- 1Department of Materials Science and Engineering, Politecnico di Torino, Turin, Italy
- 2Central Electrochemical Research Institute (CSIR-CECRI), Karaikudi, India
This review article mainly encompasses on the state-of-the-art electrolytes for lithium–sulfur batteries. Different strategies have been employed to address the issues of lithium–sulfur batteries across the world. One among them is identification of electrolytes and optimization of their properties for the applications in lithium–sulfur batteries. The electrolytes for lithium–sulfur batteries are broadly classified as (i) non-aqueous liquid electrolytes, (ii) ionic liquids, (iii) solid polymer, and (iv) glass-ceramic electrolytes. This article presents the properties, advantages, and limitations of each type of electrolytes. Also, the importance of electrolyte additives on the electrochemical performance of Li–S cells is discussed.
Introduction
The global warming and depletion of fossil fuel resources have accelerated immense research on energy storage devices unquestionably (Tarascon, 2001). In response to the modern society, it is now essential to develop new, low-cost and environmental friendly energy conversions and storage systems with new advanced materials (Arico et al., 2005; Bruce et al., 2008; Goodenough and Kim, 2010; Scrosati, 2011). Undoubtedly, lithium-ion battery is one of the great successes of modern electrochemistry due to its appealing properties such as high single cell voltage, no-memory effect, long cycle life, and high energy density. The fundamental aspects of lithium-ion batteries, working principles, and their limitations can be understood from numerous review articles (Whittingham, 2004) and dedicated books (Schalkwijk and Scrosati, 2002). The state-of-the-art lithium-ion batteries are composed of a carbonaceous anode and lithium transition metal oxide cathode separated by a polyolfine porous separator soaked in a non-aqueous liquid electrolyte (Manuel Stephan, 2006). The lithium-ion battery has become an inevitable power source not only for portable electronic devices such as laptop computers, cellular phones, and MP3 players but also find applications in satellites (Santoni et al., 2002) and in medical equipments (Bock et al., 2012). Nevertheless, with the existing insertion cathode materials (e.g., LiCoO2, LiFePO4, etc.), lithium-ion batteries have attained a maximum discharge capacity of approximately 250 mAh g−1 (with a theoretical energy density of 800 Wh kg−1), which is not sufficient to meet out the demand of key markets such as transport and power grid applications (Lee et al., 2011). Obviously, intense research has been accelerated to find alternative electrochemical lithium-based power systems across the world. Among the systems known today, both Li–S and Li–O2 are expected to fulfill the requirements of mankind with enhanced capacity and energy density. Nevertheless, so many technological and scientific problems remain unsolved in Li2O systems (Bruce et al., 2012). The Li–S batteries have inspired many researchers recently, because sulfur is electrochemically active and can accept up to two electrons per atom approximately at 2.1 V vs. Li/Li+. It has a high-theoretical capacity of 1675 mAh g−1, which corresponds to an energy density of 2600 Wh kg−1 or 2800 Wh l−1 based on weight or volume, respectively. However, their practical applications are impeded by several major issues (Cakan et al., 2013; Xia et al., 2013).
Unfortunately, sulfur undergoes a series of compositional and structural changes during cycling, which involves soluble polysulfides and insoluble sulfides (Cheon et al., 2003a,b; Mikhaylik and Akridge, 2004). The typical Li–S batteries are composed of a lithium metal anode, an organic liquid electrolyte, and a sulfur composite cathode as depicted in Figure 1.
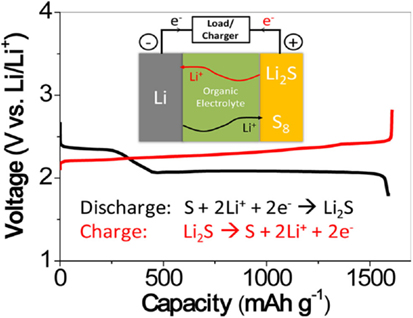
Figure 1. Schematic diagram of a typical Li–S cell [adopted from Manthiram et al. (2013)].
Although the concept of elemental sulfur as positive electrode material was introduced almost five decades ago by Hebert and Ulam (1962) its technological importance was only recognized in the beginning of year 2000. In the recent years, many review articles are available in the literature describing the working principles, advantages, and limitations of lithium/sulfur batteries (Ji and Nazar, 2010; Barghamadi et al., 2013; Bresser et al., 2013; Manthiram et al., 2013; Nazar and Evers, 2013). Also, numerous articles appear on the fundamental chemistry (Akridge et al., 2004; Zhang, 2013a), performance of modified cathode materials (Ji et al., 2009; Liang et al., 2009; Yang et al., 2011; Fu et al., 2012), their fading mechanism (Diao et al., 2013), AFM, and Raman characterizations (Aurbach et al., 2009; Elazari et al., 2010; Yeon et al., 2012; Hagen et al., 2013) in situ XRD (Canas et al., 2013a) and impedance analysis (Yuan et al., 2009; Canas et al., 2013b). The preparation and characterization of cathode and anode materials for Li–S batteries are beyond the scope of this article. This article mainly encompasses the basic properties of electrolytes, their types, electrolyte additives, their advantages and limitations for the applications in Li–S batteries. The electrolytes for Li–S batteries can be classified as (i) non-aqueous liquid, (ii) ionic liquids, (iii) solid polymer electrolytes, and (iv) superionic conductors.
Lithium-ion reacts with elemental sulfur (S8) and produces lithium polysulfides of general formula Li2Sn, e.g., Li2S8, Li2S6. Upon discharge the length of the polysulfide is shortened as the sulfur is being further reduced. The overall reaction is
The elemental sulfur is a promising electrode material, which is available abundantly, cheap, non-toxic, and environmentally benign. Despite all these advantages sulfur suffers from poor electronic conductivity.
Figure 2 shows typical discharge–charge profile of a Li–S cell. The discharge process is generally divided into four reduction regions depending upon the phase changes of sulfur species.
