- 1Climate and Sustainable Cities, IVL Swedish Environmental Research Institute, Stockholm, Sweden
- 2Division of Physical Resource Theory, Department of Energy and Environment, Chalmers University of Technology, Göteborg, Sweden
- 3Division of Energy Technology, Department of Energy and Environment, Chalmers University of Technology, Göteborg, Sweden
This paper maps, categorizes, and quantifies all major point sources of carbon dioxide (CO2) emissions from industrial and combustion processes in Sweden. The paper also estimates the Swedish technical potential for electrofuels (power-to-gas/fuels) based on carbon capture and utilization. With our bottom-up approach using European databases, we find that Sweden emits approximately 50 million metric tons of CO2 per year from different types of point sources, with 65% (or about 32 million tons) from biogenic sources. The major sources are the pulp and paper industry (46%), heat and power production (23%), and waste treatment and incineration (8%). Most of the CO2 is emitted at low concentrations (<15%) from sources in the southern part of Sweden where power demand generally exceeds in-region supply. The potentially recoverable emissions from all the included point sources amount to 45 million tons. If all the recoverable CO2 were used to produce electrofuels, the yield would correspond to 2–3 times the current Swedish demand for transportation fuels. The electricity required would correspond to about 3 times the current Swedish electricity supply. The current relatively few emission sources with high concentrations of CO2 (>90%, biofuel operations) would yield electrofuels corresponding to approximately 2% of the current demand for transportation fuels (corresponding to 1.5–2 TWh/year). In a 2030 scenario with large-scale biofuels operations based on lignocellulosic feedstocks, the potential for electrofuels production from high-concentration sources increases to 8–11 TWh/year. Finally, renewable electricity and production costs, rather than CO2 supply, limit the potential for production of electrofuels in Sweden.
Highlights
• Sweden emits 50 million metric tons of CO2 per year from different types of point sources, the vast majority of which is emitted at low concentrations.
• Of this, 65% is from biogenic sources, most of which are located in southern Sweden.
• Currently, the high-concentration sources of CO2 in Sweden can provide a potential 1.5–2 TWh electrofuels/year (2% of current transportation demand).
• The Swedish potential for electrofuels is currently limited by the electricity required and production costs rather than the amount of recoverable CO2.
Introduction
Anthropogenic greenhouse gas (GHG) emissions need to be reduced in order to limit global climate change and reach ambitious climate targets (Pachauri et al., 2014). Carbon dioxide (CO2) emissions can be reduced by using less fossil fuels or by using fossil fuels in combination with carbon capture and storage (CCS) or carbon capture and utilization (CCU) [e.g., Cuéllar-Franca and Azapagic (2015), Wismans et al. (2016)]. In Sweden, the overall national vision is for zero net emissions of GHG to the atmosphere by 2050 (likely to be changed to 2045), along with a fossil fuel-independent vehicle fleet by 2030 (Government offices of Sweden, 2009; Swedish Government Official Reports, 2016). An extensive official investigation commissioned by the Swedish government has concluded that a range of options are needed to reduce CO2 emissions from the transport sector, including biomass-based liquid and gaseous fuels (biofuels) along with hydrogen and electricity produced from renewable energy sources (Swedish Government Official Reports, 2013).
However, neither government nor academia have explored electrofuels (i.e., power-to-gas/fuels or synthetic hydrocarbons produced from CO2 and water using electricity), extensively. Interest in electrofuels is on the rise, both in the literature (Graves et al., 2011; Mohseni, 2012; Nikoleris and Nilsson, 2013; Taljegård et al., 2015)1 and in terms of demonstration plants in the EU, in some cases, including CO2 capture (Gahleitner, 2013). Studies mainly investigate electrofuels as a (i) technology for storing intermittent electricity [e.g., Streibel et al. (2013), de Boer et al. (2014), Vandewalle et al. (2014), König et al. (2015), Qadrdan et al. (2015), Varone and Ferrari (2015), Zakeri and Syri (2015), Zhang et al. (2015), and Kötter et al. (2016)], (ii) fuel for transport [e.g., Connolly et al. (2014), Ridjan et al. (2014), Larsson et al. (2015)], or (iii) means of producing chemicals [e.g., Ganesh (2013), Perathoner and Centi (2014), and Chen et al. (2016)]. Different types of energy carriers [e.g., methane, methanol, DME (dimethyl ether), gasoline, and diesel] can be produced, which makes electrofuels a potentially interesting option for all transport modes, especially shipping, aviation, and long distance road transport, where the potential for other renewable fuel options, such as electricity and hydrogen, may be limited. Electrofuels may allow increased use of biofuels, if the CO2 associated with their production is used for production of electrofuels instead of being emitted to the atmosphere (Mignard and Pritchard, 2008; Mohseni, 2012; Hannula, 2015, 2016).
CO2 emissions can be captured from various point sources, including industrial processes that produce CO2, such as biofuel production (including anaerobic digestion and fermentation), natural gas processing, steel plants, and oil refineries, fossil and biomass combustion in heat and power plants, or directly from the air.
Many studies have estimated CO2 emissions from point sources in China [e.g., Chen and Chen (2010), Liu et al. (2010), Zhang and Chen (2014)]. Zhang and Chen (2014) used a bottom-up approach to estimate CO2 emissions from fuel combustion and the main industrial processes at 7.7 Gt CO2 per year in 2008, with coal as the main source. The potential global supply of CO2 from point sources is estimated in Naims (2016). The total estimated global capturable CO2 supply from point sources amount to approximately 12.7 Gton of CO2 (Naims, 2016). High purity point sources (e.g., fermentation of biomass and ammonia production) and other low cost sources (e.g., bioenergy, natural gas, and hydrogen production) represent in total approximately 0.3 Gton of CO2. Naims (2016) further indicates that there is enough CO2 to meet the estimated global CO2 demand in the near and long term.
In Austria, the iron and steel, cement industry, and power and heat industries are the largest point sources of CO2 emissions (Reiter and Lindorfer, 2015). Biofuel production, a relatively modest point source at about 113 kton in 2013, is considered the most suitable Austrian source for power-to-gas application by Reiter and Lindorfer (2015). A German feasibility study by Trost et al. (2012) identifies a large potential for biogenic CO2 sources, including biogas upgrading, bioethanol plants, and sewage treatment plants. Trost et al. (2012) also found a substantial electrofuels potential of over 130 TWh fuel per year in the form of methane produced using CO2 from industrial processes and biogenic sources. Reiter and Lindorfer (2015) and Trost et al. (2012), both conclude that availability of CO2 will not be a limiting factor for using power-to-gas as a balancing strategy for intermittent renewable power sources (wind power and photovoltaics) in Austria or Germany.
In Sweden, carbon capture is currently implemented at, for instance, Agroetanol in Norrköping. Agroetanol produces grain-based ethanol; the resulting CO2 is purified and sold to the AGA Gas AB. Detailed quantification of current and/or future Swedish CO2 emissions from point sources is, however, lacking in the scientific literature, and there are no assessments of the technical potential for Swedish production of electrofuels. Electrofuels may represent an interesting option in Sweden, that is a forest-rich country, due to the ambitious GHG emission reduction targets in general and specifically in the transport sector. Assessing the Swedish potential for CCS and CCU requires detailed knowledge of the stationary CO2 emissions. The overall impact on CO2 emissions of the production and use of electrofuels mainly depends on the electricity-related CO2 emissions. The Swedish electricity production consists mainly of hydro power and nuclear power implying relatively low GHG emissions.
