- 1School of Environmental Science and Engineering, Gwangju Institute of Science and Technology (GIST), Gwangju, South Korea
- 2Department of Environmental Engineering, National Chung Hsing University, Taichung City, China
- 3Department of Earth and Environmental Engineering, Lenfest Center for Sustainable Energy, Columbia University, New York, NY, USA
- 4Department of Chemical Engineering, Lenfest Center for Sustainable Energy, Columbia University, New York, NY, USA
- 5Department of Chemical Engineering, Imperial College London, London, UK
CO2 capture by amine scrubbing, which has a high CO2 capture capacity and a rapid reaction rate, is the most employed and investigated approach to date. There are a number of recent large-scale demonstrations including the Boundary Dam Carbon Capture Project by SaskPower in Canada that have reported successful implementations of aqueous amine solvent in CO2 capture from flue gases. The findings from these demonstrations will significantly advance the field of CO2 capture in the coming years. While the latest efforts in aqueous amine solvents are exciting and promising, there are still several drawbacks to amine-based CO2 capture solvents including high volatility and corrosiveness of the amine solutions as well as the high parasitic energy penalty during the solvent regeneration step. Thus, in a parallel effort, alternative CO2 capture solvents, which are often anhydrous, have been developed as the third-generation CO2 capture solvents. These novel classes of liquid materials include ionic liquids, CO2-triggered switchable solvents (i.e., CO2-binding organic liquids, reversible ionic liquids), and nanoparticle organic hybrid materials. This paper provides a review of these various anhydrous solvents and their potential for CO2 capture. Particular attention is given to the mechanisms of CO2 absorption in these solvents, their regeneration and their processability – especially taking into account their viscosity. While not intended to provide a complete coverage of the existing literature, this review aims at pointing the major findings reported for these new classes of CO2 capture media.
Introduction
Mitigation of anthropogenic CO2 has become a global challenge to prevent the negative consequences associated with high atmospheric CO2 concentration. One may capture CO2 either from large point sources, such as power plants, or directly from the atmosphere (Keith, 2009; Orr, 2009). In this review paper, the focus will be on technologies used to capture CO2 from flue gas streams, particularly via a post-combustion capture process. Various technologies that have been developed for post-combustion CO2 capture include chemical absorption, physical and chemical adsorption, and membrane separation (MacDowell et al., 2010; Boot-Handford et al., 2014).
Amine scrubbing using aqueous solutions of monoethanolamine (MEA), diethanolamine (DEA), and methyldiethanolamine (MDEA), represents the most mature technology for post-combustion capture. It relies on chemical absorption and more specifically on the chemical reaction between CO2 and the amine group of the solvent (Rochelle, 2009). Amine-based reagents exhibit favorable thermodynamic and kinetic properties for CO2 absorption and are considered affordable since the solvents are already commercially available. Nevertheless, these materials are corrosive and tend to degrade during their regeneration, which is typically performed via heating (MacDowell et al., 2010; Boot-Handford et al., 2014). Moreover, during the thermal regeneration of the materials, not only is the solvent heated but also the water. The process is, therefore, impaired by a high parasitic energy penalty since water represents 70–85 wt% of the amine reagent. For all these reasons, alternative CO2 capture solvents that are environmentally benign, thermally and chemically stable, and that exhibit high absorption capacity and fast kinetics, are coveted for effective CO2 capture, from both process and cost points of view.
A number of alternative materials have, therefore, been proposed to substitute for the amine-based solvents including solid sorbents, advanced membranes, and novel liquid solvents (MacDowell et al., 2010; Boot-Handford et al., 2014). In particular, the recent investigation of novel anhydrous liquid materials, such as ionic liquids (ILs), CO2-triggered switchable absorbents [i.e., CO2-binding organic liquids (CO2BOLs)], and nanoparticle organic hybrid materials (NOHMs), provide an interesting selection of alternative solvents for CO2 capture. Indeed, owing to their anhydrous nature, the energy required to recycle them is overall diminished compared to aqueous amine-based solvents. In addition, these absorbents could be directly used in the same type of reactor as that designed for amine-based solvents. This review paper provides an overview of the most recent advances in the field of anhydrous liquid materials for CO2 capture. Each section of the paper is dedicated to a specific type of absorbent, i.e., ILs, CO2-triggered switchable absorbents and NOHMs. This paper does not provide an exhaustive review of all papers on the topic, but rather aims at presenting the major and most recent findings for each type of absorbent. In the case of ILs, the reader is invited to refer to these comprehensive reviews: Ramdin et al. (2012), Zhang et al. (2012), and Lei et al. (2014). The three classes of solvents, i.e., ILs, CO2-triggered switchable absorbents, and NOHMs – are discussed below starting from the most mature technologies to the most recently developed absorbents. Overall, the materials presented here are still being investigated at the lab-scale and could represent the next generation of CO2 capture materials.
Liquids (ILs)
Ionic liquids are composed solely of cations and anions (no additional solvent) and typically melt below 100°C. As such, ILs differ from typical molten salts, which remain solid even at hundreds of degrees Celsius. The asymmetry of the cations accounts for the low melting points of ILs and the anions are responsible for ILs’ physical properties (Dzyuba and Bartsch, 2002). Various combinations of cations and anions have been selected to synthesize a wide range of ILs – the number of potential ILs is on the order of 1018. Owing to the tunability of their physicochemical properties and structures, ILs can be employed in a wide variety of environmental and energy-related applications (Koel, 2009; MacFarlane et al., 2014). Typical examples of ILs employed for CO2 capture consist of nitrogen- or phosphorous-containing organic cations. Examples of ILs are shown in Scheme 1. Besides negligible vapor pressure (~10 N m−2), ILs also exhibit high thermal stability over a wide temperature range, up to 300°C (Freemantle, 2010). Owing to these merits, ILs have been proposed for CO2 capture. ILs for CO2 capture can be classified into three major categories based on their CO2 capture mechanisms: (i) conventional ILs, (ii) task-specific ILs (TSILs), and (iii) reversible ILs (RevILs). All these categories are described below. It must be noted that RevILs are discussed in the section dedicated to CO2-triggered switchable solvents.
Conventional Ionic Liquids
Conventional ILs are characterized by the absence of any task-specific functional groups, i.e., groups that can chemically react with CO2, such as amines. Despite this feature, conventional ILs exhibit promising CO2 capture capacity and selectivity. In fact, a key strength of these ILs is their facile regeneration via a mild treatment (e.g., low temperature heating 70−80°C, inert gas bubbling or pressure swing). Indeed, unlike amine-based solvents, which utilize strong chemical interactions between amine and CO2 (−ΔH = 80–130 kJ mol−1), conventional ILs interact with CO2 via relatively weak forces (−ΔH = ~15 kJ mol−1) (Ramdin et al., 2012). A detailed study of solubilities of various gases, including water vapor, Ar, CO, CO2, CH4, C2H4, C2H6, H2, N2, and O2 in [bmim][PF6], was conducted by Anthony et al. (2002). Besides water vapor, CO2 exhibited the highest solubility in [bmim][PF6], followed by CH4, C2H4, and C2H6. It was indicated that the large quadrupole moment of CO2 makes it highly soluble in [bmim][PF6]. Anderson et al. (2007) also found that even common ILs, such as [hmmim][Tf2N], have excellent selectivity for CO2 relative to N2 and other components in flue gas streams based on physical absorption. Blanchard et al. (2001) determined the solubility of CO2 in a series of imidazolium-based ILs in the pressure region of 1–100 bar, and the solubilities followed this order: [bmim][PF6] > [omim][PF6] > [omim][BF4] > [N-bupy][BF4] > [bmim][NO3] > [emim][EtSO4]. Interestingly, imidazolium-based ILs exhibited remarkable CO2 capture capacity (Anthony et al., 2002; Kamps et al., 2003; Husson et al., 2010). Semi-empirical studies were also conducted to determine the Henry constant of several ILs (Mortazavi-Manesh et al., 2011; Sistla and Khanna, 2011). Overall, it was found that the shape and size of ILs played a major role in the value of the Henry constant of ILs.
The solvating mechanisms of ILs for CO2 are not fully understood, but several explanations have been put forth. Kazarian et al. (2000) found spectroscopic evidence of a Lewis acid–base intermolecular interaction between anions and CO2 using attenuated total reflectance (ATR) Fourier-transform infrared (FT-IR) spectroscopy, explaining the high CO2 capture capacity in [bmim][PF6]. The anion acted as a basic site for CO2, allowing for weak Lewis acid–base interactions between anion and CO2 to drive the capture of CO2. Cadena et al. investigated the effect of anions in alkylimidazolium-based ILs for CO2 capture via experimental and molecular simulation studies. They revealed that the anions played a critical role in interacting with CO2 (Cadena et al., 2004). Anthony et al. also investigated the effect of anions, focusing on [BF4]−, [PF6]−, and [Tf2N]−, with [bmim]+ as a counterion. They observed that [bmim][Tf2N] exhibited relatively high capacity for CO2, while [bmim][BF4] and [bmim][PF6] had lower affinity for capturing CO2 (Anthony et al., 2005). Among the anions, those with fluoride have drawn significant interest (Aki et al., 2004; Bara et al., 2009; Sistla and Khanna, 2011). For instance, a detailed study on the effect of anions on CO2 capture was conducted focusing on the influence of fluorination on CO2 solubility in ILs (Aki et al., 2004). The fluorinated anions, such as [BF4]−, [PF6]−, [Tf2N]−, [TfO]−, and [methide]−, were compared with non-fluorinated anions like [DCA]− and [NO3]− ([bmim]+ used as a cation). The results indicated that ILs with fluorinated anions captured more CO2. In particular, ILs that had anions with fluoroalkyl groups, such as [TfO]−, [Tf2N]−, and [methide]−, showed the highest CO2 solubilities. The reported order of solubility in different types of anions was: [NO3]− < [DCA]− < [BF4]− ≈ [PF6]− < [TfO]− < [methide]− < [Tf2N]−, where [TfO]−, [methide]−, and [Tf2N]− contain one, two, and three –CF3 groups, respectively. On the other hand, Bara et al. (2009) observed a decrease CO2/N2 selectivity in ILs fluorinated anions compared to their alkyl counterparts. Fluorination of the alkyl chains in cations, as well as anions, has also been investigated to increase CO2 capture capacity (Baltus et al., 2003; Muldoon et al., 2007). A modeling study conducted by Sistla and Khanna (2011) also focused on the effect of fluorination on the Henry’s constant of several ILs. In addition, the effects of anions with sulfate and phosphonium groups on CO2 solubility were investigated. These ILs exhibited comparable CO2 capture capacity to imidazolium-based ILs (Zhang et al., 2005; Ferguson and Scovazzo, 2007; Carvalho et al., 2010; Harper et al., 2011).