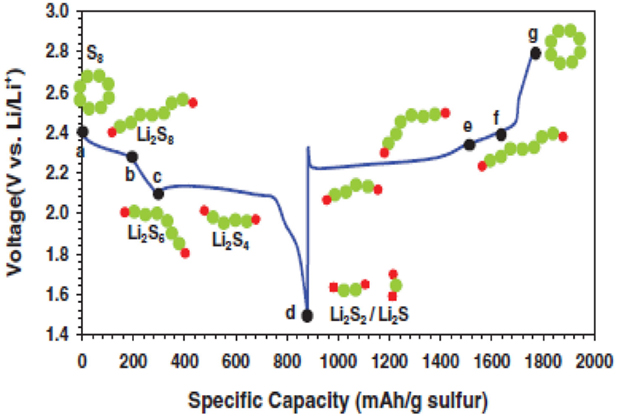
Figure 2. First discharge and charge profiles of a Li–S cell between 1.5 and 2.8 V vs. Li+/Li [adopted from Barghamadi et al. (2013)].
According to Zhang (2013a), the first upper voltage plateau between 2.2 and 2.3 V corresponds to a solid–liquid two-phase reduction from elemental sulfur to Li2S8. This process results in formation of huge voids in cathode due to the dissolution of Li2S8 into liquid electrolyte.
In the second region, the cell voltage suddenly reduced with an increase in viscosity of electrolyte solution. The solution’s viscosity reaches a maximum value at the end of the discharge region, which corresponds to the equation;
The low voltage plateau, which occurs between 1.9 and 2.1 V offers majority of the total capacity of Li–S cell. In this third region, a liquid-solvent two-phase reduction takes place in which dissolved low-order polysulfide (PS) to insoluble Li2S2 or Li2S.
In the fourth region, a solid–solid reduction from insoluble Li2S2 to Li2S process takes place, which is kinetically slow and suffers from high polarization due to the non-conductive and insoluble natures of Li2S2 and Li2S.
According to Mikhaylik and Akridge (2004), during the first charge polysulfides do not transform into elemental sulfur in a Li–S cell. Instead, in the second and subsequent charges, the higher-order polysulfides, which are generated at the sulfur electrode diffuse to lithium electrode and form lower-order polysulfides due to parasitic reaction. These species diffuse back again to the sulfur electrode as polysulfide and where polysulfide, of higher order are formed as a result of shuttle mechanism.
The sulfur and lithium sulfides, which are strong insulators, will easily dissolve in common organic liquid electrolytes. Spontaneously, they diffuse through the liquid electrolyte and subsequently leads to self-discharge and thereby increasing the viscosity of the electrolyte solution. These drawbacks lead the lithium/sulfur batteries to poor cycle life, low specific capacity, and lower efficiency.
Non-Aqueous Liquid Electrolytes
In order to improve the electrochemical performance of Li–S batteries, different strategies have been adopted, which also include the optimization of the electrolyte compositions (Barchasz et al., 2013a). The dissolution of polysulfides, LiSx, leads to an unfavorable side reactions and adversely deteriorates the performance of the Li–S cells. It is well known that the carbonate-based solvents such as ethylene carbonate (EC) and diethyl carbonate (DEC) react with polysulfides irreversibly (Barchasz et al., 2013b; Barghamadi et al., 2014). In the beginning, dimethyl sulfoxide (DMSO) was explored as a possible electrolyte solvent for lithium–sulfur batteries; however, the sulfur can be reduced only to with an efficiency of 25% (Brummer et al., 1976). On the other hand, a 50% efficiency was achieved when dimethyl acetamide (DMAc) was used with a corresponding reduction of (Abraham et al., 1978). Although, tetrahydrofuran (THF) exhibited about 100% efficiency at 50°C, unfortunately, it is found to be thermodynamically unstable with lithium metal (Kock and Young, 1977; Yamin and Peled, 1983).
In a pioneering work, Rauh et al. (1979) reported electrolytes with high basicity can dissolve huge amount of lithium polysulfides. Also, the sulfur solubility can go up to 10M in DMSO or ethers like THF. Based on the electrochemical and spectroscopic studies of poly sulfides in non-aqueous solutions, the same group (Rauh et al., 1977) concluded that dynamic equilibrium, redox chemistry, kinetics are strongly affected by solvent complexation. Unquestionably, the selection of solvents, which is stable against nucleophilic attack of polysulfides is mandatory. 1,3-dioxolane (DIOX) has a good polysulfide solubility and high stability in contact with lithium metal forms a passive solid-electrolyte interphase. Tetraethylene glycol dimethylether (TEGDME) has a glyme structure with high donor number (DN = 18.6) and with low dielectric constant of εr = 7.9, which can dissociate both lithium salt and sulfur active material. The ether-based electrolytes have high donor number and co-ordinate with Lewis acidic cation of Li+. 1,2-dimethoxyethane (DME) also has high lithium polysulfide dissolution ability and can provide sufficient amount of active materials (Tachikawa et al., 2011).
Generally, both DIOX and glyme solvents exhibit higher sulfur solubility (Peled et al., 1989; Chu et al., 2000; Shim et al., 2002). The impedance spectroscopic studies on Li–S cells containing 1M solution of LiClO4 in sulfonane have been reported by Kolosnitsyn et al. (2011). It is suggested that the electrochemical processes in Li–S cells were controlled by diffusion in the surface layer on the sulfur electrode at high degrees of charge–discharge and by the transport properties of the electrolyte system at moderate degrees of charging. More importantly, the rate of shuttling of sulfur is determined by the solubility of lithium polysulfides in electrolytes, the transport properties of electrolyte systems and the dissolution rates on the electrodes. The structure of the positive electrode also significantly affects the transfer rate (Kolosnitsyn and Karaseva, 2008). The shuttling of polysulfide, although protects the Li–S batteries from overcharging, it adversely induces the self-discharge. Moreover, in Li–S batteries, the dentritic formation is much lower than other type of lithium batteries with metallic lithium (Chang et al., 2002). Due to the instability of ether solvents at higher potential values, they are not widely used in lithium batteries; however, they find applications in Li–S system because of 3 V vs. Li+/Li (Choi et al., 2008; Mikhaylik, 2008; Mikhaylik et al., 2010). The importance of solvation ability and additives has been illustrated by Barchasz et al. (2013b) by using different combinations of TEGDME/DIOX binary electrolytes. The authors have also demonstrated that the lithium salt concentration also plays a vital role for the formation of good passivation and helps to reduce the shuttle mechanism and capacity loss. The solvation ability is also a key parameter for better electrochemical performance of Li–S cells.
