- 1Graduate School of Engineering, Mie University, Tsu, Japan
- 2Department of Physics, Faculty of Engineering, Jiangsu University, Zhenjiang, China
- 3Group 4, Component Engineering Development Department, Suzuki Motor Corporation, Hamamatsu, Japan
- 4Department of Physics, Zhejiang University, Hangzhou, China
A water-stable solid electrolyte is a key material without which aqueous lithium–air batteries could not be operated. In this study, we have examined the electrical conductivity and mechanical properties of a water-stable lithium-ion-conducting solid electrolyte, Li1+xAlxGeyTi2−x−y(PO4)3 with the NASICON-type structure, as a function of the Al and Ge content. Li1+xAlxGeyTi2−x−y(PO4)3 was synthesized by the conventional solid-state reaction method. The highest lithium-ion conductivity of 1.0 × 10−3 S cm−1 at 25°C and the highest three-point bending strength of 90 N mm−2 at room temperature were observed for a pellet of Li1.45Al0.45Ge0.2Ti1.35(PO4)3 sintered at 900°C.
Introduction
In the last half century, many types of lithium-ion-conducting solid electrolytes have been reported, such as Li3N (Alpen et al., 1977), B2S3–Li2S–LiI glass (Wada et al., 1983), NASICON-type Li1+xAxTi2−x(PO4)4 (Aono et al., 1990), perovskite-type La2/3−xLixTiO3 (Inaguma et al., 1993), garnet-type Li7La3Zr2O12 (Murugan et al., 2007), and thio-LISICON-type Li10GeP2S12 (Kamaya et al., 2011). At present, the highest lithium-ion conductivity of 1.2 × 10−2 S cm−1 at room temperature is reported in Li10GeP2S12, which is higher than that of conventional liquid electrolytes, because its lithium-ion transport number is unity. Lithium-ion-conducting solid electrolytes are generally hygroscopic and so are difficult to handle in the open atmosphere, especially the high conductivity sulfide-based solid electrolytes. NASICON-type Li1+xAxTi2−x(PO4)3 lithium-conducting solid electrolytes are less sensitive to moisture and can be prepared in the open air, and are also stable in contact with LiCl-saturated aqueous solution (Shimonishi et al., 2011). Aono et al. (1990) has reported the electrical conductivity of the Li1+xAxTi2−x(PO4)3 (A = Al, Cr, Ga, Fe, In, La, Sc, and Y) system and found the highest electrical conductivity of 7 × 10−4 S cm−1 at 25°C for Li1.3Al0.3Ti1.7(PO4)3.
Since the report by Aono et al., the NASICON-type lithium-ion-conducting solid electrolytes have been extensively examined. The highest electrical conductivity of 4.62 × 10−3 S cm−1 at 27°C was reported for the Li1.5Al0.5Ge1.5(PO4)3 glass–ceramic by Thokchom and Kumar (2010). However, Fu (1997) studied the Li1−xAlxGe2−x(PO4)3 glass–ceramics and found the electrical conductivity of Li1.5Al0.5Ge1.5(PO4)3 was 4.0 × 10−4 S cm−1 at 25°C, and also Xu et al. (2007) found an electrical conductivity of 7.25 × 10−4 S cm−1 at room temperature for the Li1.5Al0.5Ge1.5(PO4)3–0.05 Li2O glass–ceramic. The preparation of glass–ceramics is somewhat complex, and the effect of aging on the electrical conductivity is questionable. The Li1+x+yAlx(Ge, Ti)2−xSiyP4−yO12 glass–ceramics have been commercialized by Ohara, Ltd., Japan. The glass–ceramic is water-permeation free, and the electrical conductivity is 10−4 S cm−1 at room temperature. Recently, Zhang et al. (2013) reported that the electrical conductivity of Li1.4Al0.4Ti1.6(PO4)3 was enhanced by a partial substitution of Ge for Ti. The highest electrical conductivity of 1.3 × 10−3 S cm−1 at 25°C was observed in Li1.4Al0.4Ge0.2Ti1.4(PO4)3, where the content of Al was fixed to 0.4, and the Li1.4Al0.4GexTi1.6−x(PO4)3 powders were prepared by a sol–gel method using expensive Ti and Ge alkoxides. In this study, we have examined the electrical conductivity and mechanical properties of the Li1+xAlxGeyTi2−x−y(PO4)3 system in the range of x = 0.30–0.55 and y = 0.1–0.3 using less expensive starting materials. Water-stable high lithium-ion-conducting solid electrolytes have potential applications for aqueous lithium–air batteries (Zhang et al., 2010; Bruce et al., 2012) and aqueous lithium batteries with aqueous cathodes (Lu et al., 2011; Zhao et al., 2013). These electrolytes have been used as a protective layer for the lithium metal electrode to avoid direct contact with the aqueous solution, because lithium metal reacts vigorously with water.