Although many studies pointed to the importance of the anion effect on CO2 solubility, the cation effect has also been investigated (Blanchard et al., 2001; Aki et al., 2004). Blanchard et al. (2001) selected three ILs with different imidazolium-based cations [i.e., [hmim]+, [omim]+, and [bmim]+] and [Tf2N]− as an anion to investigate the cation effect on CO2 solubility. It was found that longer alkyl chains noticeably increased CO2 solubility. Hojniak et al. (2013) used dicationic supported ILs membranes based on imidazolium, pyrrolidinium, piperidinium, and morpholinium ions. They showed that increasing the cationic charge led to an enhancement of selectivity toward CO2 compared to N2. Another mechanism proposed to explain CO2 solubility in ILs is the presence of a free volume that can accommodate CO2 molecules. There is, however, no agreement on the location of this free volume as two theories have been proposed: (i) on the one hand, it is thought that the free volume is near the alkyl chain of the cation and (ii) on the other hand, some studies showed that the free volume could be located in the space between the cation and anion (Babarao et al., 2011; Zhang et al., 2011; Lei et al., 2014). Despite the uncertainty, the free volume is considered to play a significant role in CO2 solubility. Further in-depth investigations on the nature of free volume are required, particularly focusing on the relationship between total pressure and molecular structure.
The cation and anion effects as well as the presence of a free volume are the most common drivers for CO2 capture in conventional ILs. In addition to these, other parameters have been investigated to enhance CO2 solubility. One of them is the introduction of ether groups, which has a dual purpose: (i) rendering ILs more flexible, thereby increasing free volume (Sarbu et al., 2000; Beckman, 2004) and (ii) enabling dipole–quadrupole interactions between the oxygen atoms of the ether groups and CO2 (Ghosal et al., 1996; Van Ginderen et al., 2003). An example of IL with ether groups is [N4444][doc] for which a high CO2 solubility was measured (Muldoon et al., 2007). The carbonyl groups and long branched alkyl groups in [N4444][doc] were also thought to contribute to its high CO2 solubility, which was even higher than fluorinated ILs at high partial pressure of CO2. However, the extremely high viscosity of [N4444][doc] (12,100 cP at 25°C) is one of the challenges for its practical application. The positive impact of the presence of ether groups on CO2 solubility was also observed by Makino et al. (2014) who used ammonium bis(trifluoromethanesulfonyl)amide IL systems to capture CO2. On the other hand, Bara et al. showed that imidazolium-based ILs with one to three oligo(ethylene glycol) substituents absorbed the same amount of CO2 compared to their alkyl-substituted counterparts. However, their study pointed to a higher selectivity toward CO2 compared to N2 and CH4 for ILs with ether groups (Bara et al., 2007).
The effect of other functional groups, such as carbonyl and ester, on CO2 solubility in ILs has also been investigated. For instance, [b2Nic][Tf2N], a pyridinium-based IL with a butyl ester group, was studied by Muldoon et al. (2007) to analyze the effect of ester groups on CO2 solubility. Results indicated that [b2Nic][Tf2N] had a noticeably higher CO2 solubility than [hmim][Tf2N] under high partial pressure of CO2, which was the result of minor interactions between CO2 and ester groups. This was contrary to the finding of Makino et al. (2014) whose study on ammonium bis(trifluoromethanesulfonyl)amide ILs showed that the presence of ester groups led to a decrease in CO2 solubility.
Overall, conventional ILs exhibit a very CO2 capture capacity. Typically, at pressures between 9 and 30 bar at room temperature, capacities between 0.1 and 0.6 mol CO2/IL (or 3 and 16 wt%) have been reported for various conventional ILs (Zhang et al., 2012). This has to be compared to 0.3 mol CO2/MEA (or 7 wt%) at 1 bar and 25°C for 30 wt% MEA solvent. In addition, the absence of any functional groups can restrict the selectivity of the materials. These aspects have prompted the development of another class of ILs referred to as task-specific ionic liquids (TSILs). These ILs are characterized by their amine or other functionalities that allow for relatively strong interactions with CO2. This type of ILs is discussed in the next section.
Task-Specific Ionic Liquids
Task-specific ionic liquids are mostly similar to conventional ILs expect that they exhibit functional groups, which can capture CO2 more rapidly. In most cases, the functional groups in question are amine groups but more recent studies have looked into other functionalities as well, which are discussed below. Regeneration of TSILs requires slightly more stringent conditions than conventional ILs, such as higher temperature or longer heat treatment in vacuum. In general, these treatments enable the complete recycling of the ILs with no or minimal loss of capture capacity and material. This is due to the chemical interactions involved in the capture process. Interestingly though, a recent study showed that by properly selecting the structure of TSILs, and particularly the position of the amine group on the benzene-derived anion (i.e., para, meta, or ortho), one could tune the binding energy of CO2 and hence the regeneration requirements (Luo et al., 2014b). Bates et al. (2002) synthesized the first type of TSILs, [pabim][BF4], shown in Scheme 1A. They successfully introduced the primary amines on cations, and it allowed for the formation of an ammonium carbamate salt, which reacted with CO2 in the same way as in a conventional amine scrubbing process (Scheme 2). The molar equilibrium ratio of CO2 to [pabim][BF4] was 1:2 and the weight gain due to CO2 capture was 7.4%, compared to 0.1% in [hmim][PF6], a conventional IL. The findings pointed to the enhancement of CO2 solubility at low pressure owing to the presence of the amine groups, while physical sorption occurred at high pressure.
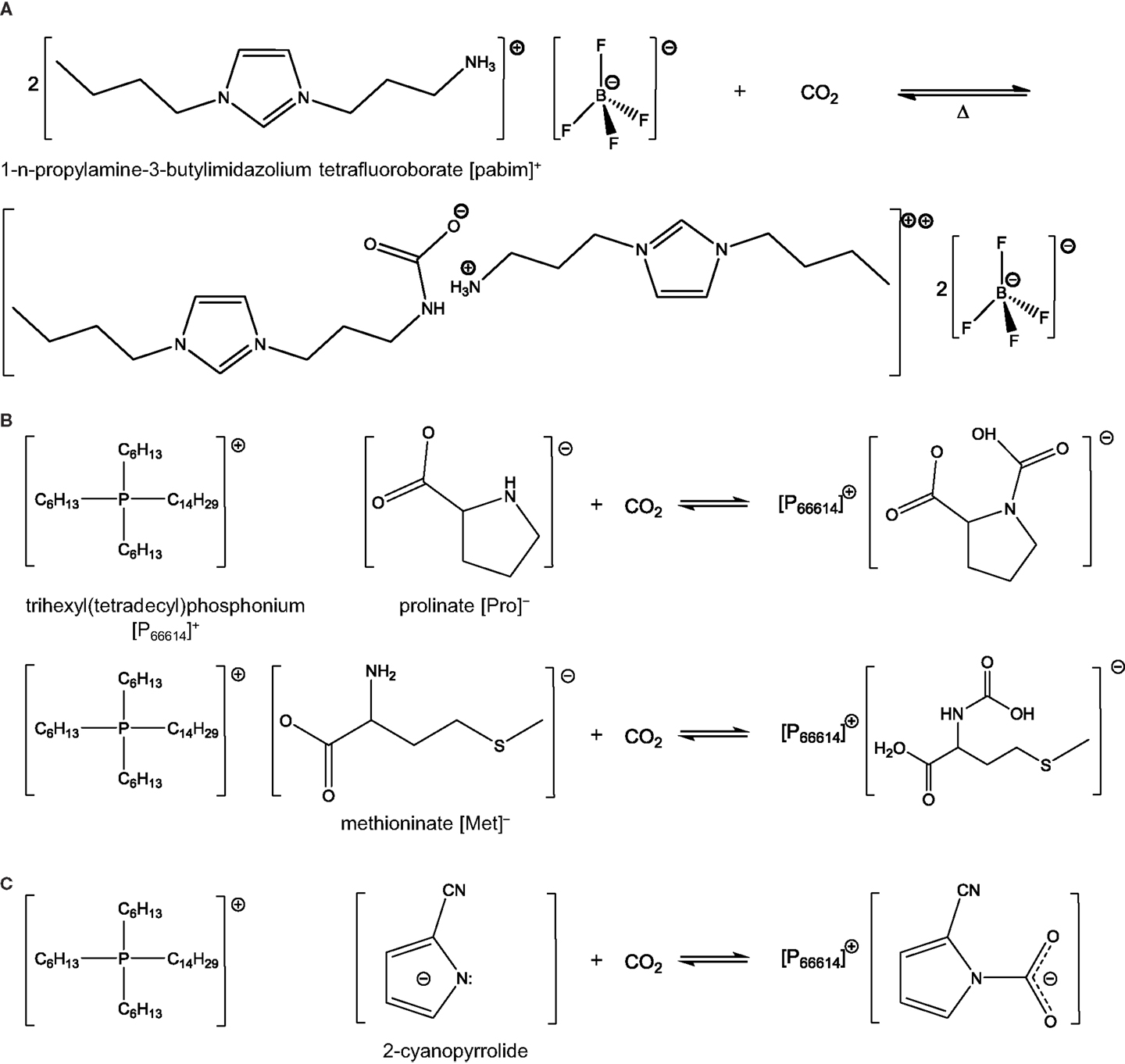
Scheme 2. Reaction between CO2 and TSILs: (A) ratio 1:2 with [pabim][BF4], (B) ratio 1:1 with [P66614][Pro] and [P66614][Met] [adapted from Gurkan et al. (2010b) and Heldebrant (2015)], and (C) AHA ILs reacting with CO2.
The first examples of TSILs all led to a CO2 to ILs molar ratio of 1:2 as can be deduced from Scheme 2A. Later, Gurkan et al. reported the preparation of new amine-based TSILs resulting in an equimolar ratio between the two entities (Gurkan et al., 2010b). Such a behavior significantly enhanced the capacity of ILs. The way to reach such high solubility was to introduce the amine functionality on the anionic component and not on the cationic one as in “regular” TSILs. Specifically, the ILs used by Gurkan et al. consisted of [P66614]+ and [Met]− or [Pro]− (Scheme 2B). Upon exposure to CO2, the amino group of the ILs reacted with CO2 and formed carbamic acid. More recently, a CO2 to ILs molar ratio of 1.6:1 was reported by Luo et al. at 1 bar and 20°C (Luo et al., 2014a). The researchers used a pyridium-based anion functionalized with a hydroxide group. Both functionalities were hypothesized to react with CO2 in a cooperative way. The cation used was [P66614]+.