By employing electrochemical and in situ X-ray absorption spectroscopy, Gao et al. (2011) demonstrated the influence of electrolytes on the charge–discharge studies of Li–S cells. A discharge capacity of about 1000 mAh g−1 was delivered by the Li–S cell when TEGDME and DOL/DME were used as electrolytes. While using PC/EC/DEC and EMS/DEC as electrolyte the cell delivered almost no capacity. On the basis of the electrochemical study, DOL/DME and TEGDME have been suggested as appropriate and promising solvents for the electrolytes of Li–S batteries. Although the discharge products of sulfur in DOL/DME and TEGDME are similar, the authors found a faster self-discharge and a more complete reduction of solution-phase sulfur species upon discharge for the combination of electrolytes, DOL/DME. While comparing the effect of lithium salts, LiPF6, LiCF3SO3, and LiClO4 a relatively stable capacity is seen for LiClO4; however, poor safety concerns hamper it from practical application.
The electrochemical performance of LiClO4/DOL/DME as electrolyte was reported by Wang et al. (2010). Impedance analysis indicated that the formation of impermeable layer on the surface of cathode considerably increased the interfacial resistance of the battery. They also suggested the combination of electrolyte DME:DOL of 2:1 v/v is capable of delivering a discharge capacity of 1200 mAh g−1 during its initial stage and retained a discharge capacity of 800 mAh g−1 even after 20 cycles.
The cycling behavior of Li/TEGDME/S cells was examined at low temperature by Rhu et al. (2006). The cell delivered a discharge capacity of 1030 and 357 mAh g−1 at 20 and −10°C, respectively. Upon addition of 1,3-dioxalane and methyl acetate to the TEGDME the discharge capacity was enhanced to 1342 and 994 mAh g−1, respectively. The better performance of the cell has been attributed to the reduced viscosity of the electrolyte at lower temperature. The electrochemical properties of ether-based electrolytes have also been reported by Barchasz et al. (2013b). The same group (Barchasz et al., 2012) has added polysulfide (0.1M Li2S6) as co-salt in order to provide additional capacity and compensate the active mass loss. Unfortunately, it adversely reduced the capacity and cycle life of the cell. On contrary, to the above work Chen et al. (2013) achieved a dramatic increase in capacity, cyclability, and rate capability by optimizing the concentration of polysulfide species and the amount of electrolyte in the cell. As sulfur is a conversion type-cathode material, it does not require Li+-ionic electrolyte and the discharge can be performed even with an ammonium solution. In an unconventional way of thinking, Zhang (2013c) found that the introduction of quarternary ammonium into liquid electrolyte could effectively suppress the disproportion of polysulfide intermediate, which increased the Li–S cells capacity retention; however, it is not able to stop the redox shuttle of polysulfide. Very recently, Agostini et al. (2014) exploited the use of non-flammable TEGDME-based electrolyte containing Li2S8 electrolytes. The electrochemical properties of the cell were effectively enhanced by the addition of LiNO3, which provided a stable and protective solid-electrolyte interface on a lithium surface. An excess amount of electrolyte and low loading of sulfur have significantly enhanced the cycle life and capacity retention of Li–S cells (Brückner et al., 2014). However, the energy density of the cell was reduced due to the dead weight of the liquid electrolyte.
Ionic Liquids as Electrolytes
Ionic liquids are identified as an electrolyte system for lithium-ion batteries due to their unique properties such as non-flammability, wide electrochemical stability, non-volatility, high ionic conductivity, and environmental friendliness (Galinski and Lewandowski, 2006). However, the reports on ionic liquids as electrolytes for Li–S batteries are very scanty. Very recently, Watanabe and co-workers reviewed the ionic liquids as electrolytes for lithium–sulfur batteries (Park et al., 2013). The same group also demonstrated the anionic effect as solvate ionic liquid electrolytes in rechargeable Li–S batteries (Ueno et al., 2013). Wang et al. (2008) compared the cycling profile of Li/S cells consisting of 1-ethyl-3-methylimidazolium bis(trifluoromethanesulfonyl) imide and lithium bistrifluoromethanesulfonylimide with a sulfur coated carbon cathode. The cell delivered higher discharge capacity with ionic liquid than the conventional organic solvent electrolyte. The physical and chemical properties of a ternary mixture comprising TEGDME as a polymer solvent in mixed electrolytes composed of N-methyl-n-butyl pyrrolidinium bis(trifluoromethanesulfonyl)imide (PYR14TFSI) and LiTFSI were reported by Shin and Cairns (2008). The ternary compound was employed as electrolyte for Li–S cells. The cell delivered a discharge capacity of 887 and 440 mAh g−1at ambient temperature and at 0°C, respectively. With N-butyl-N-methyl-piperidinium–TFSI as electrolyte solvent and sulfur cathode, Yuan (2006) reported the cycling behavior of Li–S cells. Although, the cells delivered an initial discharge capacity of 1055 mAh g−1 in its first cycle an abrupt decrease in discharge capacity (about 770 mAh g−1) was found after a few cycles. The effect of imidazolium cation on the cycle life of Li–S batteries has been analyzed by Kim et al. (2007). Wang and Byon (2013) designed a new ionic liquid-based organic electrolyte, which swaps solubility and diffusion rate of polysulfides by a combination of 1,2-dimethoxyethane and highly viscous N-methyl-N-propylpiperidinium bis(trifluoromethane sulfonyl) imide (PP13-TFSI). This engineered electrolyte offered high Coulombic efficiency, capacity retention, and suppressed internal shuttling of polysulfides.
Polymer Electrolytes
In the last three decades, extensive research has been devoted on the development of polymer electrolytes as they find applications not only in lithium batteries but also other electrochemical devices such as supercapacitors, fuel cells, and electrochromic devices (Manuel Stephan, 2006). The advantages of polymer electrolytes, their types, advantages, and limitations have already been reported elsewhere (Manuel Stephan et al., 2009; Quartarone and Mustarelli, 2011). In lithium sulfur batteries, polymer electrolytes play a vital role in suppressing the dissolution of poly sulfides in lithium/sulfur cells resulting in good cyclability and enhanced capacity retention (Hassoun and Scrosati, 2010, 2012). Hassoun and Scrosati (2010) demonstrated an all-solid-state lithium/sulfur cells composed of a Li2S/C cathode, lithium metal anode, and a nanocomposite polymer as electrolyte. The cell was capable of delivering a discharge capacity of 300 mAh g−1 at 70°C. The added-ZrO2 filler in a PEO-LiCF3SO3 filler significantly promoted the ionic conductivity of the composite polymeric membrane. Efforts have also been made to replace the highly reactive anode material with alloy anodes with same type of nanocomposite polymer electrolytes (Jeng et al., 2007). Jeddi et al. (2013a,b) proposed a novel polymer electrolyte by blending poly(vinylidene fluoride-co-hexafluoropropene) (PVdF-HFP) with monofunctional poly(methyl methacrylate) (PMMA) containing inorganic trimethoxysilone domains. The gel polymer electrolyte (GPE) was found to be capable of upholding the electrolyte solution and preventing polysulfide diffusion, which improved the cycling performance of the sulfur-based electrode material.