The overall aim of this paper is to map and quantify stationary Swedish CO2 emissions by concentration, origin, and geographical distribution, as well as investigate the potential for CCU. Specifically, we aim to (i) map and quantify the major point sources of CO2 emissions from industrial and combustion processes in Sweden with a bottom-up approach and estimate the technical potential for CO2 capture or recovery and (ii) estimate the technical potential for production of electrofuels in Sweden, as an example of CCU. We analyze the potential for biofuels-related CO2 in the future (a 2030 scenario), since the use of biomass and biofuels is expected to increase and use of fossil fuels decrease. Additionally, we estimate the potential demand for CO2 and electricity corresponding to the use of electrofuels for road transport, heavy trucks, and shipping, at scale, in order to give a first indication of the potential role for electrofuels in transportation in Sweden.
Materials and Methods
This section describes the methodology for estimating both CO2 emissions from major point sources and the potential for capturing and using the emissions.
Assumptions about the CO2 Sources Included
CO2 emission sources can be divided into diffuse sources (e.g., transport and agriculture) and point sources (e.g., factories and power production). This study uses a bottom-up approach to estimate CO2 emissions from the following point sources in Sweden:
• Industrial process plants (including iron and steel, non-ferrous metal, oil and gas refineries, lime and cement, pulp and paper, chemical, metal, and other similar plants)
• Heat and power production (including biomass, waste, and fossil fuel-fired plants)
• Biofuels production facilities (including ethanol, biogas, and more advanced biofuels).
Emissions data for year 2013 from the European Environment Agency’s “European Pollutant Release and Transfer Register” (European Environment Agency, 2015) was used to estimate (i) the available amount of CO2 and (ii) the share of fossil and biogenic CO2, for Swedish point sources, including all sources emitting 0.1 million metric tons of CO2 per year or more. Other CO2 sources are assumed to be negligible (except in the case of biofuels production). The concentration of CO2 for each type of sources was estimated using (Chapel et al., 1999; Bosoaga et al., 2009) (see Table 1). For the purposes of analysis, the concentrations were divided in three ranges: low (<15 vol%), medium (15–90 vol%), and high (>90 vol%).
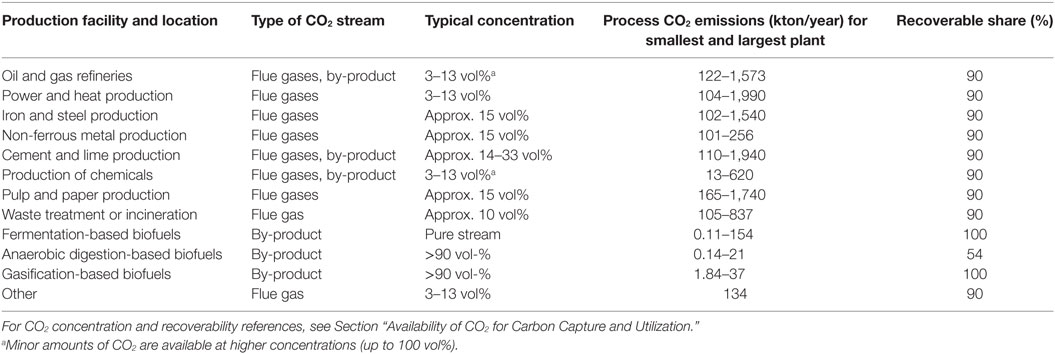
Table 1. The type of CO2 stream, CO2-concentration range, range of CO2 emissions per unit, and share of recoverable CO2, for different point sources in Sweden based on European Environment Agency (2015).
For biofuels plants, the CO2 estimates are based on data gathered by Swedish Energy Agency and Energigas Sverige (2015) and Grahn and Hansson (2015) in 2012–2013. Also, the sources emitting less than 0.1 million metric tons of CO2 per year are included in the case of biofuels since these are relatively pure and, therefore, well suited for electrofuels production. In most biofuels production processes, there is a surplus of CO2 and the CO2 is of high purity (Xu et al., 2010). When biogas is upgraded to transport fuel quality, a cleaning step to remove CO2 is included, resulting in a relatively pure stream of CO2. The CO2 emissions from domestic biofuel production in a 2030 scenario are estimated based on biofuels production scenarios from Grahn and Hansson (2015) and on scenarios for anaerobic digestion and gasification-based biogas production from Dahlgren et al. (2013). Grahn and Hansson (2015) assessed the potential contribution of domestically produced biofuels for transport in Sweden in 2030 based on a mapping of the prospects for current and potential Swedish biofuel producers. Some of the planned biofuels production plants included in the scenario for 2030 have been canceled or put on hold and are, therefore, excluded in this study.
The 2030 scenario was constructed exclusively for biofuel plants because these represent a relatively pure stream of CO2 of particular interest in electrofuels production, and because the use of biofuels is expected to increase in the future. For many biofuels, no extra major purification step is needed in the capture process, which leads to a relatively low capture cost. This can also be assumed for the case of biogas since CO2 is already removed when biogas is upgraded to transport fuel quality. This can be compared to the CO2 capture cost linked to processes requiring an extra purification step like steel and iron, ammonia, refinery, cement, and fossil or biomass combustion plants estimated at 20€2015–170€2015/ton CO2 in the short term (10–15 years) and 10€2015–100€2015/ton CO2 in the more long term (Damen et al., 2007; Finkenrath, 2011; Kuramochi et al., 2012, 2013; IEA, 2013). Even though it has been indicated that the cost for carbon capture represents a relatively modest share (a few percent) of the total electrofuel-production cost unless air capture is assumed (Graves et al., 2011; Tremel et al., 2015; Varone and Ferrari, 2015; see text footnote 1), using CO2 from biofuel production represent an attractive source for electrofuel production since more pure streams will likely be used first for economic reasons and the domestic biofuel actors, representing a considerable biofuel production capacity, in order to comply with sustainability requirements need to improve their production processes in terms of CO2 emissions.
Table 1 presents the type of CO2 stream, typical concentration of CO2, the range of CO2 emissions per unit, and the amount of recoverable CO2, for different point sources. Table 2 includes a list of all the biofuel production facilities in operation in 2015, their production capacity and associated CO2 emissions, and the corresponding information for the biofuels plants planned by 2030. Table 3 summarizes the main assumptions used in estimating the amount of CO2 that is available for recovery from current and future biofuels plants.
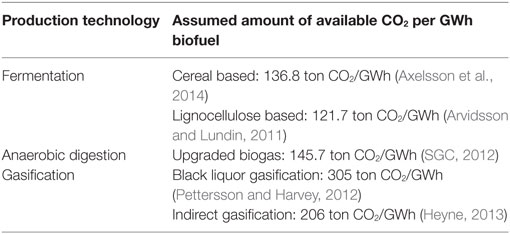
Table 3. Main assumptions for assessing CO2 availability from current and future biofuels plants in Sweden.
Availability of CO2 for CCU
In order for CO2 to be used to produce electrofuels, the gas needs to be separated from other substances in emissions from industrial and combustion processes, such as sulfur dioxide. The concentration of CO2 in power plant flue gases is relatively low (<15 vol%) (Chapel et al., 1999); for process-related emissions, e.g., in the lime and cement industry, CO2 concentrations are somewhat higher (14–33 vol%) (Bosoaga et al., 2009) (see Table 1). In this study, we assume that 90% of the CO2 from medium- (15–90 vol%) and low- (<15 vol%) concentration CO2 sources is recoverable (Chapel et al., 1999). Current CO2 capture technologies do not usually capture all the CO2 as this is too expensive and requires too much energy.