Besides amines, nitrile functional groups have also been trialed as a way to improve the CO2 selectivity and permeance in ILs. Mahurin et al. (2010) prepared a series of nitrile-containing ILs for supported IL membranes. These nitrile-containing ILs exhibited high CO2 solubilities and selectivities over N2. Among them, 1-ethyl-3-methylimidazolium tetracyanoborate [emim][B(CN)4], exhibited the highest CO2 solubility as well as permeance. The positive impact of nitrile groups was further confirmed by a modeling study by Gupta and Jiang (2014), which indicated that increasing the number of nitrile groups led to an increased CO2 solubility. Among the various ILs tested, [bmim][B(CN)4] was identified as the most promising one. It was suggested that the presence of the nitrile group weakens the cation–anion interactions and this was correlated with an increase in CO2 solubility. Carlisle et al. (2008) showed that nitrile-functionalized ILs exhibited enhanced CO2/CH4 and CO2/N2 solubility selectivity compared to their alkyl-substituted counterparts.
Wang et al. (2011) studied the solubility of CO2 in a triethylbutylammonium acetate TSIL ([N2224][CH3COO]) and its derived hydrated complexes. While in the dried TSIL the capture mechanism mainly involved Lewis acid-based interactions, in the case of the hydrated complexes, CO2, H2O, and the acetate component reacted to form the bicarbonate ion and acetic acid. The mole fractions reported at room temperature and a pressure of 1 bar ranged from 0.05 to 0.39.
Although amine-functionalized TSILs have allowed a noticeable improvement in terms of CO2 capture capacities, they suffer from a relatively high viscosity. Furthermore, an increase in viscosity is typically observed in those materials upon reaction with CO2 (Soutullo et al., 2007). A molecular simulation study reported that the high viscosity in neat TSILs (i.e., not yet exposed to CO2) is caused by the strong H-bonding interactions between the anions and the amine groups of the cations (Yu et al., 2007). Another simulation study was conducted by Gutowski and Maginn (2008) to clarify the mechanism causing the dramatic increase in viscosity when TSILs are exposed to CO2. The authors suggested that it was due to the complexation of TSIL and CO2 leading to an extensive H-bonding network, i.e., a salt bridge network connecting carbamate and ammonium species (Gutowski and Maginn, 2008).
Reducing the viscosity of TSILs has become one of the key challenges and many studies have been dedicated to this aspect. A reduction in the extent of this H-bonding network was observed on a representative IL of a new class of TSILs referred to as aprotic heterocyclic anion (AHA) ILs (Gurkan et al., 2010a; Wu et al., 2011; Seo et al., 2014). AHA ILs corresponds to ILs with pyrrolide-based anions (Gurkan et al., 2010a). These anions can react with CO2 to form a carbamate (Scheme 2C). In this reaction, the CO2 to IL ratio is 1. A key advantage of the reaction is that it does not lead to the formation of H-bonds, thereby addressing the viscosity issue. Experimental studies supported by computational measurements confirmed the absence of increase in viscosity in AHA ILs (Gurkan et al., 2010a; Wu et al., 2011). In addition, the CO2 solubilities were in a similar range to that of many other “regular” TSILs (Wu et al., 2011). A recent study conducted on several AHA ILs with varying substituents on the pyrrolide anion evidenced that functionalization of these ILs could be used to tune the binding energy of CO2 (Seo et al., 2014). When the cation in AHA ILs is imidazolium, it was found that the mechanism of CO2 capture was not simply via the CO2-anion reaction. A parallel reaction also occurred in which a proton exchange took place between the anion and the cation leading to the formation of carbene species that could react with CO2 (Seo et al., 2014).
Besides the use of AHA ILs, another way to deal with the viscosity issue is to mix TSILs with a low-viscosity fluid. A range of solvents have been tested including conventional ILs, alcohols (Liu et al., 2003), ketones (Zhang et al., 2007; Lei et al., 2012), organic acids (Bogel-Łukasika et al., 2010), water (Goodrich et al., 2011; Stevanovic et al., 2012) as well as alkanolamines (Camper et al., 2008; Sairi et al., 2011). For instance, Bara et al. (2010) dissolved a TSIL into a conventional RTIL, [hmim][Tf2N], to lower overall viscosity so that the sorbent could be employed in gas separation units. Following the same principle, other studies have looked at mixtures of alkanolamines and RTILs or TSILs to dramatically lower viscosity while increasing CO2 capture capacity (Camper et al., 2008; Sairi et al., 2011). Goodrich et al. (2011) mixed [P66614][Pro] and water and observed a decrease in the overall viscosity of the mixture, while the decrease in CO2 capture capacity was negligible. On the other hand, an enhancement of CO2 capture in ILs in the presence of water was observed by Romanos et al. (2013). This trend was opposite to that observed by Stevanovic et al. (2012) with a different ILs. Overall, these studies show promising potential to integrate ILs and other solvents to address the viscosity issue but the simultaneous increase in CO2 solubility is not systematic and depends on the solvent as well as the IL used.
CO2-Triggered Switchable Solvents
CO2-Binding Organic Liquids
Another class of anhydrous solvents for CO2 capture is that of CO2BOLs. CO2BOLs are organic liquids consisting of an alcohol and a strong amidine or guanidine base that can chemically capture CO2 to form amidinium or guanidinium alkylcarbonate, respectively (Liu et al., 2006; Phan et al., 2008; Heldebrant et al., 2009). The reaction between CO2 and CO2BOLs is shown in Scheme 3. Typically, the CO2 capture capacity of CO2BOLs can be up to about 20 wt% (Heldebrant et al., 2008, 2009). The first generation of CO2BOLs consisted of two distinct molecules, the strong base and the alcohol, and exhibited relatively high volatility. To address this aspect, it was decided to combine the two molecules into one single component forming either alkanolguanidine or alkanolamidine. The reaction of these species with CO2 leads to the formation of zwitterionic species as shown in Scheme 3B (Heldebrant et al., 2010a, 2011; Kim and Park, 2010). While improvement was made on one front, it was found that single-component CO2BOLs were highly viscous when loaded with CO2 (>2,000 cP). As a result, a further advancement was the development of a low-viscosity single-component CO2BOLs (Koech et al., 2013). The best results were obtained with alkanolguanidines with an imidazolidine-based core.
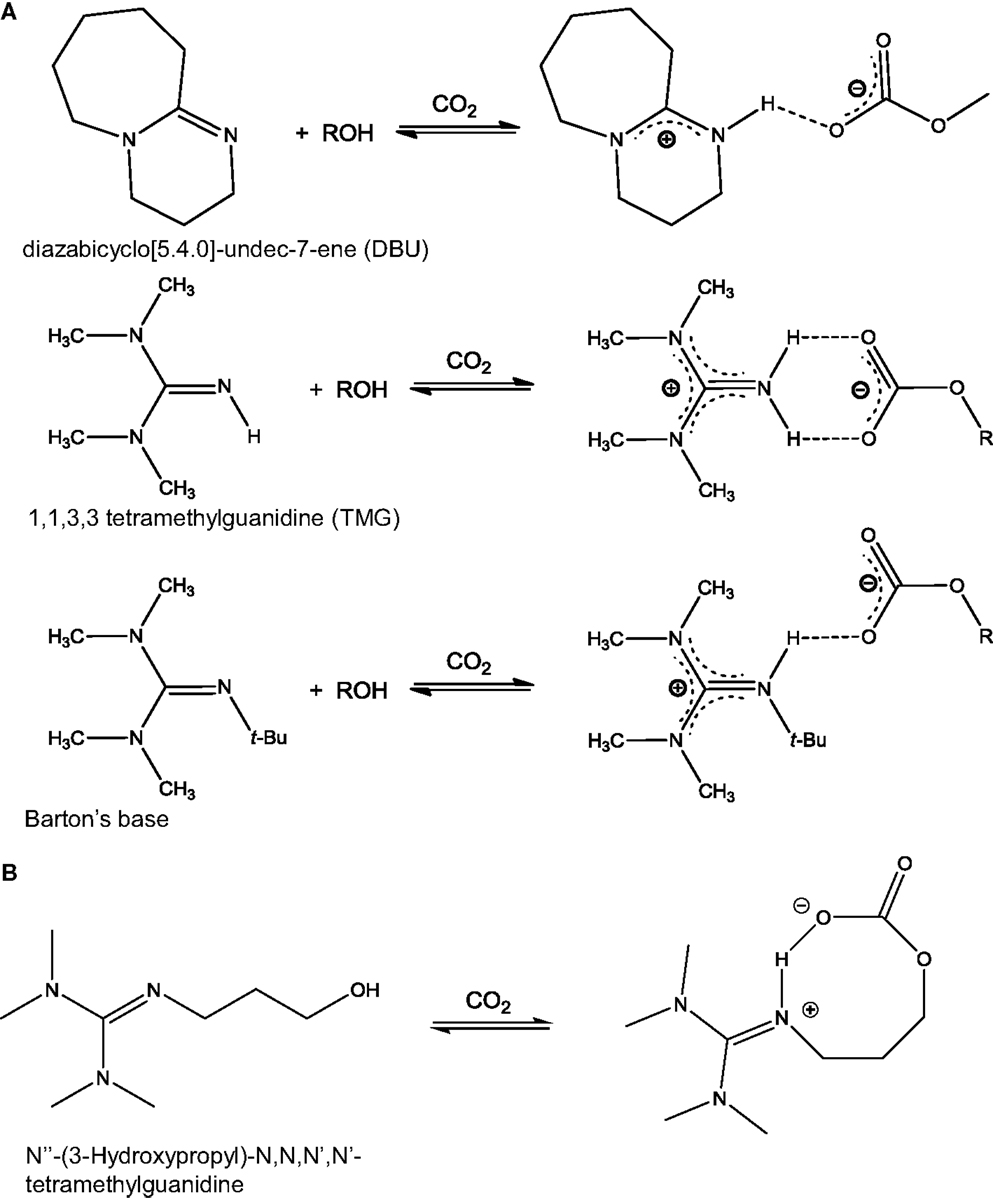
Scheme 3. Reaction between CO2 and CO2BOLs: (A) CO2BOLs composed of two species, an alcohol and an amidine (top) or guanidine based (middle and bottom). Reaction with CO2 leads to the formation of H-bonds [adapted from Heldebrant et al. (2008)]; (B) CO2BOLs composed of a single species. Reaction with CO2 leads to the formation of a zwitterionic species [adapted from Park et al. (2011)].