Marmorstein et al. (2000) reported the charging–discharging characteristics of Li/S cells with three different polymer electrolytes at various operating temperatures ranging from ambient temperature to 100°C. Among the systems studied the cell operated between 90 and 100°C with poly ethylene oxide as electrolyte delivered highest discharge capacity. Unfortunately, these systems suffer from poor rate capability.
According to Zhang and Tran (2013d), the selection of electrolyte materials for lithium/sulfur batteries is very limited. Being a very strong nucleophilic agent, the polysulfides can react with many electrolyte solvents such as esters and carbonates and lithium salts such as LiPF6, LiBF4, LiB(C2O4)2, and LiBF2C2O4. Further, elemental sulfur and polysulfides can replace fluorine from fluorinated polymers such as (PVDF-HFP). Eventually, this leads the formation of thiols and vulcanization of unsaturated polymers. In view of the chemical stability, the lithium salts such as LiSO3CF3, LiTFSI, linear or cyclic ethers such as 1,2-dimethyl ether (DME), DIOX as solvents and poly (ethylene oxide), PEO as the ultimate polymer host for polymer electrolyte researchers. The authors also illustrated the influence of GPEs on the cycling performance of Li–S cells (Zhang, 2013b). The authors prepared a composite gel polymer electrolyte (CGPE) composed of PEO and SiO2. Results showed that the incorporation of SiO2 in the polymeric matrix has significantly trapped the polysulfide species in the separator and reduced the utilization of sulfur active materials. The authors also concluded the benefits of GPEs and CGPEs are adversely compensated by sulfur specific capacity. Zhang and Tran (2013d) reported the electrochemical properties lithium/sulfur cell with a flexible and free-standing CGPE membrane composed of 50% PEO–50% SiO2.
Lécuyer et al. (2013) analyzed the structural evolution of Li/S cells based on PEO-based dry polymer electrolytes by employing SEM and EDX. Diffusion of sulfide species resulted in volume changes of both electrode and electrolyte. Attempts have been to enhance the mechanical property of cathode material by incorporation of PVdF in the composite cathode. Although incorporation of PVdF significantly enhanced the mechanical integrity, it could not solve the problems associate with the cyclability.
The cycling profile of Li–S cells with PEO-based gel electrolytes with LiClO4 and TEGDME. The cell delivered higher discharge capacity than the subsequent cycles and a flat discharge has been observed at 2.0 V. The capacity fading has been attributed to low utilization of sulfur, which arises to the aggregation of sulfur (polysulfide) upon cycling (Jeon et al., 2002). The electrochemical and interfacial properties TiO2-laden PEO-LiCF3SO3 complexes have been reported by Shin et al. (2002). The addition of TiO2 into PEO-LiCF3SO3 complexes has substantially promoted not only the ionic conductivity but also interfacial resistance between composite polymer electrolyte and lithium metal anode. The remarkable decrease in the interfacial resistance is attributed to the lowering contact area between lithium and electrolyte. The increased discharge capacity of Li–S cells is attributed to the better interfacial property of composite polymer electrolyte and lithium metal anode.
Superionic Conductors
The appealing properties such as high safety, non-flammability, high thermal stability, reliability, and prevention of polysulfide formation and migration qualify the ionically conducting solids as potential electrolytes for lithium sulfur batteries. Based on their properties they are classified as (i) sulfides, (ii) oxides, and (iii) phosphates (Fergus, 2010). Among the three types, oxides are more stable in air than sulfides (Machida et al., 2004). The search for glass-ceramic materials with high stability in contact with lithium metal combined with a high ionic conductivity has been intensified for the fabrication of all-solid-state lithium batteries in order to ascertain the safety aspects. The major challenge for all solid-state batteries depends mainly on the electrode/electrolyte interfaces (Kim et al., 2006).
Nagao et al. (2011) fabricated an all solid-state lithium sulfur cell comprising sulfur-carbon electrode with Li2S–P2S5 glass–ceramic electrolytes and examined their electrochemical performances. The authors performed the charge-discharge analysis between −20 and 80°C. The cells retained a reversible capacity higher than 850 mAh g−1 for 200 cycles at 25°C. The enhanced discharge capacity of the cell was attributed to the reduced crystallinity of sulfur and particle size of the electrode materials achieved by milling, which facilitated better contacts between the particles.
Hayashi et al. (2003) have successfully introduced a novel all-solid-state Li/S batteries with Li2S–P2S5 as a highly conductive glass–ceramic electrolytes. The cathode materials consisting of sulfur and CuS were synthesized by mechanical milling using sulfur and copper crystals as starting materials. The cell was capable of delivering over 650 mAh g−1 for 20 cycles. The time of ball milling was found to influence the electrochemical properties of Li–S cell.
In a similar way, Kobayashi et al. (2008) reported the cycling performance of an all-solid-state-lithium sulfur battery with sulfur electrode and thio-LISICON electrolyte. The same group also reported the cycling behavior for mesoporous electrode with thio-LISICON electrolyte (Kobayashi et al., 2013).
Electrolyte Additives
Electrolyte additives play an extraordinary role in lithium-ion batteries. Although the amount of additive in the electrolyte is around 5% either by volume or by weight it plays a significant role in the electrochemical performance of lithium-ion batteries. It significantly improves the solid-electrolyte interface on the surface of graphite, reduces irreversible capacity, enhances thermal stability, promotes physical properties of the electrolyte such as ionic conductivity, viscosity, and wettability of the polyolifine membrane (Zhang, 2006). It also protects battery safety by lowering flammability of organic electrolytes, increases tolerance, and stops battery operations in abuse conditions.