Materials and Methods
The NASICON-type Li1+xAlxGeyTi2−x−y(PO4)3 lithium-ion-conducting solid electrolytes were prepared by conventional solid-state reaction. Corresponding amounts of chemical reagent grade Li2CO3, TiO2, GeO2, Al2O3, and NH4H2PO4 were ball milled with zirconia balls in a zirconia vessel for 2 h at 400 rpm using high energy mechanical milling (HEMM) with a planetary micro mill (Fritsch Pulverisette 7), and the mixed powders were then pressed into pellets at 150 MPa and calcined at 600°C for 4 h. The calcined pellets were reground and again ball milled using HEMM. The obtained powders were isostatically pressed into pellets at 150 MPa and sintered at various temperatures (850–1,000°C) for 7 h. Tape-cast Li1.45Al0.45Ge0.2Ti1.35(PO4)3 films were prepared using a previously reported method (Zhang et al., 2015). Briefly, fine Li1.45Al0.45Ge0.2Ti1.35(PO4)3 powders prepared by the solid-state reaction were dispersed in a mixed solution of ethanol and toluene (1:1 v/v) using menhaden fish oil [2 wt% to Li1.45Al0.45Ge0.2Ti1.35(PO4)3] as a dispersant. The mixed slurry was ball milled for 10 h using HEMM. Polyvinyl alcohol [8 wt% to Li1.45Al0.45Ge0.2Ti1.35(PO4)3] was then added to the mixed slurry as a plasticizer and ball milled for another 12 h. After tape casting, the green sheets were kept in a sealed box with a small amount of ethanol in a refrigerator to slow the drying process at 5°C for 24 h. Several green sheets were hot pressed at 90°C for 10 min and then sintered at 900°C for 7 h.
The crystal structures of sintered samples were analyzed by X-ray diffraction (XRD) analysis using a Rigaku RINT 2500 diffractometer with Cu-Kα radiation in the 2θ range from 10° to 90° at a scanning step rate of 0.02°s−1. The relative density of the sintered samples was estimated from the ratio of the density calculated from the lattice constants and that calculated from the volume and weight of the sintered body. The electrical conductivity of the sintered pellets (ca. 12 mm diameter and 1 mm thick) with gold sputtered electrodes were measured using an impedance phase analyzer (Solartron 1260) in the frequency range of 0.1 Hz–1 MHz with the bias voltage at 10 mV. Bulk and grain boundary conductivities of the sintered samples were estimated from complex impedance plots using Zview 2. Three-point bending strength of the sintered pellets (ca. 0.24 mm thick and ca. 15 mm wide) was measured at room temperature using a materials tester (Shimadzu EZ-SX 500N).
Results and Discussion
Figure 1 shows XRD patterns of the Li1.5Al0.5Ge0.2Ti1.3(PO4)3 samples sintered at various temperatures for 7 h with a silicon internal standard to measure the lattice constant. An impurity phase of AlPO4 was observed for the sample sintered at 850°C. At sintering temperatures as low as 850°C, the reaction was not completed. All diffraction lines of the samples sintered at 900, 950, and 1,000°C could be indexed as the NASICON-type structure (Perez-Estebanez et al., 2014). Figure 2 shows the relative density of Li1.5Al0.5Ge0.2Ti1.3(PO4)3 pellets sintered at various temperatures. The sample with the impurity phase that was sintered at 850°C showed a low relative density of 87%. The highest relative density of 95.5% was observed for the sample sintered at 900°C, and the relative density decreased with further increase of the sintering temperature. The decreasing of the relative density may be due to the evaporation of lithium compounds at these higher temperatures. Figure 3 shows impedance profiles of Li1.5Al0.5Ge0.2Ti1.3(PO4)3 samples (ca. 1 mm thick) sintered at various temperatures and measured at 25°C. The impedance profiles showed a large semicircle followed by a straight line. The semicircle may be attributed to the grain boundary resistance (Bruce and West, 1983). The intercept of the semicircle on the real axis at high frequency represents the bulk resistance, and the diameter of the semicircle indicates the grain boundary resistance. The semicircle due to the bulk resistance was out of the frequency range for the impedance analyzer used. The samples sintered at 900, 950, and 1,000°C showed almost the same bulk conductivity of ca. 2 × 10−3 S cm−1, while the sample sintered at 850°C showed a low bulk conductivity of ca. 10−3 S cm−1. The low bulk conductivity may be due to the non-equilibrium phase of Li1.5Al0.5Ge0.2Ti1.3(PO4)3 prepared at the lower sintering temperature. The grain boundary resistance was dependent on the sintering temperature, and the sample sintered at 900°C with the highest relative density exhibited the lowest grain boundary resistance.