In biofuels production processes (fermentation, anaerobic digestion, gasification), relatively pure streams (>90 vol%) of CO2 are available in latter cases due to the demand for high fuel purity in the transport sector. We assume that 100% of the CO2 from biofuel plants is recoverable and could be converted into fuel. Approximately 54% of the biogas produced in Sweden is upgraded for the transportation sector (Swedish Energy Agency and Energigas Sverige, 2016), which means that CO2 capturing technology already exist on several Swedish anaerobic digestion facilities. Another opportunity for anaerobic digestion-based biogas plants is to feed raw biogas to a methanation reactor, thereby combining biogas upgrading and electrofuels production (Johannesson, 2016). Biogas plants that currently do not upgrade their gas are generally small implying high costs for upgrading and currently supplying other markets than the transport sector, making them less suitable as a source of CO2 for electrofuels production. Therefore, only CO2 from biogas-upgrading plants is considered in this study. For simplicity, we assume that the share of upgraded biogas of total biogas production by 2030 remains at 54%.
Geographic Distribution of CO2 Emissions
The CO2 emission sources have been mapped and categorized by concentration and geographical area. The geographical areas are those used for the Swedish electricity market, i.e., four price areas (SE1, SE2, SE3, and SE4) (Swedish Energy Markets Inspectorate, 2014) (see Figure 1). The electricity price areas were implemented in Sweden in order to control the transmission of electricity between regions and to promote the construction of power generation and transmission capacity in and to areas with electricity deficits. On average, the northern parts of the country (SE1 and SE2) are characterized by an excess of electricity production due to the available hydropower resources and relatively low overall power consumption. In the southern parts (SE3 and SE4), electricity consumption often exceeds production, which leads to relatively higher electricity prices in these areas (Nord Pool, 2016).
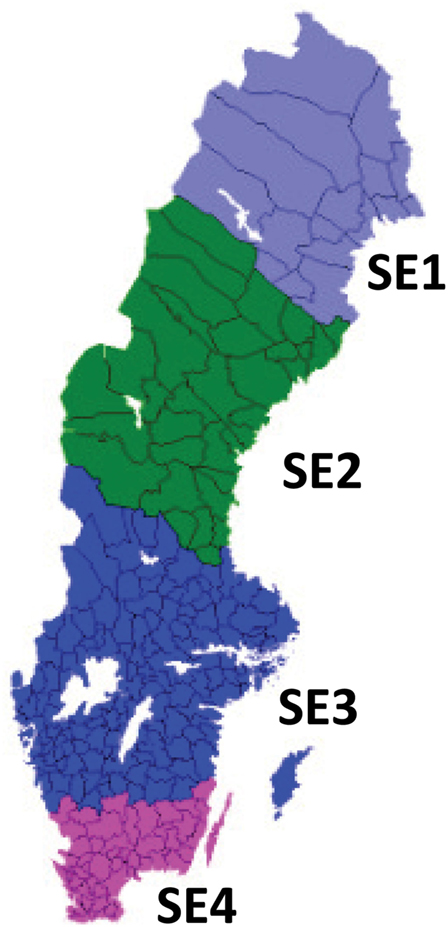
Figure 1. The electricity price areas (SE1, SE2, SE3, and SE4) in Sweden, which are used to illustrate the geographic distribution of the CO2 emissions. Figure based on SCB (2015).
Electrofuel-Production Efficiency and Cost
The focus in this study is on electrofuels in the form of methane, methanol, and DME since these are the most discussed electrofuels in the literature (see text footnote 1), are of interest for the relevant transport sector (shipping and trucks), and include fuels in liquid and gaseous form. The amounts of CO2 and electricity necessary for the types of electrofuels included in this study are given in Table 4 and are based on lower heating value (LHV).

Table 4. Estimated values for CO2 and electricity demand per unit of electrofuel and production cost for 2015 and 2030 (based on literature review and base case reference scenario by Brynolf et al. (see text footnote 1) representing average data and based on lower heating value, for assumptions see the text).
Table 4 also presents cost ranges for 2015 and 2030 estimated in the base case reference scenario in Brynolf et al. (see text footnote 1). The electricity-to-fuel efficiency of the electrofuel-production process strongly depends on the type of electrolyzer and the future development of production technologies. Alkaline electrolysers have efficiencies in the range of 43–69% today, while the most efficient electrolysers are expected to reach efficiencies above 80% based on LHV (Smolinka et al., 2011; Benjaminsson et al., 2013; Grond et al., 2013; Mathiesen et al., 2013; Bertuccioli et al., 2014; Hannula, 2015; Schiebahn et al., 2015). Combining this with the efficiency for fuel synthesis yields electricity-to-fuel efficiencies in the 30–75% range for methane, methanol, and DME, this corresponds to an electricity demand of 1.33–3.33 MWh electricity/MWh electrofuel.
Brynolf et al. (see text footnote 1) suggest costs for different electrofuels (methane, methanol, DME, gasoline, and diesel) in the span of 120€2015–1,050€2015/MWhfuel and 100€2015–430€2015/MWhfuel in 2015 and 2030, respectively. However, in the base case of the reference scenario representing average data, the same costs are 200€2015–280€2015/MWhfuel and 160€2015–210€2015/MWhfuel in 2015 and 2030, respectively. The most important factors affecting the production cost of electrofuels are the capital cost of the electrolyzer, the electricity price, the capacity factor of the unit, and the lifetime of the electrolyzer. The base case reference scenario assumes alkaline electrolyzer with a capital cost of 600€2015/kWel, capacity factor of 80%, lifetime of the electrolyzer at 25 years, carbon capture cost at 30€2015/ton, and electricity price of 50€2015/MWh. A capacity factor at 80% implies that the plant is run the major part of the year. However, if electrofuels are used to balance intermittent renewable power production (i.e., there is production only when there is a surplus of power from these sources), the capacity factor will be reduced. This will not influence the estimated technical potential for production of electrofuels in Sweden in this study, but it will lead to increased electrofuel-production costs [which is further assessed in Brynolf et al. (see text footnote 1)]. In the case of a carbon capture cost at 10€2015/ton representing more pure streams like biofuels operation, the production cost of electrofuels is reduced by approximately 3%. In their review of the literature, Brynolf et al. (see text footnote 1) also found that the cost of capturing CO2 generally is a minor factor in the total production cost of electrofuels representing less than 10% (when not considering CO2 capturing from air). CO2 can be captured from various industrial sources with costs ranging from about 10€2015 to 170€2015/ton CO2, depending on the CO2 concentration (Damen et al., 2006, 2007; Finkenrath, 2011; Goeppert et al., 2012; Kuramochi et al., 2012, 2013; IEA, 2013; see text footnote 1). This indicates that from an economic point of view, all CO2 sources (except from pure air) might be of interest for electrofuel production in the future.
Results
CO2 Emissions in Sweden
In Sweden, major stationary point sources currently emit approximately 50 Mton CO2 per year. Of this, about 45 Mton CO2 is recoverable (see Figure 2). Our analysis includes 148 facilities, with 14 U emitting more than 1 Mton CO2/year, 88 U emitting between 1 Mton and 100 kton CO2/year, and 47 U emitting less than 100 kton/year.