In a similar way, the electrolyte additives play a vital role in lithium/sulfur batteries. The polysulfides involve reactions with cathode and anode during cycling and therefore protection of lithium anode from chemical reactions is mandatory. This will significantly eliminate the shuttling of polysulfide and effectively promotes the columbic efficiency and cycling performance. Lithium nitrate (LiNO3) reacts with metallic lithium and forms a rigid passivation layer that prevents the chemical reactions of polysulfides with metallic lithium and is attributed to the decomposition of LiNO3 in the non-aqueous liquid electrolyte (Xiong et al., 2012; Zhang and Read, 2012). Very recently, Lin et al. (2013) illustrated that the addition of P2S5 as additive promoted the dissolution of Li2S and alleviates the loss of capacity caused by the precipitation of Li2S and also eliminated the shuttling of polysulfides by passivating metallic lithium.
Summary and Future Outlook
Li–S batteries are considered as one of the ultimate power source by virtue of its appealing properties such as low cost, non-toxic, abundance, and above all higher discharge capacity (1640 mAh g−1) and energy density (2400 Wh kg−1) than lithium-ion batteries. Regarding the non-aqueous liquid electrolytes for lithium–sulfur batteries, no one system is found to be optimal in all aspects. In this area, more work is certainly needed. Although, extensive research work has been pursued on the development of cathode materials for lithium/sulfur batteries, reports on solid polymer electrolytes and superionic conductors are very scanty. A few reports illustrate the glass-ceramic electrolytes with ionic conductivity exceeding 10−3 Scm−1 at ambient temperature. However, attention should be focused on the major drawbacks such as fragility at low thickness, high cost, and poor interfacial property at electrode/electrolyte interfaces. Prevention of shuttling of polysulfides by suitable polymeric/solid electrolytes will be appreciable and exotic. Ionic liquids in conjunction with polymer electrolytes and sulfurized carbon electrodes may offer a safe, reliable, and polysulfide shuttle-free lithium–sulfur batteries.
Conflict of Interest Statement
The authors declare that the research was conducted in the absence of any commercial or financial relationships that could be construed as a potential conflict of interest. The Review Editor Jijeesh Ravi Nair declares that, despite having collaborated with author Natarajan Angulakshmi, the review process was handled objectively and no conflict of interest exists.
Abbreviations
AFM, atomic force microscope; CGPE, composite gel polymer electrolyte; DEC, diethyle carbonate; DIOX, 1,3-dioxolane; DMAc, dimethyl acetamide; DME, dimethyl ether; DMSO, dimethyl sulfoxide; EC, ethylene carbonate; EDX, energy dispersive X-ray; EMS, ethyl methane sulfonate; GPE, gel polymer electrolyte; Li2S, lithium sulfide; LiB(C2O4)2, lithium bis(oxalate)borate (LiBOB); LiBF2C2O4, lithium bid(difluoro oxalate)borate (LiDFOB); LiBF4, lithium tetrafluoroborate; LiClO4, lithium perchlorate; LiCF3SO3, lithium trifluoromethanesulfonate; LiPF6, lithium hexafluorophosphate; LiTFSI, lithium bis(trifluoromethylsulfonyl)imide; P2S5, phosphorous pentasulfide; PC, propylene carbonate; PEO, poly(ethylene oxide); PMMA, polymethylmethacrylate; PVDF, poly(vinylidene fluoride); PVDF-HFP, poly(vinylidene fluoride-co-hexafluoropropene); PYR14TFSI, N-methyl-n-butyl-pyrrolidinium-bis(trifluoromethylsulfonyl)imide; SEM, scanning electron microscope; TEGDME, tetra (ethylene glycol) dimethyl ether; TEOS, triethoxysilane; THF, tetrahydrofuran; XRD, X-ray diffraction.
References
Abraham, K. M., Rauh, R. D., and Brummer, S. B. (1978). A low temperature Na-S battery incorporating a soluble sulfur cathode. Electrochim. Acta 23, 501–507. doi: 10.1016/0013-4686(78)85027-0
Agostini, M., Lee, D.-J., Scrosati, B., Sun, Y. K., and Hassoun, J. (2014). Characteristics of Li2S8-tetraglyme catholyte in a semi-liquid lithium-sulfur battery. J. Power Sources 265, 14–19. doi:10.1016/j/jpowsour.2014.04.074
Akridge, J. R., Mikhaylik, Y. V., and White, N. (2004). Li/S fundamental chemistry and application to high performance rechargeable batteries. Solid State Ionics. 175, 243–245. doi:10.1016/j.ssi.2004.07.070
Arico, A. S., Bruce, P. G., Scrosati, B., Tarascon, J.-M., and Schalkwijk, M. V. (2005). Nanostructured materials for advanced energy conversion and storage devices. Nat. Mater. 4, 366–377. doi:10.1038/nmat1368
Aurbach, D., Pollak, E., Elazari, R., Salitra, G., Kelley, C. S., and Affinito, J. (2009). On the surface chemical aspects of very high energy density, rechargeable Li-sulfur batteries. J. Electrochem. Soc. 156, A694–A702. doi:10.1149/1.3148721
Barchasz, C., Leprêtre, J. C., Alloin, F., and Patoux, S. (2012). New insight into the limiting parameters of the Li/S rechargeable cell. J. Power Sources 199, 322–330. doi:10.1016/j.jpowsour.2011.07.021
Barchasz, C., Leprêtre, J.-C., Patoux, S., and Alloin, F. (2013a). Electrochemical properties of ether-based electrolytes for lithium/sulfur rechargeable batteries. Electrochim. Acta 89, 737–743. doi:10.1016/j.electacta.2012.11.001
Barchasz, C., Leprêtre, J.-C., Patoux, S., and Alloin, F. (2013b). Revisiting TEGDME/DIOX binary electrolytes for lithium/sulfur batteries: importance of solvation ability and additives. J. Electrochem. Soc. 160, A430–A436. doi:10.1149/2.022303jes
Barghamadi, M., Best, A. S., Bhatt, A. I., Hollenkamp, A. F., Musameh, M., Ress, R. J., et al. (2014). Lithium-sulfur batteries – the solution is in the electrolyte, but is the electrolyte a solution? Energy Environ. Sci. 7, 3902–3920. doi:10.1039/C4EE02192D
Barghamadi, M., Kapoor, A., and Wen, C. (2013). A review on Li-S batteries as a high efficiency rechargeable lithium battery. J. Electrochem. Soc. 160, A1256–A1263. doi:10.1149/2.096308jes
Bock, D. C., Marchilok, A. C., Takeuchi, K. J., and Takeuchi, E. S. (2012). Batteries used to implantable biomedical devices. Electrochim. Acta 84, 155–164. doi:10.1016/j.electacta.2012.03.057
Bresser, D., Passerini, S., and Scrosati, B. (2013). Recent progress and remaining challenges in sulphur-based lithium secondary batteries – a review. Chem. Commun. (Camb.) 49, 10545–10562. doi:10.1039/c3cc46131a
Bruce, P. G., Freunberger, S. A., Hardwick, L. J., and Tarascon, J. M. (2012). Li-O2 and Li-S batteries with high energy storage. Nat. Mater. 11, 19–29. doi:10.1038/nmat3191
Bruce, P. G., Scrosati, B., and Tarascon, J. M. (2008). Nanomaterials for rechargeable lithium batteries. Angew. Chem. Int. Ed. Engl. 47, 2930–2946. doi:10.1002/anie.200702505
Brückner, J., Soren Thieme, S., Grossmann, H. T., Deorfler, S., Althues, H., and Kaskel, S. (2014). Lithium-sulfur batteries: influence of C-rate, amount of electrolyte and sulfur loading on cycle performance. J. Power Sources 268, 82–87. doi:10.1016/j/jpowsour.2014.05.143
Brummer, S. B., Rauh, R. D., Msrston, J. M., and Shuker, F. S. (1976). Low Temperature Alkali Metal-S Batteries. Annu Prog Rep. U.S. ERDA Contract No.Ey-76-c-02-2520.