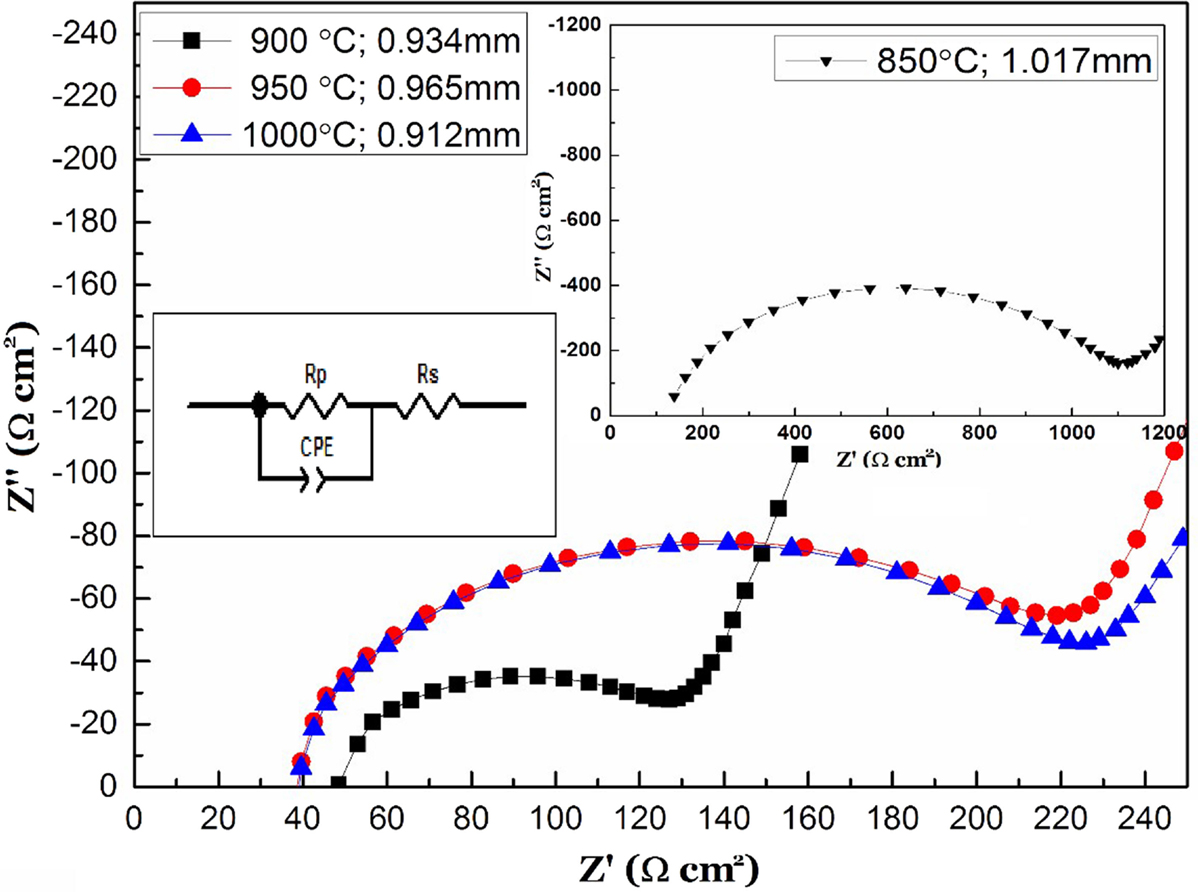
Figure 3. Impedance profiles of Au/Li1.5Al0.5Ge0.2Ti1.3(PO4)3/Au as a function of the sintering temperature.