One of the most interesting features of CO2BOLs is their low binding energy for CO2, which allows them to be regenerated without significant energy consumption. Initially focusing on the first generation of CO2BOLs, Heldebrant et al. (2008) reported the free energies of CO2BOLs for CO2 capture and they found that the binding energies for CO2 in these materials were notably small, ranging from −9 to 2 kJ mol−1 depending on the solvent. Because the CO2-binding energy of CO2BOLs was small, a relatively low temperature swing process could be applied to regenerate the solvents (90°C) over five cycles with no visible loss of activity and selectivity (Heldebrant et al., 2009). It must be noted though that some CO2BOLs required higher temperature for regeneration up to 160°C (Mathias et al., 2013). As the single-component CO2BOLs were developed, the product formed as a result of CO2 capture was polar – zwitterionic species – and therefore the idea of using polarity-swing-assisted regeneration (PSAR) process was investigated to replace the common temperature swing approach (Mathias et al., 2013). The process consisted in adding an inert and non-polar anti-solvent to the CO2BOLs as the way to lower the polarity of the system, thereby releasing CO2. The non-polar solvent could then be separated from CO2BOLS by lowering the temperature to induce a phase separation. Overall, this process called for lower regeneration temperatures (about 85°C) and energies for CO2 separation relative to conventional technology.
Heldebrant et al. (2011) studied the robustness and recyclability of alkanolganidines, alkanolamidines, and mixtures of these two types of CO2BOLs. Regeneration of the materials was tested for 10 cycles via heat treatment at 75°C under nitrogen atmosphere, in dry conditions, or in the presence of 10 mol% water. In both cases, the CO2 uptake was not significantly decreased. This was confirmed by a subsequent study (Koech et al., 2013). It must be noted that BOLs have also been shown to capture SO2 (Heldebrant et al., 2010b).
Reversible Ionic Liquids
Other representative solvents of the switchable solvents family are RevILs (Blasucci et al., 2009, 2010; Blasuccia et al., 2010; Gonzalez-Miquel et al., 2012; Switzer et al., 2013, 2014). RevILs consist of silylated amines that react with CO2 to form an IL. As CO2 reacts with RevILs, an IL is formed, as shown in Scheme 4. This newly formed IL can, in turn, absorb CO2 like a conventional IL. RevILs are distinguished from ILs in that the species are non-ionic prior to reaction with CO2, which makes them theoretically less viscous upon reaction with CO2 compared to TSILs. RevILs also differ from CO2BOLs since CO2 reacts with the N-containing group of RevILs, while it reacts with the alcohol group in the case of CO2BOLs. Nevertheless, it must be noted that some papers would classify single-component CO2BOLs as a sub-category of RevILs. In this review, the two are distinguished.
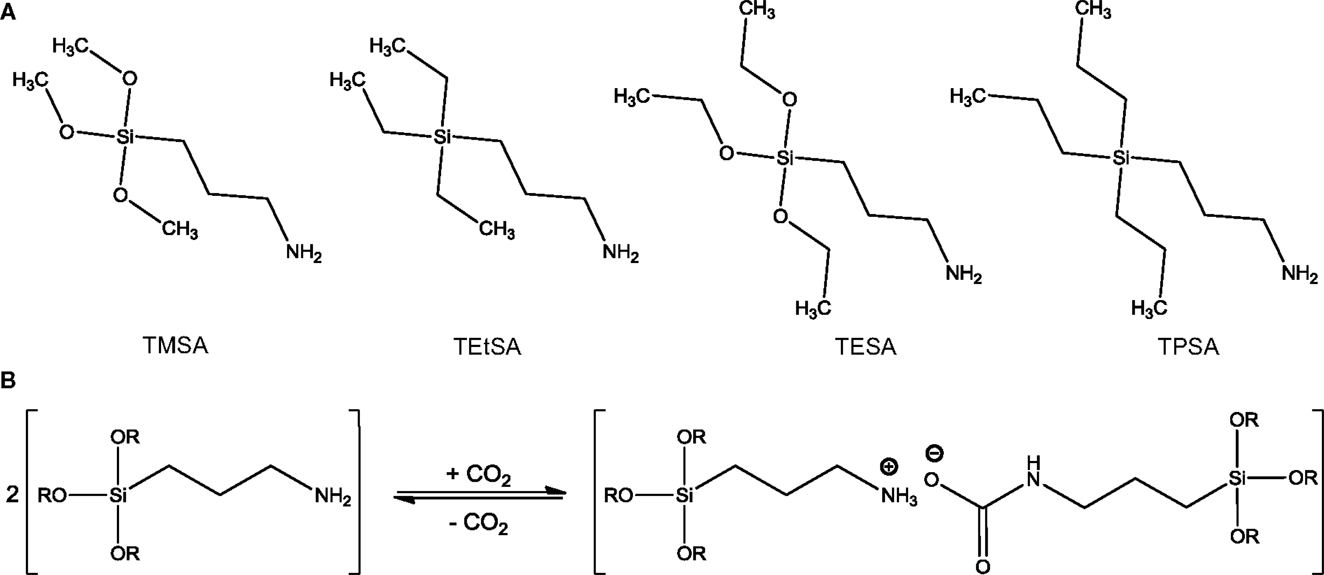
Scheme 4. (A) Examples of structures of RevILs: (3-aminopropyl)-trimethoxysilane (TMSA), (3-aminopropyl)-triethylsilane (TEtSA), (3-aminopropyl)-triethoxysilane (TESA), and (3-aminopropyl)-tripropylsilane (TPSA), and (B) reversible reaction of RevILs with CO2 where R = methyl or ethyl [adapted from Blasucci et al. (2009)].
It was found that the CO2 to amine ratio in RevILs could reach up to 0.7:1 (Switzer et al., 2013, 2014). The structural properties of silylated amines (i.e., length of the carbon chains, branched or unbranched, primary or secondary amines) strongly determine the viscosity (600–4,000 cP) and CO2 solubility of RevILs (Blasuccia et al., 2010; Gonzalez-Miquel et al., 2012; Switzer et al., 2014). RevILs can be easily switched back to molecular liquids after exposure to CO2 by purging with an inert gas to remove CO2, or heating up to 50–100°C, depending on the types of RevILs (Jessop et al., 2005; Blasucci et al., 2010; Blasuccia et al., 2010). Heat of reaction between 20 and 150 kJ/mol has been reported (Switzer et al., 2014).
Nanoparticle Organic Hybrid Materials
The last set of anhydrous solvents for CO2 capture discussed in this review is that of NOHMs. NOHMs are nanomaterials consisting of a nanocore functionalized with polymer chains (canopy). In some cases, a linker (corona) can be used to link the canopy to the core (Bourlinors et al., 2005; Bourlinos et al., 2005a,b, 2006; Rodriguez et al., 2008; Agarwal et al., 2009; Yu and Koch, 2010; Zheng et al., 2010). The canopy can be tethered onto the nanocore either covalently or ionically as shown in Scheme 5A. Various materials have been used as cores including oxide nanoparticles, metallic nanoparticles, carbon nanotubes, and polyoxometalates (Bourlinors et al., 2005; Bourlinos et al., 2005a,b, 2006; Rodriguez et al., 2008; Agarwal et al., 2009; Yu and Koch, 2010; Zheng et al., 2010). The corona is typically a silane or thiol compound, while polyethylene glycol, polyetheramine, and polyethyleneimine are examples of polymers used to form the canopy. Depending on their composition, NOHMs can exhibit liquid-, gel-, or solid-like properties. When applied for CO2 capture, the focus has been on the former type of NOHMs, which corresponds to the category discussed in this review. Like ILs, NOHMs exhibit negligible vapor pressure owing to the bond existing between the canopy (or canopy plus corona) and the thermostable nanocore. The latter feature also confers NOHMs with a good thermal stability up to about 300°C, which is always higher than the parent polymer (Lin et al., 2014). Currently, NOHMs are classified based on their representative phase and synthesis methods as well as the existence of task-specific functional groups (see Scheme 5B) (Lin and Park, 2011).