Cakan, R., Morcrette, M., and Tarascon, J.-M. (2013). Li-S batteries: simple approaches for superior performance. Energy Environ. Sci. 6, 176–182. doi:10.1039/C2EE23411D
Canas, N. A., Wolf, S., Wagner, N., and Friedrich, K. A. (2013a). In-situ X-ray diffraction studies of lithium-sulfur batteries. J. Power Sources 226, 313–319. doi:10.1016/j.jpowsour.2012.10.092
Canas, N. A., Hirose, K., Pascucci, B., Wagner, N., Friedrich, K. A., and Hiesgen, R. (2013b). Investigations of lithium-sulfur batteries using impedance spectroscopy. Electrochim. Acta 97, 42–51. doi:10.1016/j.electacta.2013.02.101
Chang, D. R., Lee, S. H., Kim, S. W., and Kim, H. T. (2002). Binary electrolyte based on tetra(ethylene glycol) dimethyl ether and 1,3-dioxolane for lithium-sulfur battery. J. Power Sources 112, 452–460. doi:10.1016/S0378-7753(02)00418-4
Chen, S., Dai, F., Gordin, M. L., and Wang, D. (2013). Exceptional electrochemical performance of rechargeable lithium-sulfur batteries with a poysulfide containing electrolyte. RSC Adv. 3, 3540–3543. doi:10.1039/C3RA23070H
Cheon, S. E., Ko, K. S., Cho, J. S., Kim, S. W., Chin, E. Y., and Kim, H. T. (2003a). Rechargeable lithium sulfur battery: 1. Structural change of sulfur cathode during discharge and charge. J. Electrochem. Soc. 150, A796–A799. doi:10.1149/1.1571532
Cheon, S. E., Ko, K. S., Cho, J. S., Kim, S. W., Chin, E. Y., and Kim, H. T. (2003b). Rechargeable lithium sulfur battery: 2. Rate capability and cycle characteristics. J. Electrochem. Soc. 150, A800–A805. doi:10.1149/1.1571533
Choi, J. W., Cheruvally, G., Kim, D. S., Ahn, J. H., Kim, K. W., and Ahn, H. J. (2008). Rechargeable lithium/sulfur battery with liquid electrolytes containing toluene as additive. J. Power Sources 183, 441–445. doi:10.1016/j.jpowsour.2008.05.038
Chu, M. Y., PeJonghe, L. C., Visco, S. J., and Katy, B. D. (2000). Liquid Electrolyte Lithium Sulphur Batteries. US Patent 08/948,969.
Diao, Y., Xie, K., Xiong, S., and Hong, X. (2013). Shuttle phenonmenon: the irreversible oxidation mechanism of sulphur active material in Li-S battery. J. Power Sources 235, 181–186. doi:10.1016/j.jpowsour.2013.01.132
Elazari, R., Salitra, G., Talyosef, Y., Grinblat, J., Kelley, S. C., Xiao, A., et al. (2010). Morphological and structural studies of composite sulfur electrodes upon cycling by HRTEM, AFM and Raman spectroscopy. J. Electrochem. Soc. 157, A1131–A1138. doi:10.1149/1.3479828
Fergus, J. W. (2010). Ceramic and polymeric solid electrolytes for lithium-ion batteries. J. Power Sources 195, 4554–4569. doi:10.1016/j.jpowsour.2010.01.076
Fu, Y., Su, Y.-S., and Manthiram, A. (2012). Sulfur-carbon nanocomposite cathodes improved by anamphiphilic block copolymer for high rate lithium-sulfur batteries. ACS Appl. Mater. Interfaces 4, 6046–6052. doi:10.1021/am301688h
Galinski, M., and Lewandowski, A. (2006). Ionic liquids as electrolytes. Electrochim. Acta 51, 5567–5580. doi:10.1016/j.electacta.2006.03.016
Gao, J., Lowe, M. A., Kiya, Y., and Abruna, H. D. (2011). Effects of liquid electrolytes on the charge-discharge performance of rechargeable Li/S batteries: electrochemical and in-situ X-ray absorption spectroscopic studies. J. Phys. Chem. C 115, 25132–25137. doi:10.1021/jp207714c
Goodenough, J. B., and Kim, Y. S. (2010). Challenges for rechargeable lithium batteries. Chem. Mater. 22, 587–603. doi:10.1021/cm901452z
Hagen, M., Schiffels, P., Hammer, M., Dörfler, S., Tübke, J., Hoffmann, M. J., et al. (2013). In-situ Raman investigation of polysulfide formation in Li-S cells. J. Electrochem. Soc. 160, A1205–A1214. doi:10.1149/2.045308jes
Hassoun, J., and Scrosati, B. (2010). A high performance polymer tin sulfur lithium-ion battery. Angew. Chem. Int. Ed. 49, 2371–2374. doi:10.1002/anie.200907324
Hassoun, J., and Scrosati, B. (2012). Moving to a solid-state configuration: a valid approach to making Li-S batteries viable for practical applications. Adv. Mater. 22, 5198–5201. doi:10.1002/adma.201002584
Hayashi, A., Ohtomo, T., Mizuno, F., Tadanaga, K., and Tatsumisago, M. (2003). All solid-state Li/S batteries with highly conductive glass-ceramic electrolytes. Electrochem. commun. 5, 701–705. doi:10.1016/S1388-2481(03)00167-X
Jeddi, K., Ghaznavi, M., and Chen, P. (2013a). A novel polymer electrolyte to improve the cycle life of high performance Li-sulfur batteries. J. Mater. Chem. A 1, 2769–2772. doi:10.1039/C3TA01169K
Jeddi, K., Zhao, Y., Zhang, Y., Kunarov, A., and Chen, P. (2013b). Fabrication and characterization of and effective nanocomposite electrolyte membrane for high performance lithium/sulfur batteries. J. Electrochem. Soc. 160, A1052–A1060. doi:10.1149/2.010308jes