The electrical conductivity, relative density, and three-point bending strength for the Li1+xAlxGe0.2Ti1.8−x(PO4)3 system sintered at 900°C for 7 h were examined as a function of x. Figure 4 shows the XRD patterns of Li1+xAlxGe0.2Ti1.8−x(PO4)3. Almost all the diffraction lines for Li1+xAlxGe0.2Ti1.8−x(PO4)3 were indexed with the NASICON-type structure. However, Li1+xAlxGe0.2Ti1.8−x(PO4)3 with x = 0.45, 0.5, and 0.55 also showed diffraction lines due to AlPO4. The changes in the lattice parameter with x in Li1+xAlxGe0.2Ti1.8−x(PO4)3 are shown in Figure 5. The a lattice parameter of 0.812 at x = 0.30 increased to 0.882 nm at x = 0.40, and the c lattice parameter of 2.171 nm decreased to 2.043 nm at x = 0.4. Cretin and Fabry (1999) reported that the a parameter decreases and the c parameter increases with increasing x in Li1+xAlxTi2−x(PO4)3, while Aono et al. (1990) found both the a and c parameters decreased with increasing x. The decrease of the c parameter can be attributed to the substitution of Al3+ with a small ionic radius (0.53 nm) for Ti4+ with a large ionic radius (0.605 nm) in the octahedral sites. Several factors play a determinant role in the inference of the cation substitution on the structure (Delmas et al., 1981). Additional Li+ ions are located in the unoccupied Li sites by the substitution of Al3+ for Ti4+ and Ge4+ sites, as observed in Na1+xAlxTi2−x(PO4)3 (Maldonado-Manso et al., 2005), to maintain charge neutrality. The additional lithium ions in these sites lead to repulsion along the a axis. The reason for the smaller c and larger a parameters for Li1.55Al0.55Ge0.2Ti1.25(PO4)3 compared to those for Li1.5Al0.5Ge0.2Ti1.3(PO4)3 is not clear but may be due to the formation of AlPO4 impurity phases. These results suggest that the solubility limit of Al in Li1+xAlxGe0.2Ti1.8−x(PO4)3 is x = 0.4, as observed by Aono et al. (1990) for Li1+xAlxTi2−x(PO4)3. Figure 6 shows impedance profiles for Li1+xAlxGe0.2Ti1.8−x(PO4)3 measured at 25°C as a function of x. The lowest grain boundary resistance was observed for Li1.45Al0.45Ge0.2Ti1.35(PO4)3. The equivalent circuit in Figure 6 assumes a general model comprising grains and uniform grain boundaries that are parallel or perpendicular to the current flow. This results in the one with two parallel resistance–capacitance elements, one for the perpendicular grain boundary (Rp1 and CPE1) and one from the parallel grain boundary (Rp2 and CPE2) connected in parallel. In microcrystalline ceramics, where the effective grain boundary width is negligible compared to the grain size, the contribution of the parallel grain boundary can be neglected. However, parallel grain boundary contribution must be taken into account if the parallel grain boundary conductivity becomes significantly larger than that of the grain and/or if the effective grain boundary width is no longer negligible with respect to the grain size (Bouchet et al., 2003). The change in the grain boundary resistance with x could be explained by the change of the relative density as shown in Figure 7. Li1.55Al0.55Ge0.2Ti1.25(PO4)3 with the AlPO4 impurity phase had similar impedance profiles to those of Li1.50Al0.5Ge0.2Ti1.3(PO4)3 with the AlPO4 impurity phase sintered at 850°C, which revealed a high grain boundary resistance and low bulk conductivity. Figure 7 shows the electrical conductivities of total, grain bulk, grain boundary, and the relative density of Li1+xAlxGe0.2Ti1.8−x(PO4)3 measured at 25°C that are plotted as a function of x. The highest total conductivity of 1.0 × 10−3 S cm−1 and the highest relative density of 95.8% were observed for Li1.45Al0.45Ge0.2Ti1.35(PO4)3 at x = 0.45. The grain bulk conductivities at x = 0.40 and 0.50 are higher than that at x = 0.45. The reason for this tendency is not yet clarified. Aluminum composition in the grain bulk may slightly deviate from the nominal one by its accumulation at the grain boundary region. As another thought, the estimation of the bulk conductivity could be influenced by a parallel grain boundary conduction path as illustrated in the equivalent circuit in Figure 6, although the grain boundary conductivities of Li1+xAlxGe0.2Ti1.8−x(PO4)3 at x = 0.40 and 0.50 were lower than that for Li1.45Al0.45Ge0.2Ti1.35(PO4)3. As a rough tendency, it is possible to state that conductivity becomes maximum around x = 0.45, and as leaving from the composition, the bulk and the grain boundary conductivity decreases. Figure 8 shows the temperature dependence of the total electrical conductivity for Li1.45Al0.45Ge0.2Ti1.35(PO4)3. The activation energy for the electrical conduction was calculated to be 31 kJ mole−1, which is comparable to that for Li1.4Al0.4Ge0.2Ti1.4(PO4)3, as reported previously (Zhang et al., 2013). Figure 9 shows the dependence of the three-point bending strength on the Al content for Li1+xAlxGe0.2Ti1.8−x(PO4)3 sintered at 900°C for 7 h. The maximum bending strength of 90 N mm−2 was observed for Li1.45Al0.45Ge0.2Ti1.35(PO4)3 with a relative density of 95.8%. The bending strength is higher than that of 65 N mm−2 for Li1.4Al0.4Ge0.2Ti1.4(PO4)3 prepared by tape casting using powder prepared by the sol–gel method (Zhang et al., 2015) and lower than that of 140 N mm−2 for a polished Ohara plate of Li1+x+yAlx(Ti,Ge)SiyP3−yO12 glass–ceramics, the conductivity of which is as low as 1 × 10−4 S cm−1 at room temperature. The high bending strength of Li1.45Al0.45Ge0.2Ti1.35(PO4)3 prepared by the conventional solid-state reaction is quite attractive for applications, such as the water-stable protective layer in aqueous lithium batteries.