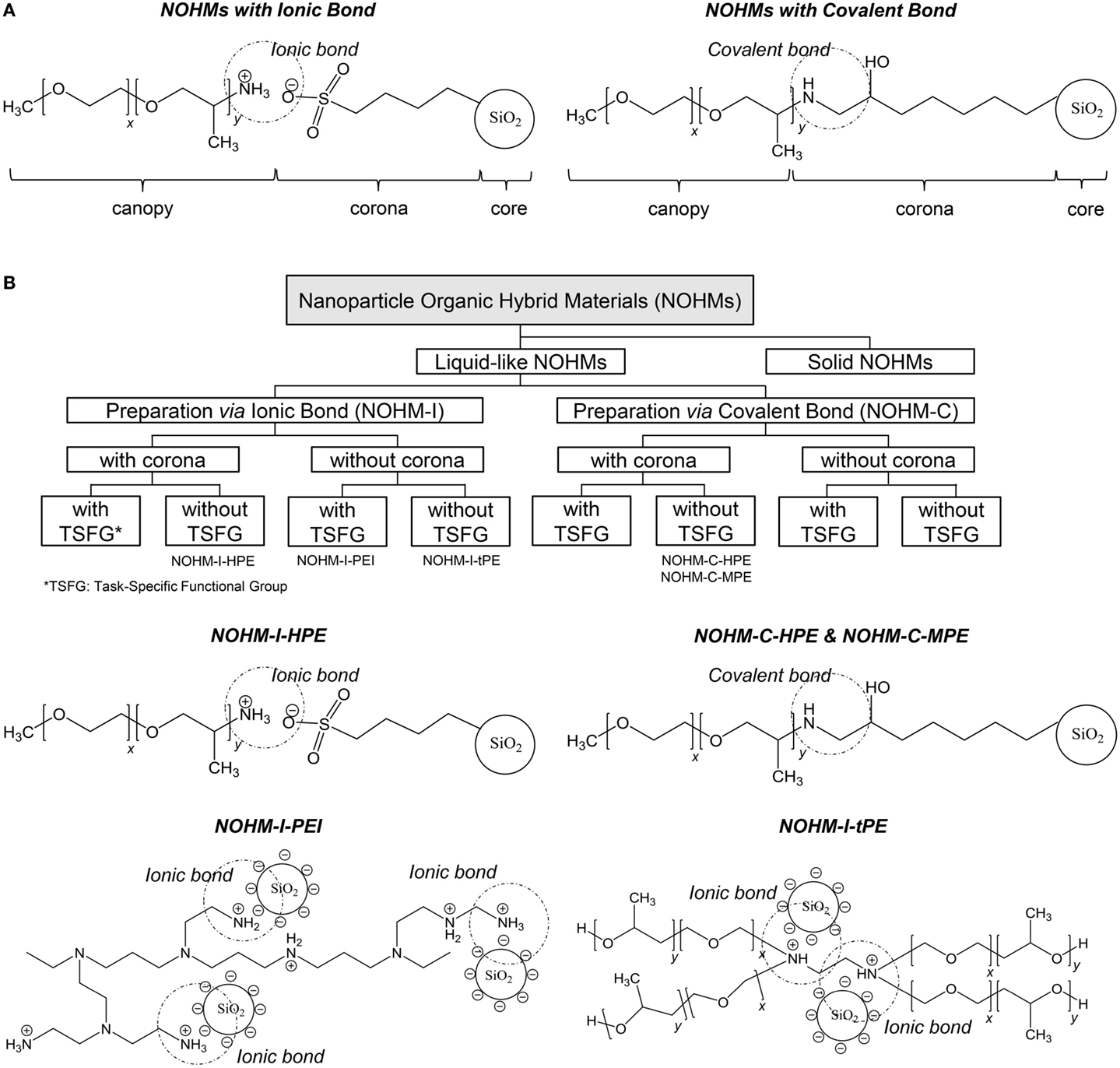
Scheme 5. NOHMs: (A) overview of their structure with either ionic or covalent bonding between the canopy and the functionalized core; (B) overview of the classification of NOHMs and examples of NOHMs: NOHM-I-HPE (corona = sulfonic acid, polymer chain = polyetheramine); NOHM-I-PEI (no corona, polymer chain = polyethylenimine); NOHM-I-tPE (no corona, polymer chain = tertiary amine polyether); and NOHM-C-HPE and NOHM-C-MPE (corona = glycidyl ether, polymer chain = polyetheramine) [adapted from Lin and Park (2011)].
It was found that CO2 capture in NOHMs arises from two effects: an entropic effect and an enthalpic effect. The former contribution is due to the conformation of the polymer chains in NOHMs, which are better aligned and ordered than in pure polymers as they have to “fill out the gaps” between the nanocores (Park et al., 2011; Petit et al., 2012). Capture of CO2 in NOHMs is, therefore, favored as it allows the solvents to return to a more relaxed state. This entropic effect can be tuned by changing the shape of the canopy – i.e., linear versus branched polymer – as well as the canopy grafting density – i.e., the number of polymer chain tethered onto the nanocore (Petit et al., 2012; Park et al., 2014). Nevertheless, the entropic contribution toward CO2 capture remains minimal. On the other hand, the enthalpic effect is a major controlling factor and is related to the presence of functional groups along the polymer chains. As expected, it was found that ether groups – owing to Lewis acid-based interactions (Park et al., 2011) – enhanced CO2 capture. This was also observed, albeit in a more pronounced way, in the case of the presence of amine groups (Lin and Park, 2011). It was found that NOHMs with polyethyleneimine could absorb up to 3 mmol/g of CO2, which is in the range of MEA-based aqueous solvents (Lin and Park, 2011). A good selectivity toward CO2 compared to N2 was also demonstrated (Lin and Park, 2011). The effect of another flue gas components, i.e., SO2, on the performance of NOHMs was also studied (Lin et al., 2013). No significant effect was observed in the presence of SO2 in the range of concentration typically encountered in post-combustion plants (Lin et al., 2013).
In addition to the selectivity, the recyclability of NOHMs was tested via a vacuum pressure swing process (Lin and Park, 2011; Park et al., 2011). No degradation of the material was noticed and the efficiency for CO2 capture was maintained for NOHMs deprived of task-specific functional groups. However, the presence of accessible amine groups led to a decrease in the capture capacity after the first cycle and it is anticipated that a mild thermal treatment, as in the case of TSILs, can lead to the release of chemisorbed CO2. The decrease in capture capacity upon the first cycle was related to the particular capture mechanism. The NOHMs exhibited amine groups, which reacted with CO2 in the same way as observed with TSILs. The pressure swing cycle that was applied was, therefore, not sufficient to break the bond formed between the amine group and CO2. Given the similar chemistry of the tested NOHMs to that of TSILs, it is expected that a thermal swing can enable full recovery of the initial capture capacity. This discussion has been added to the revised manuscript. Overall, since NOHMs can capture CO2 from two different effects of entropic and enthalpic contributions, one can desorb CO2 from NOHMs via pressure, temperature, or pressure–temperature swing processes.
Like TSILs, a major obstacle to the applications of NOHMs in the large scale is their high viscosity (up to 15,000 cP). Reducing the size of the nanocore was, therefore, explored as a way to decrease the viscosity in NOHMs (Petit et al., 2013b). Indeed, it was recently proposed to use polysilsesquioxane (POSS) cages as the core particles instead of the typically used larger SiO2 nanoparticles – POSS correspond to the smallest silica particles that exist. This approach led to a decrease in viscosity down to about 1,500 cP. Mixing NOHMs and water was also investigated as a way to decrease the viscosity of NOHMs. The viscosity was decreased down to about 8,000 and 500 cP for 15 and 70 wt% water contents, respectively. It was also found that water, while decreasing the CO2 capture capacity, significantly helped reducing the viscosity of the NOHMs, thereby enhancing CO2 diffusion (Petit et al., 2013a).
Conclusion
Amine-based solvents have been selected to move to the next stage of development in terms of CO2 capture with pilot-scale and demonstration-scale projects relying on these solvents. Aqueous amine-based solvents, such as MEA, have a great CO2 capture capacity and fast kinetics, while still facing some challenges associated with their high volatility causing fugitive emissions and loss of materials, corrosive nature, and high water content leading to relatively high energy consumption. Thus, there has been a desire to develop alternative CO2 capture solvents with a potentially lowered regeneration energy requirement.
In this review, we summarized new and promising developments in CO2 capture media, focusing on novel liquid or liquid-like materials including ILs, CO2-triggered switchable solvents, and NOHMs. These materials exhibit low and/or negligible vapor pressure, which would minimize or eliminate the issues of fugitive emissions or loss of materials during CO2 capture and solvent regeneration. In addition, being anhydrous, the problem of high parasitic energy consumption associated with water vaporization would be circumvented. Finally, these new materials are highly tunable, and therefore, they can capture CO2 via a range of chemical or physical mechanisms. This aspect allows for the CO2-binding energy to be tuned and for various desorption/regeneration processes to be used, such as thermal swing, pressure swing, polarity swing, or a combination of the three. A summary of the properties of the different anhydrous solvents discussed along with their merits/weaknesses is provided in Table 1. Overall, TSILs and CO2BOLs likely appear as the most promising candidates as alternative CO2 capture solvents. TSILs have been around for quite some time and exhibit an excellent tunability along with a high capture capacity. It was estimated that ILs can reduce the energy loss by 16% (CO2 capture with [bmim][Ac]), bringing the costs down for investment for the process by 11% (Zhang et al., 2012). A high capture capacity has also been reported with CO2BOLs, in some cases surpassing that of TSILs. CO2BOLs are characterized by a very low binding energy for CO2, and therefore benefit from a significantly lower energy requirement for solvent regeneration. In fact, it was calculated that, provided that the viscosity of CO2BOLs is below 20 cP, a 68% increase in the levelized cost of electricity could be reach with CO2BOLs as opposed to 75–100% typically reported for MEA aqueous solvent (Heldebrant, 2015). Comparatively to TSILs and CO2BOLs, relatively little efforts has been dedicated to the study of NOHMs with task-specific functional groups for CO2 capture. Given the tunability, chemistry, and zero vapor pressure, it is expected that they will behave similarly to TSILs. On the other hand, conventional ILs as well as NOHMs without task-specific groups are likely better suited for high-pressure separation processes rather than post-combustion CO2 capture. It must be noted that while one can find commercially available ILs, the other solvents discussed in the review as well as the TSILs exhibiting the highest capture capacity are not yet produced at a large scale.
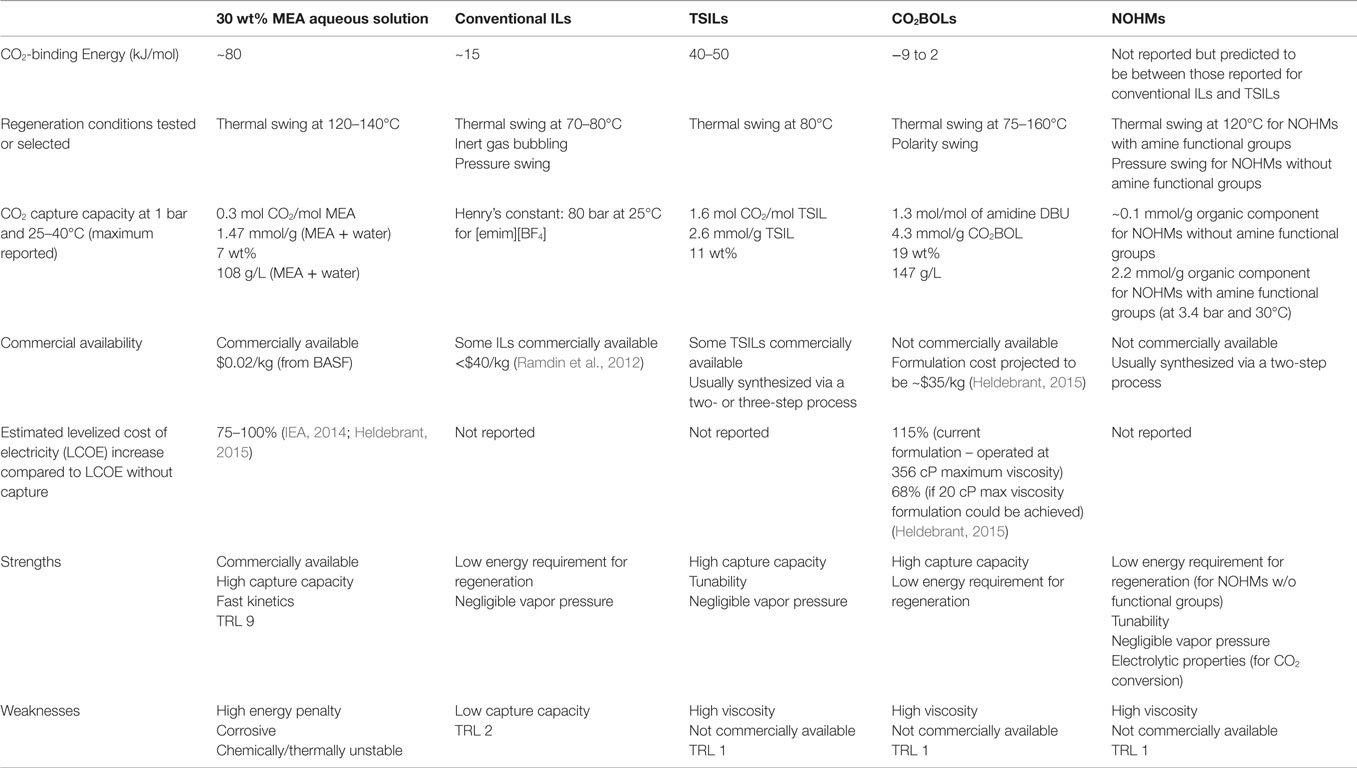
Table 1. Overview of the properties, merits, and weaknesses of the reviewed anhydrous solvents with respect to CO2 capture and compared to MEA aqueous solvent.