Jeng, S. S., Lim, Y. T., Choi, Y. J., Cho, G. B., Kim, K. W., Ahn, H. J., et al. (2007). Electrochemical properties of lithium sulfur cells using PEO polymer electrolytes prepared under three different mixing conditions. J. Power Sources 174, 745–750. doi:10.1016/j.jpowsour.2007.06.108
Jeon, B. H., Yeon, J. H., Kim, K. M., and Chung, I. J. (2002). Preparation and electrochemical properties of lithium-sulfur polymer batteries. J. Power Sources 109, 89–97. doi:10.1016/S0378-7753(02)00050-2
Ji, X., Lee, K. T., and Nazar, L. F. (2009). A highly ordered nanostructured carbon-sulfur cathode for lithium-sulfur batteries. Nat. Mater. 8, 500–506. doi:10.1038/nmat2460
Ji, X., and Nazar, L. (2010). Advances in lithium-sulfur batteries. J. Mater. Chem. 20, 9821–9826. doi:10.1039/b925751a
Kim, S., Jung, Y., and Park, S. J. (2007). Effect of imidazoliumcation on cycle life characteristics of secondary lithium-sulfur cells using liquid electrolytes. Electrochim. Acta 52, 2116–2122. doi:10.1016/j.electacta.2006.08.028
Kim, Y., Saienga, J., and Martin, S. W. (2006). Anamalous ionic conductivity increase in Li2S+GeS2+GeO2 glass. J. Phys. Chem. B 110, 16318–16325. doi:10.1021/jp060670c
Kobayashi, T., Imade, Y., Shishihara, D., Homma, K., Nagao, M., Watanabe, R., et al. (2008). All solid-state battery with sulphur electrode and thio-LISICON electrolyte. J. Power Sources 182, 621–625. doi:10.1016/j.jpowsour.2008.03.030
Kobayashi, T., Watanabe, R., Yokoi, T., Tatsumi, T., Kanno, R., Nagao, M., et al. (2013). All-solid-state Li/sulfur batteries with mesoporous electrode and thio-LISICON solid electrolyte. J. Power Sources 222, 237–242. doi:10.1016/j.jpowsour.2012.08.041
Kock, V. R., and Young, J. H. (1977). The Electrochemical Society Fall Meeting. Atlanta, GA: Electrochemical Society.
Kolosnitsyn, V. S., and Karaseva, E. V. (2008). Lithium-sulfur batteries: problems and solutions. Russ. J. Electrochem. 44, 506–509. doi:10.1134/S1023193508050029
Kolosnitsyn, V. S., Kuz’mina, E. V., Karaseva, E. V., and Michalov, S. E. (2011). Impedance spectroscopy studies of changes in the properties of lithium sulfur cells in the course of cycling. Russ. J. Electrochem. 47, 793–798. doi:10.1134/S1023193511070093
Lécuyer, M., Gaubicher, J., Deschamps, M., Lestriez, B., Brousse, T., and Guyomard, D. (2013). Structural changes of a Li/S rechargeable cell in lithium metal polymer technology. J. Power Sources 241, 249–254. doi:10.1016/j.jpowsour.2013.04.119
Lee, J. S., Kim, S. T., Cao, R., Choi, N. S., Liu, M., Lee, K. T., et al. (2011). Metal-air batteries with high energy density: Li-air versus Zn-air. Adv. Energy Mater. 1, 34–50. doi:10.1002/aenm.201000010
Liang, C. D., Dudney, N. J., and Howe, J. Y. (2009). Hierarchically structured sulfur/carbon nanocomposite materials for high energy lithium battery. Chem. Mater. 21, 4724–4730. doi:10.1021/cm902050j
Lin, Z., Liu, Z., Fu, W., Dudney, N. J., and Liang, C. (2013). Phosphorous pentasulfide as a novel additive for high performance lithium-sulfur batteries. Adv. Funct. Mater. 23, 1064–1069. doi:10.1002/adfm.201200696
Machida, N., Yoneda, Y., and Shigematsu, T. (2004). Mechano-chemical synthesis of lithium ion conducting materials in the system Li2O-Li2S-P2S5. J. Jpn. Soc. Powder Powder Metall. 51, 2. doi:10.2497/jjspm.51.91
Manthiram, A., Fu, Y., and Su, Y. S. (2013). Challenges and prospects of lithium-sulfur batteries. Acc. Chem. Res. 46, 1125–1134. doi:10.1021/ar300179v
Manuel Stephan, A. (2006). Review on gel polymer electrolytes for lithium batteries. Eur. Polym. J. 42, 21–42. doi:10.1016/j.eurpolymj.2005.09.017
Manuel Stephan, A., Kumar, T. P., Nathan, M. A. K., and Angulakshmi, N. (2009). Chitin-incorporated poly(ethylene oxide)-based nanocomposite electrolytes for lithium batteries. J. Phys. Chem. B 113, 1963–1972. doi:10.1021/jp808640j
Marmorstein, D., Yu, T. H., Striebel, K. A., McLarnon, F. R., Hou, J., and Cairns, E. J. (2000). Electrochemical performance of lithium/sulfur cells with three different polymer electrolytes. J. Power Sources 89, 219–226. doi:10.1016/S0378-7753(00)00432-8
Mikhaylik, Y., Kovalev, I., Scholck, R., Kumaresan, K., Xu, J., and Affinito, J. (2010). High energy rechargeable Li-S cells for EV application: status, remaining problems and solutions. ECS Trans. 25, 23–25. doi:10.1149/1.3414001
Mikhaylik, Y. V. (2008). Lithium Sulfur Electrochemical Cell Including Insoluble Nitrogen-Containing Compound. US 2008/0193835 A1