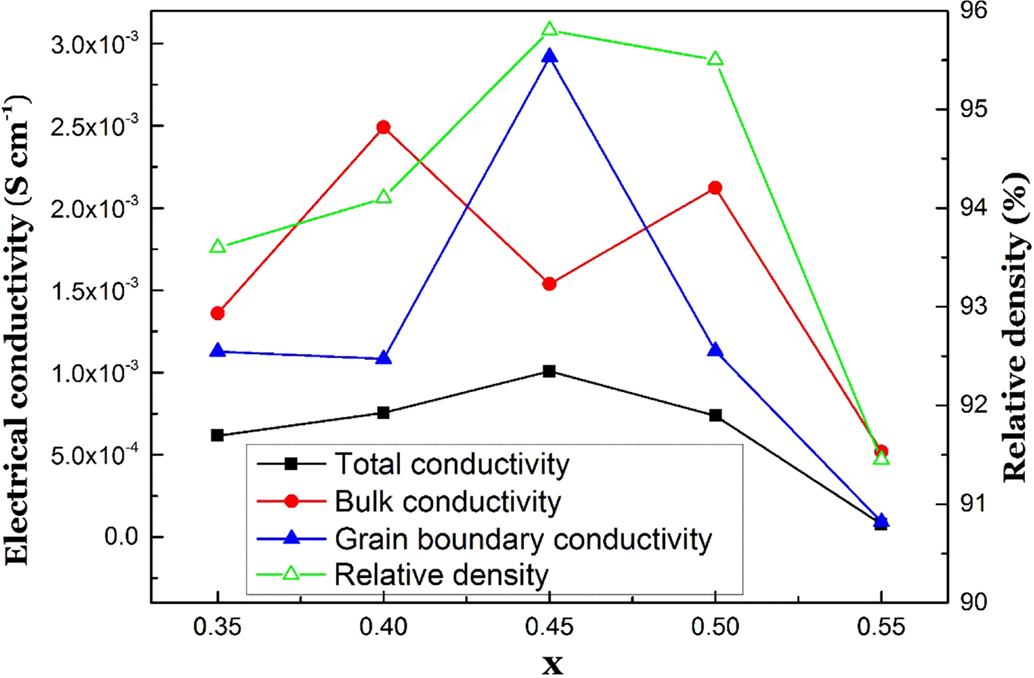
Figure 7. Total, bulk, and grain boundary electrical conductivity at 25°C, and relative density of Li1+xAlxGe0.2Ti1.8−x(PO4)3 as a function of x.