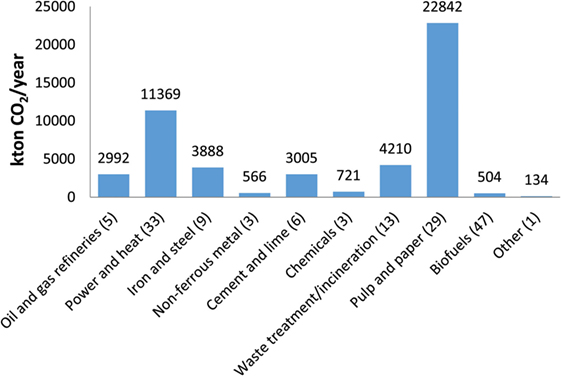
Figure 2. Current recoverable CO2 from major point sources in Sweden, based on European Environment Agency (2015), Grahn and Hansson (2015), and Dahlgren et al. (2013). In total, 149 point sources are included; the number of plants in each category is given in parenthesis.
Figure 2 shows the distribution of CO2 emissions among different types of point sources. Pulp and paper plants and heat and power plants are the two major types of point sources, corresponding to 23 Mton CO2 (45% of the total) and 11.5 Mton CO2 (23% of the total) per year, respectively. In total, biogenic sources account for 65% or 32 Mton of CO2 emissions per year. The high share of biogenic CO2 is mainly due to the extensive use of biomass in producing pulp, paper, heat, and power and from waste treatment and incineration. Emissions from biofuel production represent a small share of the current total amount of available CO2, with approximately 0.5 Mton of recoverable CO2 per year. According to Andreas Gundberg, Innovation manager at Lantmännen Agroetanol, CCU has already been implemented at the main Swedish ethanol producer representing approximately 90% of the total Swedish ethanol production capacity. The emissions from this ethanol production (about 100 kton/year) are included in the analysis.
Figure 3 shows the amount of CO2 available and the corresponding potential production of electrofuels in the form of methanol at different CO2 concentrations in Sweden in 2013 and in 2030. The majority of the CO2 is available at low and medium concentrations, equally spread between the categories low and medium but mainly below 20 vol%. A small share of the CO2, mainly from the biofuels industry, is available at higher, significantly more accessible, concentrations.
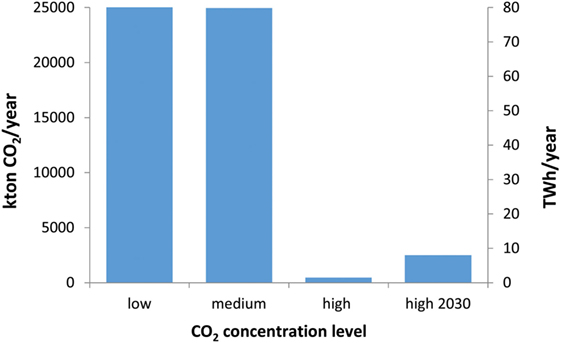
Figure 3. Recoverable CO2 and potential for production of electrofuels in the form of methanol at three different concentration levels (low: <15 vol%, medium: 15–90 vol% and, high: >90 vol%) in 2013 and at high concentration in 2030.
About 90% of the high-concentration emissions come from sources in geographic region SE3, along with about 60% of the rest of the CO2 emission sources (see Figure 4). Anaerobic digestion and ethanol production from agricultural crops currently dominate biofuels production, and these are mostly located in densely populated areas (producing biogas from digestion of sewage sludge and food waste) or in proximity to agricultural operations (farm-based ethanol and biogas production), which are mainly found in southern Sweden. However, electricity prices in the southern parts are currently less favorable than further north where hydropower resources and lower demand create an excess of electricity while the transmission capacity to the southern industrial and population centers is limited.
The projected large-scale introduction of biofuels based on lignocellulosic feedstocks should entail higher shares of high-concentration CO2 emissions in the northern regions, SE1 and SE2, if plants are located near feedstock resources.
The biofuels sector is expected to grow significantly in Sweden during the coming years in order to achieve national climate and transport targets. Figure 5 illustrates the current and estimated amount of CO2 available for electrofuels production from different biofuel production technologies and a minor share of others sources available by 2030 in Sweden based on Dahlgren et al. (2013) and Hansson and Grahn (2013). Only CO2 from the production of upgraded biogas is included. In 2030, the CO2 originates mainly from gasification, anaerobic digestion, and fermentation-based biofuels production (utilizing both cereals and lignocellulosic biomass and considering recent implementation plans). In 2030, these sources could potentially yield 2.2 Mton CO2 for electrofuels production (approximately 5.5 times the amount currently available). The largest increase in production capacity is expected with the large-scale implementation of a variety of biomass-gasification-based biofuels, such as synthetic natural gas, DME, or methanol from lignocellulosic biomass. Ethanol produced from lignocellulosic feedstocks could also potentially generate large amounts of highly concentrated biogenic CO2.
Swedish Production Potential for Electrofuels
Using all the currently recoverable CO2 from the point sources identified in this study to produce electrofuel in the form of methane would yield approximately 224 TWh per year. This corresponds to approximately 2.5 times the current Swedish demand for transportation fuels [approximately 85 TWh per year in 2014 (Swedish Energy Agency, 2015b)]. For electrofuels with lower conversion efficiencies (e.g., methanol and DME), production could instead cover about twice the current demand. Producing 224 TWh per year of electro-methane requires about 448 TWh of electricity (assuming 2 MWhel/MWhfuel), which corresponds to three times the current Swedish electricity generation [149 TWh (Swedish Energy Agency, 2015a)].
The high-concentration sources, represented mainly by biofuel plants, suffice to provide only about 2% of the current demand for transportation fuels (corresponding to 1.5–2/year, see Figure 6). Converting the high-concentration emissions to electrofuels would require about 3–4 TWh of electricity (2–3% of the current national production). In 2030, the potential production of electrofuels in the form of methane, methanol, and DME from high-CO2 sources is 8–11 TWh (see Figure 6). This corresponds to approximately 9–13% of the current demand for transportation fuels and would require about 15–21 TWh of electricity (10–14% of current electricity production).
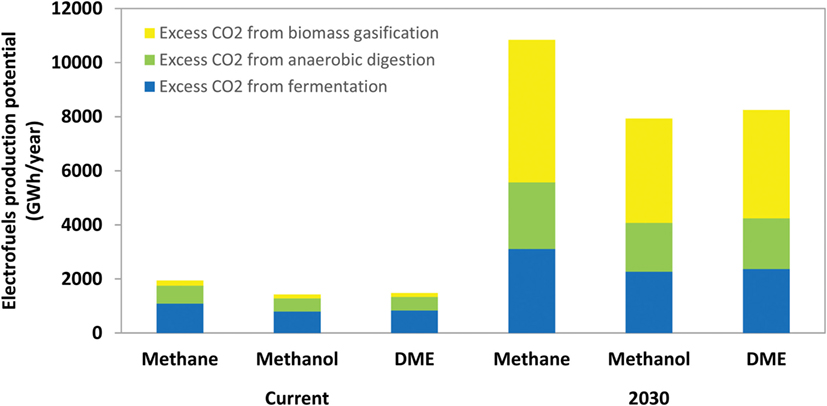
Figure 6. Production potential for electrofuels in the form of methane, methanol and DME from current and future biofuel plants with high CO2 concentrations.