While TSILs, CO2BOLs, and NOHMs with task-specific groups exhibit real merits for CO2 capture, it is also certain that they must be improved to meet the requirement of large-scale application for CO2 capture. One of the key areas that should be further explored is the decrease of their viscosity. Many of these solvents are still highly viscous and while recent studies have shown ways to address this issue, their viscosities remain relatively high and do not allow them to be used in the same reactor set-up as amine solvents. In addition, further testing these materials using real flue gas streams is required to get an insight into their “real” performance. In addition, it becomes more and more important to couple experimental efforts to design materials with modeling work at the reactor and process scales to understand the set of key performance indicators a material must exhibit. Working across these scales will allow one to identify the most promising materials and provide guidance for materials scientists toward the design of improved solvents.
Conflict of Interest Statement
The authors declare that the research was conducted in the absence of any commercial or financial relationships that could be construed as a potential conflict of interest.
Acknowledgments
This publication was based on work supported by Award KUS-C1-018-02, made by King Abdullah University of Science and Technology (KAUST).
Abbreviations
b2Nic, 1-butyl-nicotinic acid butyl ester; bmim, 1-butyl-3-methylimidazolium; bupy, 1-butylpyridinium; DCA, dicyanamide; doc, docusate; emim, 1-ethyl-3-methylimidazolium; hmim, 1-hexyl-3-methylimidazolium; Met, methionine; N2224, triethylbutylammonium; N4444, tributylmethylammonium; P66614, trihexyl(tetradecyl)phosphonium; pabim, 1-n-propyl-amine-3-butyl-imidazolium; Pro, prolinate; Tf2N, bis[(trifluoromethyl)sulfonyl]imide; TfO, trifluoromethanesulfonate.
References
Agarwal, P., Qi, H., and Archer, L. A. (2009). The ages in a self-suspended nanoparticle liquid. Nano Lett. 10, 111–115. doi:10.1021/nl9029847
Aki, S. N. V. K., Mellein, B. R., Saurer, E. M., and Brennecke, J. F. (2004). High-pressure phase behavior of carbon dioxide with imidazolium-based ionic liquids. J. Phys. Chem. B 108, 20355–20365. doi:10.1021/jp046895+
Anderson, J. L., Dixon, J. K., and Brennecke, J. F. (2007). Solubility of CO2, CH4, C2H6, C2H4, O2, and N2 in 1-hexyl-3-methylpyridinium bis(trifluoromethylsulfonyl)imide: comparison to other ionic liquids. Acc. Chem. Res. 40, 1208–1216. doi:10.1021/ar7001649
Anthony, J. L., Anderson, J. L., Maginn, E. J., and Brennecke, J. F. (2005). Anion effects on gas solubility in ionic liquids. J. Phys. Chem. B 109, 6366–6374. doi:10.1021/jp046404l
Anthony, J. L., Maginn, E. J., and Brennecke, J. F. (2002). Solubilities and thermodynamic properties of gases in the ionic liquid 1-n-butyl-3-methylimidazolium hexafluorophosphate. J. Phys. Chem. B 106, 7315–7320. doi:10.1021/jp020631a
Babarao, R., Dai, S., and Jiang, D.-E. (2011). Understanding the high solubility of CO2 in an ionic liquid with the tetracyanoborate anion. J. Phys. Chem. B 115, 9789–9794. doi:10.1021/jp205399r
Baltus, R. E., Culbertson, B. H., Dai, S., Luo, H., and DePaoli, D. W. (2003). Low-pressure solubility of carbon dioxide in room-temperature ionic liquids measured with a quartz crystal microbalance. J. Phys. Chem. B 108, 721–727. doi:10.1021/jp036051a
Bara, J. E., Camper, D. E., Gin, D. L., and Noble, R. D. (2010). Room-temperature ionic liquids and composite materials: platform technologies for CO2 capture. Acc. Chem. Res. 43, 152–159. doi:10.1021/ar9001747
Bara, J. E., Gabriel, C. J., Carlisle, T. K., Camper, D. E., Finotello, A., Gina, D. L., et al. (2009). Gas separations in fluoroalkyl-functionalized room-temperature ionic liquids using supported liquid membranes. Chem. Eng. J. 147, 43. doi:10.1016/j.cej.2008.11.021
Bara, J. E., Gabriel, C. J., Lessmann, S., Carlisle, T. K., Finotello, A., Gin, D. L., et al. (2007). Enhanced CO2 separation selectivity in oligo(ethylene glycol) functionalized room-temperature ionic liquids. Ind. Eng. Chem. Res. 46, 5380–5386. doi:10.1021/ie070437g
Bates, E. D., Mayton, R. D., Ntai, I., and Davis, J. H. Jr. (2002). CO2 capture by a task-specific ionic liquid. J. Am. Chem. Soc. 124, 926–927. doi:10.1021/ja017593d
Beckman, E. J. (2004). A challenge for green chemistry: designing molecules that readily dissolve in carbon dioxide. Chem. Commun. 2004, 1885–1888. doi:10.1039/b404406c
Blanchard, L. A., Zhiyong, G., and Brennecke, J. F. (2001). High-pressure phase behavior of ionic liquid/CO2 systems. J. Phys. Chem. B 105, 8. doi:10.1021/jp003309d
Blasucci, V., Dilek, C., Huttenhower, H., John, E., Llopis-Mestre, V., Pollet, P., et al. (2009). One-component, switchable ionic liquids derived from siloxylated amines. Chem. Commun. 2009, 116–118. doi:10.1039/b816267k
Blasucci, V., Hart, R., Mestre, V. L., Hahne, D. J., Burlager, M., Huttenhower, H., et al. (2010). Single component, reversible ionic liquids for energy applications. Fuel 89, 1315–1319. doi:10.1016/j.fuel.2009.11.015
Blasuccia, V. M., Harta, R., Pollet, P., Liotta, C. L., and Eckerta, C. A. (2010). Reversible ionic liquids designed for facile separations. Fluid Phase Equilib. 294, 1–6. doi:10.1016/j.fluid.2010.04.005
Bogel-Łukasika, R., Matkowskaa, D., Zakrzewskaa, M. E., Bogel-Łukasika, E., and Hofmanc, T. (2010). The phase envelopes of alternative solvents (ionic liquid, CO2) and building blocks of biomass orgin (lactic acid, propionic acid). Fluid Phase Equilib. 295, 177–185. doi:10.1016/j.fluid.2010.05.013
Boot-Handford, M. E., Abanades, J. C., Anthony, E. J., Blunt, M. J., Brandani, S., Mac Dowell, N., et al. (2014). Carbon capture and storage update. Energy Environ. Sci. 7, 130–189. doi:10.1039/C3EE42350F
Bourlinors, A. B., Herreraj, R., Chalkias, N., Jiang, D. D., Zhang, Q., Archer, L. A., et al. (2005). Surface-functionalized nanoparticles with liquid-like behavior. Adv. Mater. 17, 234–237. doi:10.1002/adma.200401060
Bourlinos, A. B., Chowdhury, S. R., Jiang, D. D., An, Y.-U., Zhang, Q., Archer, L. A., et al. (2005a). Layered organosilicate nanoparticles with liquidlike behavior. Small 1, 80–82. doi:10.1002/smll.200400027
Bourlinos, A. B., Ray Chowdhury, S., Herrera, R., Jiang, D. D., Zhang, Q., Archer, L. A., et al. (2005b). Functionalized nanostructures with liquid-like behavior: expanding the gallery of available nanostructures. Adv. Funct. Mater. 15, 1285–1290. doi:10.1002/adfm.200500076
Bourlinos, A. B., Georgakilas, V., Tzitzios, V., Boukos, N., Herrera, R., and Giannelis, E. P. (2006). Functionalized carbon nanotubes: synthesis of meltable and amphiphilic derivatives. Small 2, 1188–1191. doi:10.1002/smll.200600221
Cadena, C., Anthony, J. L., Shah, J. K., Morrow, T. I., Brennecke, J. F., and Maginn, E. J. (2004). Why is CO2 so soluble in imidazolium-based ionic liquids? J. Am. Chem. Soc. 126, 5300–5308. doi:10.1021/ja039615x
Camper, D., Bara, J. E., Gin, D. L., and Noble, R. D. (2008). Room-temperature ionic liquid-amine solutions: tunable solvents for efficient and reversible capture of CO2. Ind. Eng. Chem. Res. 47, 8496–8498. doi:10.1021/ie801002m
Carlisle, T. K., Bara, J. E., Gabriel, C. J., Noble, R. D., and Gin, D. L. (2008). Interpretation of CO2 solubility and selectivity in nitrile-functionalized room-temperature ionic liquids using a group contribution approach. Ind. Eng. Chem. Res. 47, 7005–7012. doi:10.1021/ie8001217
Carvalho, P. J., Álvarez, V. H., Marrucho, I. M., Aznar, M., and Coutinho, J. A. P. (2010). High carbon dioxide solubilities in trihexyltetradecylphosphonium-based ionic liquids. J. Supercrit. Fluids 52, 258–265. doi:10.1016/j.supflu.2010.02.002
Dzyuba, S. V., and Bartsch, R. A. (2002). Influence of structural variations in 1-alkyl(aralkyl)-3-methylimidazolium hexafluorophosphates and bis-(trifluoromethylsulfonyl)imides on physical properties of the ionic liquids. Chem. Phys. Chem. 3, 161–166. doi:10.1002/1439-7641(20020215)3:2<161::AID-CPHC161>3.0.CO;2-3
Ferguson, L., and Scovazzo, P. (2007). Solubility, diffusivity, and permeability of gases in phosphonium-based room temperature ionic liquids: data and correlations. Ind. Eng. Chem. Res. 46, 1369–1374. doi:10.1021/ie0610905