Zhang et al. (2013) examined the electrical conductivity dependence on the Ge content in Li1.4Al0.4GexTi1.6−x(PO4)3, and the highest conductivity was observed for Li1.4Al0.4Ge0.2Ti1.4(PO4)3 prepared by the sol–gel method. We also examined the effect of the Ge content in Li1+xAlxGeyTi2−x−y(PO4)3. Figure 10 shows impedance profiles measured at 25°C of Li1+xAlxGe0.3Ti1.7−x(PO4)3 as a function of x. The highest bulk conductivity of 1.39 × 10−3 S cm−1 and total conductivity of 8.95 × 10−4 S cm−2 were observed for Li1.45Al0.45Ge0.3Ti1.25(PO4)3. The total conductivity and relative density of Li1+xAlxGe0.3Ti1.7−x(PO4)3 are shown as a function of x in Figure 11. The bulk conductivities of Li1.5Al0.5Ge0.3Ti1.2(PO4)3 and Li1.4Al0.4Ge0.3Ti1.3(PO4)3 are considerably lower than those of Li1.5Al0.5Ge0.2Ti1.3(PO4)3 and Li1.4Al0.4Ge0.2Ti1.4(PO4)3, respectively. The highest relative density of 96.3% was observed for Li1.45Al0.45Ge0.3Ti1.25(PO4)3. The Li1.5Al0.5Ge0.1Ti1.4(PO4)3 sample with a low Ge content had a low total conductivity of 2.7 × 10−4 S cm−1 and a low grain boundary conductivity of 3.3 × 10−4 S cm−1 at 25°C.
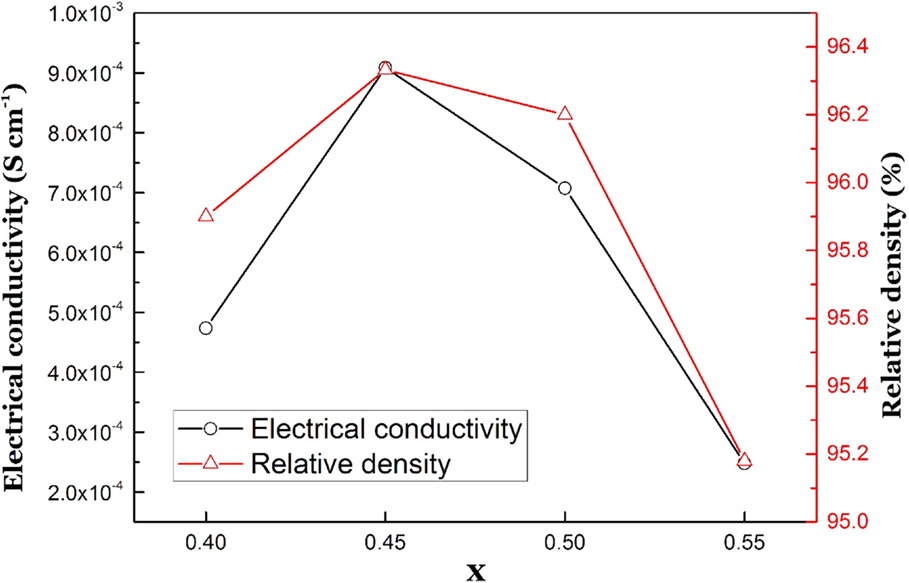
Figure 11. Total electrical conductivity at 25°C and relative density of Li1+xAlxGe0.3Ti1.8−x(PO4)3 as a function of x.
The tape casting method is suitable to prepare solid electrolytes for the large size batteries in electric vehicles and stationary electricity storage systems. Takahashi et al. (2012) reported the electrical conductivity for a tape-cast Li1.4Al0.4Ti1.6(PO4)3–3 wt% TiO2 film as 7.6 × 10−4 S cm−1 at 25°C. Zhang et al. (2015) also reported an electrical conductivity of 1.22 × 10−3 S cm−1 at 25°C for a tape-cast film of Li1.4Al0.4Ge0.2Ti1.4(PO4)3. The powders for these tape casting films were prepared by the sol–gel method using expensive Ti and Ge alkoxides. Here, we prepared Li1.45Al0.45Ge0.2Ti1.35(PO4)3 films by the tape casting method using powders prepared by the conventional solid-state reaction using TiO2 and GeO2. The impedance profile of the film measured at 25°C is shown in Figure 12. Zhang et al. (2015) reported an aging effect on the electrical conductivity of a tape-cast film stored in an air atmosphere. Therefore, the impedance was measured for a film stored for 1 week in an Ar glove box. The total and bulk conductivities at 25°C were estimated to be 1.01 × 10−3 and 2.21 × 10−3 S cm−1, respectively, which are comparable to those of a sintered plate prepared from a pressed green body.