Table 5 shows the requirements for meeting the current Swedish fuel demand for (non-air) transport with electrofuels in the form of methanol. As seen in Table 5, about half of the recoverable CO2 (23 Mton) would be needed to supply the entire current Swedish road transport demand with electrofuels in the form of methanol (assuming a conversion factor of 0.275 ton CO2/MWh methanol). The corresponding amount of CO2 needed to satisfy the entire fuel demand from heavy trucks and all domestic and international shipping currently bunkering in Sweden is estimated to be about 5 and 6 Mton CO2, respectively. This implies that in the case of large-scale introduction of electrofuels for road transport (including heavy trucks), heavy trucks only, or shipping in Sweden, the supply of CO2 is not a limiting factor.
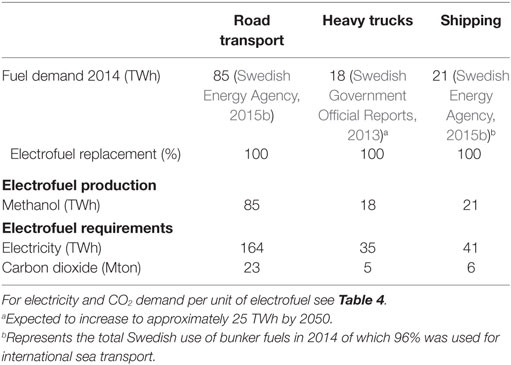
Table 5. Outputs and inputs to electrofuels production if fulfilling the fuel demand with electrofuels in the form of methanol in three different transport modes.
However, meeting the entire current road transport demand with electrofuels would require about 164 TWhel of electricity (with methanol at 1.93 MWhel/MWhfuel). This would more than double the current demand for electricity. To meet the current Swedish fuel demand for passenger cars (at about 41 TWh) (Swedish Government Official Reports, 2013) with electrofuels in the form of methanol would require approximately 11 ton CO2 and 79 TWhel of electricity. For comparison, if the entire passenger car fleet were replaced by electric vehicles, the increased demand for electricity would be approximately 10 TWh (based on Swedish Government Official Reports, 2013).
Using electrofuels for the heavy truck sector and for shipping bunker fuel sold in Sweden would require about 35 and 41 TWhel, respectively. For comparison, in 2014, domestic power generation was 150 TWh (SCB, 2016). Further, the goal is to increase domestic generation from renewable sources by about 30 TWh by 2020, compared to 2002 figures and current production of renewable electricity is approximately 85 TWh (SCB, 2016). Large-scale introduction of electrofuels would require a major increase in the supply of electricity from renewable energy sources.
Discussion and Conclusion
This study shows that Swedish point sources emit approximately 50 million metric tons of CO2 per year, 65% of which is biogenic in origin. The potentially recoverable emissions amount to 45 Mton. The main point sources are in the pulp and paper industry along with heat and power, while emissions from biofuel production (with relatively high concentrations of recoverable CO2) amounted to 0.5 Mton CO2 in 2015, with an estimated potential for 2.2 Mton CO2 in 2030. Thus, the potential streams of relatively pure CO2 are modest, at least in the near term. Currently, the potential yield from these sources is 1.5–2 TWh of electrofuels per year, corresponding to approximately 2% of the current Swedish demand for transportation fuels.
However, in Sweden, all types of CO2 emissions, whether fossil or biogenic, and whether low-concentration or high, are of interest in terms of CCU (although carbon capture can be expected to first be applied to systems with higher concentrations of CO2 because capture costs are somewhat lower for these, generally speaking). In the case of electrofuels, as mentioned earlier, it has been indicated that the cost for carbon capture represents a relatively modest share of the total electrofuel-production cost which makes the purity of the CO2 sources less important. However, CO2 from biofuel operations seem like an attractive source since biofuel actors strive to reduce their CO2 emissions due to sustainability requirements. Further, biomass-related CO2 emissions are expected to increase in the future, since the use of biomass for energy is expected to increase while fossil CO2 emissions are expected to decrease.
We conclude that the Swedish supply of CO2 does not have to be a limiting factor for the potential future production of electrofuels for the Swedish transport sector, even if the current supply of pure CO2 streams is limited. However, there might be other limiting factors such as the associated electricity demand.
As indicated in the introduction, electrofuels represent a potential long-term energy storage option and could, therefore, be of interest in terms of managing grid-integration of more intermittent renewable energy sources (e.g., wind and solar power). But large-scale introduction of electrofuels in the transport sector would in turn represent a huge new demand for electricity. The direct use of electricity needed to supply the entire current transport demand for passenger cars would increase current electricity demand by 10%, while using electrofuels would require increasing the Swedish electricity generation by about 60% to meet the same transport demand (Swedish Energy Agency, 2015b). The electrofuels production process and combustion engine are simply that much less efficient than electric motors. Therefore, large-scale introduction of electrofuels might potentially increase the challenge of balancing intermittent renewable generation, rather than help solve it with long-term energy storage, since an increased demand for power would most likely be met with new wind power installations in Sweden. Producing electrofuels only part of the year is one option to limit this problem. However, according to Brynolf et al. (see text footnote 1), the production cost of electrofuels increases drastically per megawatt hours fuel when the capacity factor (i.e., actual production as share of total production capacity) of the wind turbines is decreased. Thus, the benefit of using electrofuels for balancing renewable energy need to be further assessed.
The production cost of different electrofuels is also a limiting factor for the potential future production of electrofuels in Sweden. The literature contains a fairly broad range of estimates, but the most important factors in the production cost of electrofuels are the capital cost of the electrolyzer, the electricity price, the capacity factor of the unit, and the lifetime of the electrolyzer (see text footnote 1).
The majority of the current CO2 sources are located in southern Sweden, which is also the case for the current CO2 sources with relatively pure CO2 emissions. However, from the perspective of the electric-grid, electrofuels production may be more suitable in the northern parts of Sweden where there is generally a surplus of power generation and lower electricity prices. An increasing demand for electricity in southern Sweden might put additional pressure on the transmission capacity from north to south. Future biofuel plants based on forest biomass (as included in the 2030 scenario) are expected to be located mostly in northern Sweden and, therefore, represent an interesting source of CO2 for production of electrofuels.
From a climate perspective, it might be preferable to capture and store CO2 underground, using CCS technology, and not convert CO2 into a fuel that after combustion will be released to the atmosphere again (van der Giesen et al., 2014; Sternberg and Bardow, 2015). If the CO2 has been captured from burning fossil fuels, CCS will avoid increased CO2 concentration, and if the CO2 is captured from burning biomass (or from air), CCS will decrease the atmospheric CO2 concentration, ceteris paribus. Today, however, there are several obstacles that have to be overcome before CCS could be available at a large scale, including public acceptance (Oltra et al., 2010; Dütschke, 2011). CCS is also only applicable for relatively large CO2 sources and storage possibilities depend on geological prerequisites.
The overall impact on CO2 emissions of the production and use of electrofuels mainly depends on the electricity-related CO2 emissions and what the fuels replace (van der Giesen et al., 2014; Sternberg and Bardow, 2015). van der Giesen et al. (2014) conclude that for some production paths, the climate impact is worse than for fossil fuels, and achieving a net climate benefit requires using renewable electricity and renewable CO2 sources. Sternberg and Bardow (2015) evaluate electrofuels relative to the case in which the same amount of CO2 is instead either emitted or stored. They find that electrofuels can at best only make a small contribution to mitigation compared to other available solutions and that using CO2 emissions for electrofuels is worse from a climate perspective compared to storing them. It would be interesting to more thoroughly study the environmental impact of electrofuels compared to other CCU technologies with a lifecycle perspective. For example, the amount of CO2 emissions from electricity production will depend on (i) the time perspective (for example using a marginal or average electricity mix) and (ii) the geographical boundaries of the electricity supply. However, GHG emissions from electricity production are expected to decrease significantly as a consequence of stringent energy and climate policies changing the mix of energy sources.