Ghosal, K., Chern, R. T., Freeman, B. D., Daly, W. H., and Negulescu, I. I. (1996). Effects of basic substituents on gas sorption and permeation in polysulfone. Macromolecules 29, 4360–4369. doi:10.1021/ma951310i
Gonzalez-Miquel, M., Talreja, M., Ethier, A. L., Flack, K., Switzer, J. R., Biddinger, E. J., et al. (2012). COSMO-RS studies: structure-property relationships for CO2 capture by reversible ionic liquids. Ind. Eng. Chem. Res. 51, 16066–16073. doi:10.1021/ie302449c
Goodrich, B. F., de la Fuente, J. C., Gurkan, B. E., Lopez, Z. K., Price, E. A., Huang, Y., et al. (2011). Effect of water and temperature on absorption of CO2 by amine-functionalized anion-tethered ionic liquids. J. Phys. Chem. B 115, 9140–9150. doi:10.1021/jp2015534
Gupta, K. M., and Jiang, J. (2014). Systematic investigation of nitrile based ionic liquids for CO2 capture: a combination of molecular simulation and ab initio calculation. J. Phys. Chem. C 118, 3110–3118. doi:10.1021/jp411434g
Gurkan, B., Goodrich, B. F., Mindrup, E. M., Ficke, L. E., Massel, M., Seo, S., et al. (2010a). Molecular design of high capacity, low viscosity, chemically tunable ionic liquids for CO2 capture. J. Phys. Chem. Lett. 1, 3494–3499. doi:10.1021/jz101533k
Gurkan, B. E., de la Fuente, J. C., Mindrup, E. M., Ficke, L. E., Goodrich, B. F., Price, E. A., et al. (2010b). Equimolar CO2 absorption by anion-functionalized ionic liquids. J. Am. Chem. Soc. 132, 2116–2117. doi:10.1021/ja909305t
Gutowski, K. E., and Maginn, E. (2008). Amine-functionalized task-specific ionic liquids: a mechanistic explanation for the dramatic increase in viscosity upon complexation with CO2 from molecular simulation. J. Am. Chem. Soc. 130, 14690–14704. doi:10.1021/ja804654b
Harper, N. D., Nizio, K. D., Hendsbee, A. D., Masuda, J. D., Robertson, K. N., Murphy, L. J., et al. (2011). Survey of carbon dioxide capture in phosphonium-based ionic liquids and end-capped polyethylene glycol using DETA (DETA = diethylenetriamine) as a model absorbent§. Ind. Eng. Chem. Res. 50, 2822–2830. doi:10.1021/ie101734h
Heldebrant, D. J. (2015). CO2 Binding Organic Liquids Gas Capture with Polarity Swing Assisted Regeneration. Available at: http://www.osti.gov/scitech/servlets/purl/1185193
Heldebrant, D. J., Koech, P. K., Ang, M. T. C., Liang, C., Rainbolt, J. E., Yonker, C. R., et al. (2010a). Reversible zwitterionic liquids, the reaction of alkanol guanidines, alkanol amidines, and diamines with CO2. Green Chem. 12, 713–721. doi:10.1039/b924790d
Heldebrant, D. J., Koech, P. K., and Yonker, C. R. (2010b). A reversible zwitterionic SO2-binding organic liquid. Energy Environ. Sci. 3, 111–113. doi:10.1039/B916550A
Heldebrant, D. J., Koech, P. K., Rainbolt, J. E., Zheng, F., Smurthwaite, T., Freeman, C. J., et al. (2011). Performance of single-component CO2-binding organic liquids (CO2BOLs) for post combustion CO2 capture. Chem. Eng. J. 171, 794–800. doi:10.1016/j.cej.2011.02.012
Heldebrant, D. J., Yonker, C. R., Jessop, P. G., and Phan, L. (2008). Organic liquid CO2 capture agents with high gravimetric CO2 capacity. Energy Environ. Sci. 1, 487–493. doi:10.1039/b809533g
Heldebrant, D. J., Yonker, C. R., Jessop, P. G., and Phan, L. (2009). CO2-binding organic liquids (CO2 BOLs) for post-combustion CO2 capture. Energy Procedia 1, 1187–1195. doi:10.1016/j.egypro.2009.01.156
Hojniak, S. D., Khan, A. L., Hollóczki, O., Kirchner, B., Vankelecom, I. F. J., Dehaen, W., et al. (2013). Separation of carbon dioxide from nitrogen or methane by supported ionic liquid membranes (SILMs): influence of the cation charge of the ionic liquid. J. Phys. Chem. B 117, 15131–15140. doi:10.1021/jp409414t
Husson, P., Pison, L., Jacquemin, J., and Gomes, M. F. C. (2010). Influence of water on the carbon dioxide absorption by 1-ethyl-3-methylimidazolium bis(trifluoromethylsulfonyl)amide. Fluid Phase Equilib. 294, 98–104. doi:10.1016/j.fluid.2010.02.021
Jessop, P. G., Heldebrant, D. J., Li, X., Eckert, C. A., and Liotta, C. L. (2005). Reversible nonpolar-to-polar solvent. Nature 436, 1102–1102. doi:10.1038/4361102a
Kamps, ÁP.-S., Tuma, D., Xia, J., and Maurer, G. (2003). Solubility of CO2 in the Ionic Liquid [BMIM][PF6]. J. Chem. Eng. Data 48, 746–749. doi:10.1021/je034023f
Kazarian, S. G., Briscoe, B. J., and Welton, T. (2000). Combining ionic liquids and supercritical fluids: in situ ATR-IR study of CO2 dissolved in two ionic liquids at high pressures. Chem. Commun. 2047–2048. doi:10.1039/b005514j
Keith, D. W. (2009). Why capture CO2 from the atmosphere. Science 325, 1654–1655. doi:10.1126/science.1175680
Kim, M., and Park, J.-W. (2010). Reversible, solid state capture of carbon dioxide by hydroxylated amidines. Chem. Commun. 46, 2507–2509. doi:10.1039/b921688j
Koech, P. K., Zhang, J., Kutnyakov, I. V., Cosimbescu, L., Lee, S.-J., Bowden, M. E., et al. (2013). Low viscosity alkanolguanidine and alkanolamidine liquids for CO2 capture. RSC Adv. 3, 566–572. doi:10.1039/C2RA22801G
Koel, M. N. (2009). Ionic Liquids in Chemical Analysis: Analytical Chemistry Series, Vol. xxxi. Boca Raton, FL: CRC Press, 414.
Lei, Z., Dai, C., and Chen, B. (2014). Gas solubility in ionic liquids. Chem. Rev. 114, 1289–1326. doi:10.1021/cr300497a
Lei, Z., Qi, X., Zhu, J., Li, Q., and Chen, B. (2012). Solubility of CO2 in acetone, 1-butyl-3-methylimidazolium tetrafluoroborate, and their mixtures. J. Chem. Eng. Data 57, 3458–3466. doi:10.1021/je300611q
Lin, K.-Y. A., and Park, A.-H. A. (2011). Effects of bonding types and functional groups on CO2 capture using novel multiphase systems of liquid-like nanoparticle organic hybrid materials. Environ. Sci. Technol. 45, 6633–6639. doi:10.1021/es200146g
Lin, K.-Y. A., Park, Y., Petit, C., and Park, A.-H. A. (2014). Thermal stability, swelling behavior and CO2 absorption properties of nanoscale ionic materials (NIMs). RSC Adv. 4, 65195–65204. doi:10.1039/C4RA10722E
Lin, K.-Y. A., Petit, C., and Park, A.-H. A. (2013). Effect of SO2 on CO2 capture using liquid-like nanoparticle organic hybrid materials. Energy Fuels 27, 4167–4174. doi:10.1021/es200146g
Liu, Y., Jessop, P. G., Cunningham, M., Eckert, C. A., and Liotta, C. L. (2006). Switchable surfactants. Science 313, 958–960. doi:10.1126/science.1128142
Liu, Z., Wu, W., Han, B., Dong, Z., Zhao, G., Wang, J., et al. (2003). Study on the phase behaviors, viscosities, and thermodynamic properties of CO2/[C4mim][PF6]/methanol system at elevated pressures. Chem. Eur. J. 9, 3897–3903. doi:10.1002/chem.200204647
Luo, X., Guo, Y., Ding, F., Zhao, H., Cui, G., Li, H., et al. (2014a). Significant improvements in CO2 capture by pyridine-containing anion-functionalized ionic liquids through multiple-site cooperative interactions. Angew. Chem. Int. Ed. 53, 7053–7057. doi:10.1002/anie.201400957
Luo, X. Y., Ding, F., Lin, W. J., Qi, Y. Q., Li, H. R., and Wang, C. M. (2014b). Efficient and energy-saving CO2 capture through the entropic effect induced by the intermolecular hydrogen bonding in anion-functionalized ionic liquids. J. Phys. Chem. Lett. 5, 381–386. doi:10.1021/jz402531n
MacDowell, N., Florin, N., Buchard, A., Hallett, J., Galindo, A., Jackson, G., et al. (2010). An overview of CO2 capture technologies. Energy Environ. Sci. 3, 1645–1669. doi:10.1039/c004106h
MacFarlane, D. R., Tachikawa, N., Forsyth, M., Pringle, J. M., Howlett, P. C., Elliott, G. D., et al. (2014). Energy applications of ionic liquids. Energy Environ. Sci. 7, 232–250. doi:10.1039/C3EE42099J
Mahurin, S. M., Lee, J. S., Baker, G. A., Luo, H., and Dai, S. (2010). Performance of nitrile-containing anions in task-specific ionic liquids for improved CO2/N2 separation. J. Memb. Sci. 353, 177–183. doi:10.1016/j.memsci.2010.02.045
Makino, T., Kanakubo, M., and Umecky, T. (2014). CO2 solubilities in ammonium bis(trifluoromethanesulfonyl)amide ionic liquids: effects of ester and ether groups. J. Chem. Eng. Data 59, 1435–1440. doi:10.1021/je400971q
Mathias, P. M., Afshar, K., Zheng, F., Bearden, M. D., Freeman, C. J., Andrea, T., et al. (2013). Improving the regeneration of CO2-binding organic liquids with a polarity change. Energy Environ. Sci. 6, 2233–2242. doi:10.1039/c3ee41016a
