil fuels with a carbon price that helps to close the cost gap.
The creation of synthetic fuels also provides a highly dispatchable demand to help integrate greater levels of weather-dependent renewables, which will be increasingly desirable in future energy systems that rely on primary electricity from renewables to a much greater degree.
The question simply put is not whether we will continue to need fuels in future energy systems, but the type of fuels that will be suitable in a highly decarbonized world.
Author Contributions
Dr. Grant Wilson is the main author for the article. Professor Styring provided comments and input to several sections. Both authors approve it for publication.
Conflict of Interest Statement
The authors declare that the research was conducted in the absence of any commercial or financial relationships that could be construed as a potential conflict of interest.
Acknowledgments
The authors wish to thank Dr. Keith MacLean and the two reviewers for discussion and feedback on the paper.
Funding
This work was part funded by the United Kingdom’s Engineering and Physical Sciences Research Council’s UK Energy Research Centre Phase 3 (grant EP/L024756/1) and CO2Chem Grand Challenge Network (EP/K007947/1).
Footnote
- ^Electricity that is harvested directly from nature without the need for a fuel, inter alia solarPV, solarThermal, wind, wave geothermal, and tidal.
References
BEIS. (2017). Coal Consumption and Coal Stocks (ET 2.6). Available at: https://www.gov.uk/government/uploads/system/uploads/attachment_data/file/593387/ET_2.6.xls
Bielecki, J. (2002). Energy security: is the wolf at the door? Q. Rev. Econ. Finance 42, 235–250. doi:10.1016/S1062-9769(02)00137-0
BP. (2017). Energy Charting Tool. Available at: http://tools.bp.com/energy-charting-tool.aspx#/st/primary_energy/dt/consumption/unit/MTOE/country/GB/view/map/
Brack, D. (2017). Woody Biomass for Power and Heat: Impacts on the Global Climate. Available at: https://www.chathamhouse.org/publication/woody-biomass-power-and-heat-impacts-global-climate
Cox, E. (2016). Opening the black box of energy security: a study of conceptions of electricity security in the United Kingdom. Energy Res. Soc. Sci. 21, 1–11. doi:10.1016/j.erss.2016.06.020
Frankfurt School-UNEP Centre. (2017). Global Trends in Renewable Energy Investment 2017. Available at: http://fs-unep-centre.org/sites/default/files/publications/globaltrendsinrenewableenergyinvestment2017.pdf
GWEC. (2016). Global Wind Report 2016. Available at: http://www.gwec.net/publications/global-wind-report-2/global-wind-report-2016/
Hall, C., Balogh, S., and Murphy, D. (2009). What is the minimum EROI that a sustainable society must have? Energies 2, 25–47. doi:10.3390/en20100025
Hall, C. A. S., Lambert, J. G., and Balogh, S. B. (2014). EROI of different fuels and the implications for society. Energy Policy 64, 141–152. doi:10.1016/j.enpol.2013.05.049
IEA. (2017). PVPS A Snapshot of Global PV. Available at: http://iea-pvps.org/fileadmin/dam/public/report/statistics/IEA-PVPS_-_A_Snapshot_of_Global_PV_-_1992-2016__1_.pdf
Kiriyama, E., and Kajikawa, Y. (2014). A multilayered analysis of energy security research and the energy supply process. Appl. Energy 123, 415–423. doi:10.1016/j.apenergy.2014.01.026
McGlade, C., and Ekins, P. (2015). The geographical distribution of fossil fuels unused when limiting global warming to 2°C. Nature 517, 187–190. doi:10.1038/nature14016
Pérez-Fortes, M., Schöneberger, J. C., Boulamanti, A., and Tzimas, E. (2016). Methanol synthesis using captured CO2 as raw material: techno-economic and environmental assessment. Appl. Energy 161, 718–732. doi:10.1016/j.apenergy.2015.07.067
Sadler, D. (2016). H21 Leeds City Gate. Available at: http://www.northerngasnetworks.co.uk/archives/document/h21-leeds-city-gate
Styring, P. (2016). “CO2 utilisation fuels,” in 5th Conference on Carbon Dioxide as Feedstock for Fuels (Köln, Germany: Nova Institute).
Wilson, I. A. G. (2016). Energy data visualization requires additional approaches to continue to be relevant in a world with greater low-carbon generation. Front. Energy Res. 4:33. doi:10.3389/fenrg.2016.00033
Wilson, I. A. G., McGregor, P. G., and Hall, P. J. (2010). Energy storage in the UK electrical network: estimation of the scale and review of technology options. Energy Policy 38, 4099–4106. doi:10.1016/j.enpol.2010.03.036
Wilson, I. A. G., Rennie, A. J. R., and Hall, P. J. (2014). Great Britain’s energy vectors and transmission level energy storage. Energy Procedia 62, 619–628. doi:10.1016/j.egypro.2014.12.425
Wilson, I. A. G., and Staffell, I. (2017). The Year Coal Collapsed: 2016 was a Turning Point for Britain’s Electricity. Available at: https://theconversation.com/the-year-coal-collapsed-2016-was-a-turning-point-for-britains-electricity-70877
Keywords: synthetic fuels, CO2 fuels, seasonal storage, low-carbon fuels, TWh storage
Citation: Wilson IAG and Styring P (2017) Why Synthetic Fuels Are Necessary in Future Energy Systems. Front. Energy Res. 5:19. doi: 10.3389/fenrg.2017.00019
Received: 12 May 2017; Accepted: 04 July 2017;
Published: 24 July 2017
Edited by:
Youngjune Jason Park, Gwangju Institute of Science and Technology (GIST), South KoreaReviewed by:
Sune Dalgaard Ebbesen, Innovation Fund Denmark, DenmarkDong-Yeun Koh, Georgia Institute of Technology, United States
Copyright: © 2017 Wilson and Styring. This is an open-access article distributed under the terms of the Creative Commons Attribution License (CC BY). The use, distribution or reproduction in other forums is permitted, provided the original author(s) or licensor are credited and that the original publication in this journal is cited, in accordance with accepted academic practice. No use, distribution or reproduction is permitted which does not comply with these terms.
*Correspondence: I. A. Grant Wilson, Z3JhbnQud2lsc29uJiN4MDAwNDA7c2hlZmZpZWxkLmFjLnVr