To summarize, electrofuels are limited by electricity demand rather than the demand for CO2 and, at scale, require a substantial amount of renewable electricity at relatively low cost. The GHG impact of electrofuels compared to other options, in particular CCS, needs to be further assessed.
Author Contributions
JH is the main author; planned the work and led the writing. RH was responsible for the mapping and quantification of the major Swedish point sources of CO2 emissions and contributed to further assessments and paper writing. SB, MT, and MG contributed with the electrofuel-production characteristics, participated in the assessment, and contributed to paper writing.
Conflict of Interest Statement
The authors declare that the research was conducted in the absence of any commercial or financial relationships that could be construed as a potential conflict of interest.
Acknowledgments
Financial support from the Swedish Research Council Formas, Nordic Energy Research through the Nordic flagship project Shift (Sustainable Horizons for Transport), the Swedish Energy Agency, and the Swedish Knowledge Centre for Renewable Transportation Fuels (f3) is acknowledged. This publication is partly the result of a project within the Renewable Fuels and Systems Program (Samverkansprogrammet Förnybara drivmedel och system), financed by the Swedish Energy Agency and the Swedish Knowledge Centre for Renewable Transportation Fuels (f3). The f3 Centre contributes, through knowledge based on science, to the development of environmentally, economically, and socially sustainable and renewable transportation fuels, as part of a future sustainable society (see www.f3centre.se). The authors also thank Magnus Fröberg, Scania CV AB, and Paulina Essunger for valuable input.
Funding
This work has received financial support from (i) the Swedish Research Council Formas via the project titled “Cost-effective choices of marine fuels under stringent carbon dioxide reduction targets,” (ii) Nordic Energy Research through the Nordic flagship project Shift (Sustainable Horizons for Transport), and (iii) the Swedish Knowledge Centre for Renewable Transportation Fuels and the Swedish Energy Agency via the project titled “The role of electrofuels: a cost-effective solution for future transport?”
Footnote
- ^Brynolf, S., Taljegård, M., Grahn, M., and Hansson, J. (2017). Electrofuels for the transport sector: a review of production costs. Renew. Sust. Energ. Rev. (Submitted).
References
Arvidsson, M., and Lundin, B. (2011). Process Integration Study of a Biorefinery Producing Ethylene from Lignocellulosic Feedstock for a Chemical Cluster. Master thesis, Chalmers University of Technology, Gothenburg, Sweden.
Axelsson, P., Cederlöf, F., and Forslöf, S. (2014). Carbon Dioxide Capture from the Ethanol Industry and Use in Industrial Applications. B.Sc. thesis, Linköping University, Linköping, Sweden.
Benjaminsson, G., Benjaminsson, J., and Rudberg, R. B. (2013). Power to Gas – A Technical Review (El till gas – system, ekonomi och teknik). Malmö, Sweden: Svenskt Gastekniskt Center AB.
Bertuccioli, L., Chan, A., Hart, D., Lehner, F., Madden, B., and Standen, E. (2014). Development of Water Electrolysis in the European Union, Fuel Cells and Hydrogen Joint Undertaking. Lausanne, Switzerland. Available at: http://www.fch.europa.eu/sites/default/files/study%20electrolyser_0-Logos_0.pdf
Bosoaga, A., Masek, O., and Oakey, J. E. (2009). CO2 capture technologies for cement industry. Energy Procedia 1, 133–140. doi:10.1016/j.egypro.2009.01.020
Chapel, D. G., Mariz, C. L., and Ernest, J. (1999). Recovery of CO2 from Flue Gases: Commercial Trends. Available at: http://citeseerx.ist.psu.edu/viewdoc/download?doi=10.1.1.204.8298&rep=rep1&type=pdf
Chen, G., and Chen, Z. (2010). Carbon emissions and resources use by Chinese economy 2007: a 135-sector inventory and input-output embodiment. Commun. Nonlinear Sci. Numer. Simulat. 15, 3647–3732. doi:10.1016/j.cnsns.2009.12.024
Chen, Q., Lv, M., Tang, Z., Wang, H., Wei, W., and Sun, Y. (2016). Opportunities of integrated systems with CO2 utilization technologies for green fuel & chemicals production in a carbon-constrained society. J. CO2 Utilization 14, 1–9. doi:10.1016/j.jcou.2016.01.004
Connolly, D., Mathiesen, B. V., and Ridjan, I. (2014). A comparison between renewable transport fuels that can supplement or replace biofuels in a 100% renewable energy system. Energy 73, 110–125. doi:10.1016/j.energy.2014.05.104
Cuéllar-Franca, R. M., and Azapagic, A. (2015). Carbon capture, storage and utilisation technologies: a critical analysis and comparison of their life cycle environmental impacts. J. CO2 Utilization 9, 82–102. doi:10.1016/j.jcou.2014.12.001
Dahlgren, S., Liljeblad, A., Cerruto, J., Nohlgren, I., and Starberg, K. (2013). Realiserbar biogaspotential i Sverige år 2030 genom rötning och förgasning. Stockholm, Sweden: WSP.
Damen, K., van Troost, M., Faaij, A., and Turkenburg, W. (2006). A comparison of electricity and hydrogen production systems with CO2 capture and storage. Part A: review and selection of promising conversion and capture technologies. Prog. Energy Combust. Sci. 32, 215–246. doi:10.1016/j.pecs.2005.11.005
Damen, K., van Troost, M., Faaij, A., and Turkenburg, W. (2007). A comparison of electricity and hydrogen production systems with CO2 capture and storage-Part B: chain analysis of promising CCS options. Prog. Energy Combust. Sci. 33, 580–609. doi:10.1016/j.pecs.2007.02.002
de Boer, H. S., Grond, L., Moll, H., and Benders, R. (2014). The application of power-to-gas, pumped hydro storage and compressed air energy storage in an electricity system at different wind power penetration levels. Energy 72, 360–370. doi:10.1016/j.energy.2014.05.047
Dütschke, E. (2011). What drives local public acceptance – comparing two cases from Germany. Energy Procedia 4, 6234–6240. doi:10.1016/j.egypro.2011.02.636
European Environment Agency. (2015). European Pollutant Release and Transfer Register. Available at: http://prtr.ec.europa.eu/#/home
Finkenrath, M. (2011). Cost and Performance of Carbon Dioxide Capture from Power Generation. Paris, France: International Energy Agency (IEA).
Gahleitner, G. (2013). Hydrogen from renewable electricity: an international review of power-to-gas pilot plants for stationary applications. Int. J. Hydrogen Energy 38, 2039–2061. doi:10.1016/j.ijhydene.2012.12.010
Ganesh, I. (2013). Conversion of carbon dioxide into several potential chemical commodities following different pathways-a review. Mater. Sci. Forum 764, 1–82. doi:10.4028/www.scientific.net/MSF.764.1
Goeppert, A., Czaun, M., Surya Prakash, G. K., and Olah, G. A. (2012). Air as the renewable carbon source of the future: an overview of CO2 capture from the atmosphere. Energy Environ. Sci. 5, 7833–7853. doi:10.1039/C2EE21586A
Government offices of Sweden. (2009). Regeringens Proposition (Government Bill) 2008/2009:163: En sammanhållen Klimat och Energipolitik – Energi respektive Klimat (A Coherent Climate and Energy Policy – Energy and Climate Respectively). Available at: www.regeringen.se/rattsdokument/proposition/2009/03/prop.-200809163/ and www.regeringen.se/rattsdokument/proposition/2009/03/prop.-200809162/
Grahn, M., and Hansson, J. (2015). Prospects for domestic biofuels for transport in Sweden 2030 based on current production and future plans. Wiley Interdiscip. Rev. Energy Environ. 4, 290–306. doi:10.1002/wene.138
Graves, C., Ebbesen, S. D., Mogensen, M., and Lackner, K. S. (2011). Sustainable hydrocarbon fuels by recycling CO2 and H2O with renewable or nuclear energy. Renew. Sustain. Energ. Rev. 15, 1–23. doi:10.1016/j.rser.2010.07.014
Grond, L., Schulze, P., and Holstein, S. (2013). Systems Analyses Power to Gas: Deliverable 1: Technology Review. Groningen, the Netherlands: DNV KEMA Energy & Sustainability.