Mortazavi-Manesh, S., Satyro, M., and Marriott, R. A. (2011). A semi-empirical Henry’s law expression for carbon dioxide dissolution in ionic liquids. Fluid Phase Equilib. 307, 208–215. doi:10.1016/j.fluid.2011.05.006
Muldoon, M. J., Aki, S. N. V. K., Anderson, J. L., Dixon, J. K., and Brennecke, J. F. (2007). Improving carbon dioxide solubility in ionic liquids. J. Phys. Chem. B 111, 9001–9009. doi:10.1021/jp071897q
Orr, J. F. M. (2009). CO2 capture and storage: are we ready? Energy Environ. Sci. 2, 449–458. doi:10.1039/b822107n
Park, Y., Decatur, J., Lin, K.-Y. A., and Park, A.-H. A. (2011). Investigation of CO2 capture mechanisms of liquid-like nanoparticle organic hybrid materials via structural characterization. Phys. Chem. Chem. Phys. 13, 18115–18122. doi:10.1039/c1cp22631b
Park, Y., Petit, C., Han, P., and Alissa Park, A.-H. (2014). Effect of canopy structures and their steric interactions on CO2 sorption behavior of liquid-like nanoparticle organic hybrid materials. RSC Adv. 4, 8723–8726. doi:10.1039/c3ra46801a
Petit, C., Bhatnagar, S., and Park, A.-H. A. (2013a). Effect of water on the physical properties and carbon dioxide capture capacities of liquid-like nanoparticle organic hybrid materials and their corresponding polymers. J. Colloid Interface Sci. 407, 102–108. doi:10.1016/j.jcis.2013.05.065
Petit, C., Lin, K.-Y. A., and Park, A.-H. A. (2013b). Design and characterization of liquidlike POSS-based hybrid nanomaterials synthesized via ionic bonding and their interactions with CO2. Langmuir 29, 12234–12242. doi:10.1021/la4007923
Petit, C., Park, Y., Lin, K.-Y. A., and Park, A.-H. A. (2012). Spectroscopic investigation of the canopy configurations in nanoparticle organic hybrid materials of various grafting densities during CO2 capture. J. Phys. Chem. C 116, 516–525. doi:10.1021/jp210391c
Phan, L., Andreatta, J. R., Horvey, L. K., Edie, C. F., Luco, A.-L., Mirchandani, A., et al. (2008). Switchable-polarity solvents prepared with a single liquid component. J. Org. Chem. 73, 127–132. doi:10.1021/jo7017697
Ramdin, M., de Loos, T. W., and Vlugt, T. J. H. (2012). State-of-the-art of CO2 capture with ionic liquids. Ind. Eng. Chem. Res. 51, 8149–8177. doi:10.2533/chimia.2013.711
Rochelle, G. T. (2009). Amine scrubbing for CO(2) capture. Science 325, 1652–1654. doi:10.1126/science.1176731
Rodriguez, R., Herrera, R., Archer, L. A., and Giannelis, E. P. (2008). Nanoscale ionic materials. Adv. Mater. 20, 4353–4358. doi:10.1002/adma.200801975
Romanos, G. E., Zubeir, L. F., Likodimos, V., Falaras, P., Kroon, M. C., Iliev, B., et al. (2013). Enhanced CO2 capture in binary mixtures of 1-alkyl-3-methylimidazolium tricyanomethanide ionic liquids with water. J. Phys. Chem. B 117, 12234–12251. doi:10.1021/jp407364e
Sairi, N. A., Yusoff, R., Alias, Y., and Aroua, M. K. (2011). Solubilities of CO2 in aqueous N-methyldiethanolamine and quanidinium trifluoromethanesulfonate ionic liquid systems at elevated pressures. Fluid Phase Equilib. 300, 89–94. doi:10.1016/j.fluid.2010.10.011
Sarbu, T., Styranec, T. J., and Beckman, E. J. (2000). Design and synthesis of low cost, sustainable CO2-philes. Ind. Eng. Chem. Res. 39, 4678–4683. doi:10.1021/ie0003077
Seo, S., Quiroz-Guzman, M., DeSilva, M. A., Lee, T. B., Huang, Y., Goodrich, B. F., et al. (2014). Chemically tunable ionic liquids with aprotic heterocyclic anion (AHA) for CO2 capture. J. Phys. Chem. B 118, 5740–5751. doi:10.1021/jp502279w
Sistla, Y. S., and Khanna, A. (2011). Validation and prediction of the temperature-dependent Henry’s constant for CO2-ionic liquid systems using the conductor-like screening model for realistic solvation (COSMO-RS). J. Chem. Eng. Data 56, 4045–4060. doi:10.1021/je200486c
Soutullo, M. D., Odom, C. I., Wicker, B. F., Henderson, C. N., Stenson, A. C., and Davis, J. H. (2007). Reversible CO2 capture by unexpected plastic-, resin-, and gel-like ionic soft materials discovered during the combi-click generation of a TSIL library. Chem. Mater. 19, 3581–3583. doi:10.1021/cm0705690
Stevanovic, S., Podgoršek, A., Pádua, A. A. H., and Costa Gomes, M. F. (2012). Effect of water on the carbon dioxide absorption by 1-alkyl-3-methylimidazolium acetate ionic liquids. J. Phys. Chem. B 116, 14416–14425. doi:10.1021/jp3100377
Switzer, J. R., Ethier, A. L., Flack, K. M., Biddinger, E. J., Gelbaum, L., Pollet, P., et al. (2013). Reversible ionic liquid stabilized carbamic acids: a pathway toward enhanced CO2 capture. Ind. Eng. Chem. Res. 52, 13159–13163. doi:10.1021/ie4018836
Switzer, J. R., Ethier, A. L., Hart, E. C., Flack, K. M., Rumple, A. C., Donaldson, J. C., et al. (2014). Design, synthesis, and evaluation of nonaqueous silylamines for efficient CO2 capture. ChemSusChem 7, 299–307. doi:10.1002/cssc.201300438
Van Ginderen, P., Herrebout, W. A., and van der Veken, B. J. (2003). van der Waals complex of dimethyl ether with carbon dioxide. J. Phys. Chem. A 107, 5391–5396. doi:10.1021/jp034553i
Wang, G., Hou, W., Xiao, F., Geng, J., Wu, Y., and Zhang, Z. (2011). Low-viscosity triethylbutylammonium acetate as a task-specific ionic liquid for reversible CO2 absorption. J. Chem. Eng. Data 56, 1125–1133. doi:10.1021/je101014q
Wu, H., Shah, J. K., Tenney, C. M., Rosch, T. W., and Maginn, E. J. (2011). Structure and dynamics of neat and CO2-reacted ionic liquid tetrabutylphosphonium 2-cyanopyrrolide. Ind. Eng. Chem. Res. 50, 8983–8993. doi:10.1021/ie200518f
Yu, G., Zhang, S., Zhou, G., Liu, X., and Chen, X. (2007). Structure, interaction and property of amino-functionalized imidazolium ILs by molecular dynamics simulation and Ab initio calculation. AIChE J. 53, 3210–3221. doi:10.1002/aic.11339
Yu, H.-Y., and Koch, D. L. (2010). Structure of solvent-free nanoparticle-organic hybrid materials. Langmuir 26, 16801–16811. doi:10.1021/la102815r
Zhang, S., Chen, Y., Ren, R. X.-F., Zhang, Y., Zhang, J., and Zhang, X. (2005). Solubility of CO2 in sulfonate ionic liquids at high pressure. J. Chem. Eng. Data 50, 230–233. doi:10.1021/je0497193
Zhang, X., Liu, X., Yao, X., and Zhang, S. (2011). Microscopic structure, interaction, and properties of a guanidinium-based ionic liquid and its mixture with CO2. Ind. Eng. Chem. Res. 50, 8323–8332. doi:10.1021/ie1025002
Zhang, X., Zhang, X., Dong, H., Zhao, Z., Zhang, S., and Huang, Y. (2012). Carbon capture with ionic liquids: overview and progress. Energy Environ. Sci. 5, 6668–6681. doi:10.1039/c2ee21152a
Zheng, Y., Zhang, J., Lan, L., Yu, P., Rodriguez, R., Herrera, R., et al. (2010). Preparation of solvent-free gold nanofluids with facile self-assembly technique. Chemphyschem 11, 61–64. doi:10.1002/cphc.200900640
Keywords: CO2 capture, CO2-binding organic liquids, ionic liquids, nanoparticle organic hybrid materials, novel liquid solvent
Citation: Park Y, Lin K-YA, Park A-HA and Petit C (2015) Recent advances in anhydrous solvents for CO2 capture: ionic liquids, switchable solvents, and nanoparticle organic hybrid materials. Front. Energy Res. 3:42. doi: 10.3389/fenrg.2015.00042
Received: 30 June 2015; Accepted: 03 September 2015;
Published: 01 October 2015
Edited by:
David J. Heldebrant, Pacific Northwest National Laboratory, USAReviewed by:
Niall Mac Dowell, Imperial College London, UKJoshuah K. Stolaroff, Lawrence Livermore National Laboratory, USA
Copyright: © 2015 Park, Lin, Park and Petit. This is an open-access article distributed under the terms of the Creative Commons Attribution License (CC BY). The use, distribution or reproduction in other forums is permitted, provided the original author(s) or licensor are credited and that the original publication in this journal is cited, in accordance with accepted academic practice. No use, distribution or reproduction is permitted which does not comply with these terms.
*Correspondence: Ah-Hyung Alissa Park, Departments of Earth and Environmental Engineering and Chemical Engineering, Lenfest Center for Sustainable Energy, Columbia University, 1038A SW Mudd, Mail Code: 4711, 500 West 120th Street, New York, NY 10027–6699, USA, ap2622@columbia.edu;
Camille Petit, Department of Chemical Engineering, Imperial College ACEX Building, London, SW7 2AZ, UK, camille.petit@imperial.ac.uk