Conclusion
The NASICON-type water-stable lithium-ion-conducting solid electrolyte of Li1+xAlxGeyTi2−x−y(PO4)3 was prepared using conventional solid-state reaction at 900°C for 7 h. The highest lithium-ion conductivity of 10−3 S cm−1 at 25°C was found for the Li1+xAlxGeyTi2−x−y(PO4)3 composition with x = 0.45 and y = 0.2. The relative density of the sintered pellets was as high as 95.8%, and the three-point bending strength was 90 N mm−2. Tape cast films of Li1.45Al0.45Ge0.2Ti1.35(PO4)3 were prepared using powder prepared by solid-state reaction. The total and bulk electrical conductivities of the film were comparable with those of a sintered plate prepared from a pressed green body. This water-stable high lithium-ion-conducting solid electrolyte has potential application as the protective layer of lithium metal electrodes in aqueous lithium–air batteries and lithium batteries with aqueous liquid cathodes.
Author Contributions
SX, HN, and PX did preparation of NASICON-type oxide, characterization, and conductivity measurements. SX also did the tape casting of the ceramics. SM, MM, YT, and OY supported and gave an advice on each experimental work. NI managed the research direction and provided necessary instructions and opportunities for discussions among all authors.
Conflict of Interest Statement
The authors declare that the research was conducted in the absence of any commercial or financial relationships that could be construed as a potential conflict of interest.
References
Aono, H., Sugimoto, E., Sadaoka, Y., Imanaka, S., and Adachi, G. (1990). Ionic conductivity of solid electrolyte based on lithium titanium phosphate. J. Electrochem. Soc. 137, 1023–1027. doi: 10.1149/1.2086597
Alpen, U. V., Rubenau, A., and Talt, G. H. (1977). Ionic conducting in Li3N single crystal. Appl. Phys. Lett. 30, 621–623. doi:10.1063/1.89283
Bouchet, R., Knauth, P., and Laugler, J. M. (2003). Theoretical analysis of IS of polycrystalline materials with blocking or conducting grain boundary: from microcrystals to nanocrystals. J. Electrochem. Soc. 150, E348–E354. doi:10.149/1.1580151
Bruce, P. G., Feunberger, S. A., Hardwick, L. J., and Tarascon, J. M. (2012). Li-O2 and Li-S batteries with high energy storage. Nat. Mater. 11, 19–29. doi:10.1038/nmat3191
Bruce, P. G., and West, A. R. (1983). The A-C conductivity of polycrystalline LISICON Li2+2xZn1+xGeO4 and a model for the intergranular construction resistance. J. Electrochem. Soc. 130, 662–669. doi:10.1149/1.2119778
Cretin, M., and Fabry, P. (1999). Comparative study of lithium ion conductors in the system Li1+xAlxA2-xIV(PO4)3 with AIV=Ti or Ge and 0≤x≤0.7 for use as Li+ sensitive membranes. J. Eur. Ceram. Soc. 19, 2931–2940. doi:10.1016/s0955-2219(99)00055-2
Delmas, C., Viala, J. C., Olazcuage, R., Le Flem, G., Hagnemuller, P., and Chrkaoui, F. (1981). Conductivite ionique dnas les systmes Na1+xZr2+xLx(PO4)3(L=Cr, Yb). Mater. Res. Bull. 16, 83–90. doi:10.1016/0025-5408(81)90182-3
Fu, J. (1997). Fast Li+ ion conducting glass-ceramics in the system Li2O-Al2O3-GeO2-P2O5. Solid State Ionics. 104, 191–194. doi:10.1016/s0167-2738(99)00434-7
Inaguma, N., Chen, L., Itoh, M., Nakamura, T., Uchida, T., Ikuta, H., et al. (1993). High ionic conductivity in lithium lanthanum titanate. Solid State Commun. 86, 689–693. doi:10.1016/0038-1098(93)90841-A
Kamaya, N., Honma, K., Yamakawa, Y., Nakamura, T., Hirayama, M., Kanno, R., et al. (2011). A lithium super ionic conductor. Nat. Mater. 10, 682–686. doi:10.1038/nmat.3066
Lu, Y., Goodenough, J. R., and Kim, Y. (2011). Aqueous cathode for next-generation alkali-ion batteries. J. Am. Chem. Soc. 133, 5756–5759. doi:10.1021/ja.201118f