Hannula, I. (2015). Co-production of synthetic fuels and district heat from biomass residues, carbon dioxide and electricity: performance and cost analysis. Biomass Bioeng. 74, 26–46. doi:10.1016/j.biombioe.2015.01.006
Hannula, I. (2016). Hydrogen enhancement potential of synthetic biofuels manufacture in the European context: a techno-economic assessment. Energy 104, 199–212. doi:10.1016/j.energy.2016.03.119
Hannula, I., and Kurkela, E. (2013). Liquid Transportation Fuels via Large-Scale Fluidised-Bed Gasification of Lignocellulosic Biomass. VTT Technical Research Centre of Finland Report. Available at: http://www.ieatask33.org/download.php?file=files/file/publications/new/Techno-economics.pdf
Hansson, J., and Grahn, M. (2013). Utsikt för förnybara drivmedel i Sverige, IVL report B 2083. Available at: http://spbi.se/wp-content/uploads/2013/03/IVL_B2083_2013_final.pdf
Heyne, S. (2013). Bio-SNG from Thermal Gasification-Process Synthesis, Integration and Performance. Doctoral thesis, Linköping University, Gothenburg, Sweden.
Johannesson, T. (2016). Implementation of Electrofuel Production at a Biogas Plant – Case Study at Borås. Master thesis, Chalmers University of Technology, Gothenburg, Sweden.
König, D. H., Baucks, N., Dietrich, R. U., and Wörner, A. (2015). Simulation and evaluation of a process concept for the generation of synthetic fuel from CO2 and H2. Energy 91, 833–841. doi:10.1016/j.energy.2015.08.099
Kötter, E., Schneider, L., Sehnke, F., Ohnmeiss, K., and Schröer, R. (2016). The future electric power system: impact of power-to-gas by interacting with other renewable energy components. J. Energy Storage 5, 113–119. doi:10.1016/j.est.2015.11.012
Kuramochi, T., Ramírez, A., Turkenburg, W., and Faaij, A. (2012). Comparative assessment of CO2 capture technologies for carbon-intensive industrial processes. Prog. Energy Combust. Sci. 38, 87–112. doi:10.1016/j.pecs.2011.05.001
Kuramochi, T., Ramírez, A., Turkenburg, W., and Faaij, A. (2013). Techno-economic prospects for CO2 capture from distributed energy systems. Renew. Sustain. Energy Rev. 19, 328–347. doi:10.1016/j.rser.2012.10.051
Larsson, M., Grönkvist, S., and Alvfors, P. (2015). Synthetic fuels from electricity for the Swedish transport sector: comparison of well to wheel energy efficiencies and costs. Energy Procedia 75, 1875–1880. doi:10.1016/j.egypro.2015.07.169
Liu, L. C., Wang, J. N., Wu, G., and Wei, Y. M. (2010). China’s regional carbon emissions change over 1997–2007. Int. J. Energy Environ. 1, 161–176.
Mathiesen, B. V., Ridjan, I., Connolly, D., Nielsen, M. P., Vang Hendriksen, P., Bjerg Mogensen, M., et al. (2013). Technology Data for High Temperature Solid Oxide Electrolyser Cells, Alkali and PEM Electrolysers. Aalborg University, Denmark: Department of Development and Planning.
Mignard, D., and Pritchard, C. (2008). On the use of electrolytic hydrogen from variable renewable energies for the enhanced conversion of biomass to fuels. Chem. Eng. Res. Des. 86, 473–487. doi:10.1016/j.cherd.2007.12.008
Mohseni, F. (2012). Power to Gas – Bridging Renewable Electricity to the Transport Sector. Licentitate thesis, KTH Royal Institute of Technology, Stockholm, Sweden.
Naims, H. (2016). Economics of carbon dioxide capture and utilization – a supply and demand perspective. Environ. Sci. Pollut. Res. 23, 22226–22241. doi:10.1007/s11356-016-6810-2
Nikoleris, A., and Nilsson, L. (2013). Elektrobränslen en kunskapsöversikt (Electrofuels An Overview). IMES Report Series, Vol. 85. Lund, Sweden: Lund University. Available at: http://portal.research.lu.se/portal/files/3697397/3738230.pdf
Nord Pool. (2016). Historical Market Data. Available at: http://www.nordpoolspot.com/historical-market-data/
Oltra, C., Sala, R., Solà, R., Di Masso, M., and Rowe, G. (2010). Lay perceptions of carbon capture and storage technology. Int. J. Greenhouse Gas Control 4, 698–706. doi:10.1016/j.ijggc.2010.02.001
Pachauri, R. K., Allen, M. R., Barros, V. R., Broome, J., Cramer, W., Christ, R., et al. (2014). Climate Change 2014: Synthesis Report. Contribution of Working Groups I, II and III to the Fifth Assessment Report of the Intergovernmental Panel on Climate Change, eds R. Pachauri, and L. Meyer (Geneva, Switzerland: IPCC), 151.