Maldonado-Manso, P., Aranda, M. A. G., Bruque, J. S., and Losilla, E. R. (2005). Nominal vs. actual stoichiometries in Al-doped NASICONs; a study of the Na1.4Al0.4M1.6(PO4)3 (M=Ge, Sn, Ti, Hf, Zr) family. Solid State Ionics 176, 1613–1625. doi:10.1016/jss.2005.04.009
Murugan, R., Thangadurai, V., and Weppner, W. (2007). Fast lithium ion conduction in garnet-type Li7La3Zr2O12. Angew. Chem. Int. Ed. 46, 7778–7781. doi:10.1002/anie.200701144
Perez-Estebanez, M., Lasai-Marin, J., Tobbens, D. M., Rivera-Calzada, A., and Leon, C. (2014). A systematic study on NASICON-type Li1+xMxTi2-x(PO4)3 (M=Cr, Al, Fe,) by neutron diffraction and impedance spectroscopy. Solid State Ionics 266, 1–8. doi:10.1016/j.ssi.2014.07.018
Shimonishi, Y., Zhang, T., Imanishi, N., Im, D., Lee, D. I., Hirano, A., et al. (2011). A study on lithium/air second batteries – stability of the NASICON-type lithium ion conducting solid electrolyte in alkaline aqueous solution. J. Power Sources 196, 5128–4133. doi:10.1016/jpowersouce.2011.02.023
Takahashi, K., Johnson, P., Imanishi, N., Sammes, N., Takeda, Y., and Yamamoto, O. (2012). A water-stable high lithium ion conducting Li1.4Ti1.6 Al0.4(PO4)3-epoxy resin hybrid sheet. J. Electrochem. Soc. 159, A1065–A1069. doi:10.1149/2.072207jes
Thokchom, J. S., and Kumar, B. (2010). The effects of crystallization parameters on the ionic conductivity of a lithium aluminum germanium phosphate glass ceramics. J. Power Sources 195, 2870–2879. doi:10.1018/j.powersouces.2009.11.037
Wada, H., Menetrierm, A., Lavasseurs, A., and Hagenmuller, P. (1983). Preparation and ionic conductivity of new B2S3-Li2S-LiI glass. Mater. Res. Bull. 18, 189–192. doi:10.1018/0025-5408(83)90080-6
Xu, X., Wen, Z., Wu, X., Yang, X., and Gu, Z. (2007). Lithium ion-conducting glass-ceramics of Li1.5Al0.5Ge1.5(PO4)3-xLi2O (x=0.0-0.20) with good electrical and electrochemical properties. J. Am. Ceram. Soc. 90, 2802–2806. doi:10.1111/j.1511-2916.207.01827.x
Zhang, P., Matsui, M., Hirano, A., Takeda, Y., Yamamoto, O., and Imanishi, N. (2013). Water-stable lithium ion conducting solid electrolyte of the Li1.4Al0.4Ti1.6-xGex(PO4)3 system (A=x=0-1.0) with NASICON-type structure. Solid State Ionics 253, 175–180. doi:10.1016/j.ssi.2013.09.022
Zhang, P., Wang, H., Lee, Y.-G., Matsui, M., Takeda, Y., Yamamoto, O., et al. (2015). Tape-cast water-stable NASICON-type high lithium ion conducting solid electrolyte films for aqueous lithium-air batteries. J. Electrochem. Soc. 162, A1265–A1271. doi:10.1149/2.0711507jes
Zhang, T., Imanishi, N., Shimonishi, Y., Hirano, A., Takeda, Y., Yamamoto, O., et al. (2010). A novel high energy rechargeable lithium/air battery. Chem. Commun. 46, 1661–1663. doi:10.1039/b926012f
Keywords: electrical conductivity, lithium–air battery, lithium conductor, NASICON-type, solid electrolyte
Citation: Xuefu S, Nemori H, Mitsuoka S, Xu P, Matsui M, Takeda Y, Yamamoto O and Imanishi N (2016) High Lithium-Ion-Conducting NASICON-Type Li1+xAlxGeyTi2−x−y(PO4)3 Solid Electrolyte. Front. Energy Res. 4:12. doi: 10.3389/fenrg.2016.00012
Received: 01 February 2016; Accepted: 21 March 2016;
Published: 07 April 2016
Edited by:
Fuminori Mizuno, Toyota Research Institute of North America, USAReviewed by:
Hailei Zhao, University of Science and Technology Beijing, ChinaWenping Sun, University of Wollongong, Australia
Copyright: © 2016 Xuefu, Nemori, Mitsuoka, Xu, Matsui, Takeda, Yamamoto and Imanishi. This is an open-access article distributed under the terms of the Creative Commons Attribution License (CC BY). The use, distribution or reproduction in other forums is permitted, provided the original author(s) or licensor are credited and that the original publication in this journal is cited, in accordance with accepted academic practice. No use, distribution or reproduction is permitted which does not comply with these terms.
*Correspondence: Nobuyuki Imanishi, imanishi@chem.mie-u.ac.jp