Perathoner, S., and Centi, G. (2014). CO2 recycling: a key strategy to introduce green energy in the chemical production chain. ChemSusChem 7, 1274–1282. doi:10.1002/cssc.201300926
Pettersson, K., and Harvey, S. (2012). Comparison of black liquor gasification with other pulping biorefinery concepts – systems analysis of economic performance and CO2 emissions. Energy 37, 136–153. doi:10.1016/j.energy.2011.10.020
Qadrdan, M., Abeysekera, M., Chaudry, M., Wu, J., and Jenkins, N. (2015). Role of power-to-gas in an integrated gas and electricity system in Great Britain. Int. J. Hydrogen Energy 40, 5763–5775. doi:10.1016/j.ijhydene.2015.03.004
Reiter, G., and Lindorfer, J. (2015). Evaluating CO2 sources for power-to-gas applications – a case study for Austria. J. CO2 Utilization 10, 40–49. doi:10.1016/j.jcou.2015.03.003
Ridjan, I., Mathiesen, B. V., and Connolly, D. (2014). Synthetic fuel production costs by means of solid oxide electrolysis cells. Energy 76, 104–113. doi:10.1016/j.energy.2014.04.002
SCB. (2015). Energy Prices and Switching of Suppliers, 3rd Quarter 2015 (Prisutveckling på energi samt leverantörsbyten, tredje kvartalet 2015). Stockholm, Sweden: Statistics Sweden. Available at: www.scb.se/Statistik/EN/EN0304/2015K03/EN0304_2015K03_SM_EN24SM1504.pdf
SCB. (2016). Tillförsel och användning av el 2001–2015 (GWh) (Production and Use of Electricity 2001-2015). Available at: http://www.scb.se/hitta-statistik/statistik-efter-amne/energi/tillforsel-och-anvandning-av-energi/arlig-energistatistik-el-gas-och-fjarrvarme/tillforsel-och-anvandning-av-el-20012015-gwh/
Schiebahn, S., Grube, T., Robinius, M., Tietze, V., Kumar, B., and Stolten, D. (2015). Power to gas: technological overview, systems analysis and economic assessment for a case study in Germany. Int. J. Hydrogen Energy 40, 4285–4294. doi:10.1016/j.ijhydene.2015.01.123
SGC. (2012). Basic Data on Biogas. Report. Malmö, Sweden: Svenskt gastekniskt centrum. Available at: http://www.sgc.se/ckfinder/userfiles/files/BasicDataonBiogas2012.pdf
Smolinka, T., Günther, M., and Garche, J. (2011). Stand und Entwicklungspotenzial der Wasserelektrolyse zur Herstellung von Wasserstoff aus regenerativen Energien, Kurzfassung des Abschlussberichtes NOW-Studie. Freiburg im Breisgau: Fraunhofer ISE and FCBAT. Available at: http://www.hs-ansbach.de/uploads/tx_nxlinks/NOW-Studie-Wasserelektrolyse-2011.pdf
ST1. (2016). St1 Built a Waste-Based Etanolix® Ethanol Production Plant in Gothenburg. Available at: http://www.st1.eu/news/st1-built-a-waste-based-etanolix-ethanol-production-plant-in-gothenburg
Sternberg, A., and Bardow, A. (2015). Power-to-what? – environmental assessment of energy storage systems. Energy Environ. Sci. 8, 389–400. doi:10.1039/C4EE03051F
Streibel, M., Nakaten, N., Kempka, T., and Kühn, M. (2013). Analysis of an integrated carbon cycle for storage of renewables. Energy Procedia 40, 202–211. doi:10.1016/j.egypro.2013.08.024
Swedish Energy Agency. (2015a). Energy in Sweden 2015. Eskilstuna, Sweden: Swedish Energy Agency. Available at: https://www.energimyndigheten.se/globalassets/statistik/overgripande-rapporter/energy-in-sweden-till-webben.pdf
Swedish Energy Agency. (2015b). Transportsektorns energianvändning 2014 (Energy Use in the Transport Sector 2014). Eskilstuna, Sweden: Swedish Energy Agency.
Swedish Energy Agency and Energigas Sverige. (2015). Produktion och användning av biogas och rötrester år 2014, ES 2015:03. Available at: https://www.energimyndigheten.se/globalassets/nyheter/2015/produktion-och-anvandning-av-biogas-och-rotrester-ar-2014.pdf
Swedish Energy Agency and Energigas Sverige. (2016). Produktion och användning av biogas och rötrester år 2015, ES 2016:04. Available at: https://www.energimyndigheten.se/globalassets/nyheter/2016/es-2016-04-produktion-och-anvandning-av-biogas-och-rotrester-ar-2015.pdf
Swedish Energy Markets Inspectorate. (2014). Sverige är indelat i fyra elområden. Fact Sheet. Available at: http://ei.se/Documents/Publikationer/fakta_och_informationsmaterial/Elomraden.pdf
Swedish Government Official Reports. (2013). Fossilfrihet på väg (On Its Way to Fossil Fuel Independence). SOU 2013:84. Available at: http://www.regeringen.se/rattsdokument/statens-offentliga-utredningar/2013/12/sou-201384/
Swedish Government Official Reports. (2016). En klimat – och luftvårdsstrategi för Sverige – Del 1 (A Climate and Air Pollution Treatment Strategy for Sweden – Part 1). Delbetänkande av Miljömålsberedningen, Swedish Government Official Reports SOU 2016:47. Available at: http://www.regeringen.se/rattsdokument/statens-offentligautredningar/2016/06/en-klimat–och-luftvardsstrategi-for-sverige/
Taljegård, M., Brynolf, S., Hansson, J., Hackl, R., Grahn, M., and Andersson, K. (2015). “Electrofuels – a possibility for shipping in a low carbon future?” in Proceedings of International Conference on Shipping in Changing Climates. Glasgow, 405–418.
Tremel, A., Wasserscheid, P., Baldauf, M., and Hammer, T. (2015). Techno-economic analysis for the synthesis of liquid and gaseous fuels based on hydrogen production via electrolysis. Int. J. Hydrogen Energy 40, 11457–11464. doi:10.1016/j.ijhydene.2015.01.097
Trost, T., Jentsch, M., and Sterner, M. (2012). Erneuerbares Methan: Analyse der CO2-Potenziale für Power-to-Gas Anlagen in Deutschland. Zeitschrift für Energiewirtschaft 36, 173–190. doi:10.1007/s12398-012-0080-6
van der Giesen, C., Kleijn, R., and Kramer, G. J. (2014). Energy and climate impacts of producing synthetic hydrocarbon fuels from CO2. Environ. Sci. Technol. 48, 7111–7121. doi:10.1021/es500191g
Vandewalle, J., Bruninx, K., and D’haeseleer, W. (2014). The Interaction of a High Renewable Energy/Low Carbon Power System with the Gas System through Power to Gas. Rome, 28–31.
Varone, A., and Ferrari, M. (2015). Power to liquid and power to gas: an option for the German Energiewende. Renew. Sustain. Energ. Rev. 45, 207–218. doi:10.1016/j.rser.2015.01.049
Wismans, J., Grahn, M., and Denbratt, I. (2016). Low-Carbon Transport: Health and Climate Benefits. Background Report to Intergovernmental Ninth Regional Environmentally Sustainable Transport (EST) Forum in Asia. Gothenburg: Chalmers University of Technology.
Xu, Y., Isom, L., and Hanna, M. A. (2010). Adding value to carbon dioxide from ethanol fermentations. Bioresour. Technol. 101, 3311–3319. doi:10.1016/j.biortech.2010.01.006
Zakeri, B., and Syri, S. (2015). Electrical energy storage systems: a comparative life cycle cost analysis. Renew. Sustain. Energ. Rev. 42, 569–596. doi:10.1016/j.rser.2014.10.011
Zhang, B., and Chen, G. (2014). China’s CH4 and CO2 emissions: bottom-up estimation and comparative analysis. Ecol. Indicators 47, 112–122. doi:10.1016/j.ecolind.2014.01.022
Keywords: carbon dioxide, CO2 recovering, carbon capture and utilization, carbon recycling, power-to-gas, alternative transportation fuels
Citation: Hansson J, Hackl R, Taljegard M, Brynolf S and Grahn M (2017) The Potential for Electrofuels Production in Sweden Utilizing Fossil and Biogenic CO2 Point Sources. Front. Energy Res. 5:4. doi: 10.3389/fenrg.2017.00004
Received: 23 December 2016; Accepted: 23 February 2017;
Published: 13 March 2017
Edited by:
Katy Armstrong, University of Sheffield, UKReviewed by:
Hyungwoong Ahn, University of Edinburgh, UKAdam Hughmanick Berger, Electric Power Research Institute, USA
Copyright: © 2017 Hansson, Hackl, Taljegard, Brynolf and Grahn. This is an open-access article distributed under the terms of the Creative Commons Attribution License (CC BY). The use, distribution or reproduction in other forums is permitted, provided the original author(s) or licensor are credited and that the original publication in this journal is cited, in accordance with accepted academic practice. No use, distribution or reproduction is permitted which does not comply with these terms.
*Correspondence: Julia Hansson, julia.hansson@ivl.se