Mikhaylik, Y. V., and Akridge, J. R. (2004). Polysulfide shuttle study in the Li/S battery system. J. Electrochem. Soc. 151, A1969–A1976. doi:10.1021/ja508982p
Nagao, M., Hayashi, M., and Tatsumisago, T. (2011). Sulfur/carbon composite electrode for allsolid-state lithium/sulfur battery with Li2S-P2S5 solid electrolytes. Electrochim. Acta 56, 6055–6059. doi:10.1016/j.electacta.2011.04.084
Nazar, L. F., and Evers, S. (2013). New approaches for high energy density lithium sulfur battery cathodes. Acc. Chem. Res. 46, 1135–1143. doi:10.1021/ar3001348
Park, J.-W., Ueno, K., Tachikawa, N., Dokko, K., and Watanabe, M. (2013). Ionic liquid electrolytes for lithium-sulfur batteries. J. Phys. Chem. C 117, 20531–20541. doi:10.1002/cssc.201402800
Peled, E., Sternberg, Y., Gorenstein, A., and Lavi, Y. (1989). Lithium-sulfur battery: evaluation of dioxolane-based electrolytes. J. Electrochem. Soc. 136, 1621–1625. doi:10.1149/1.2096981
Quartarone, E., and Mustarelli, P. (2011). Electrolytes for solid-state lithium rechargeable batteries: recent advances and perspectives. Chem. Soc. Rev. 40, 2525–2540. doi:10.1039/C0CS00081G
Rauh, R. D., Abraham, K. M., Pearson, G. F., Suprenant, J. K., and Brummer, S. B. (1979). A lithium/dissolved sulfur battery with an organic electrolyte. J. Electrochem. Soc. 126, 523–527. doi:10.1149/1.2129079
Rauh, R. D., Shuker, F. S., Marston, J. M., and Brummer, S. B. (1977). Formation of lithium polysulfides in aprotic media. J. Inorg. Nucl. Chem. 39, 1761–1766. doi:10.1016/0022-1902(77)80198-X
Rhu, H. S., Ahn, H.-J., Kim, K.-W., Ahn, J.-H., Cho, K. K., Nam, T. H., et al. (2006). Discharge behavior of lithium/sulfur with TEGDME based electrolytes at low temperature. J. Power Sources 163, 201–206. doi:10.1016/j.jpowsour.2005.12.061
Santoni, F., Tortora, P., Alessandrini, F., and Passerini, S. (2002). Proceedings of the Sixth European Space Power Conference (ESPC) 6–10 May 2002, Porto, Portugal. European Space Agency, 653–658.
Schalkwijk, V. K., and Scrosati, B. (2002). Advances in lithium-Ion Batteries. New York, NY: Kluwer Academic/Plenum.
Scrosati, B. (2011). Review of bottled lightning: super batteries, electric cars, and the new lithium economy Seth Fletcher. Nature 473, 448–449. doi:10.1038/473448a
Shim, J., Striebel, K., and Cairns, E. J. (2002). The lithium/sulfur rechargeable cell: effects of electrode composition and solvent on cell performance. J. Electrochem. Soc. 149, A1321–A1325. doi:10.1149/1.1503076
Shin, J. H., and Cairns, E. J. (2008). N-methyl-N-butylpyrrolidiniumBis (trifluoromethanesulfonyl)imide-LiTFSI-tetraethylene glycol dimethyl ether mixture as a lithium cell electrolyte. J. Electrochem. Soc. 155, A368–A373. doi:10.1149/1.2869876
Shin, J. H., Kim, K. W., and Ahn, H. J. (2002). Electrochemical properties and interfacial stability of(PEO)10LiCF3SO3-/TinO2n-1 composite polymer electrolytes forlithium/sulfur battery. Mater. Sci. Eng. B 95, 148–156. doi:10.1016/S0921-5107(02)00226-X
Tachikawa, N., Yamauchi, K., Takashima, E., Park, J. W., Dokko, K., and Watanabe, M. (2011). Reversibility of electrochemical reactions of sulfur supported on inverse opal carbon in glyme-Li salt molten complex electrolytes. Chem. Commun. 47, 8157–8159. doi:10.1039/C1CC12415C
Tarascon, J.-M. (2001). Issues and challenges facing rechargeable Lithium batteries. Nature 414, 359–367. doi:10.1038/35104644
Ueno, K., Park, J. W., Yamazaki, A., Mandai, T., Tachikawa, N., Dokko, K., et al. (2013). Anionic effect as solvate ionic liquid electrolytes in rechargeable lithium/sulfur batteries. J. Phys. Chem. C 117, 20509–20516. doi:10.1021/jp407158y
Wang, J., Chew, S. Y., Zhao, Z. W., Ashraf, S., Wexler, D., Chen, J., et al. (2008). Sulfur-mesoporous carbon composites in conjunction with a novel ionic liquid electrolyte for lithium rechargeable batteries. Carbon N. Y. 46, 229–235. doi:10.1016/j.carbon.2007.11.007
Wang, L., and Byon, H. R. (2013). N-methyl-N-propylpiperidiniumbis (trifluoromethanesulfonyl)imide-based organic electrolyte for high performance lithiumesulfur batteries. J. Power Sources 236, 207–214. doi:10.1016/j.jpowsour.2013.02.068
Wang, W., Wang, Y., Huang, Y., Huang, C., Yu, Z., Zhang, H., et al. (2010). The electrochemical performance of Li-sulfur batteries with LiClO4, DOL/DME electrolyte. J. Appl. Electrochem. 40, 321–326. doi:10.1007/s10800-009-9978-z
Whittingham, M. S. (2004). Lithium batteries and cathode materials. Chem. Rev. 104, 4271–4302. doi:10.1021/cr020731c
Xia, Y., Xin, S., Guo, Y.-G., and Wan, L.-J. (2013). Li-S batteries: electrochemistry, materials and prospects. Angew. Chem. Int. Ed. Engl. 52, 13186–13200. doi:10.1002/anie.201304762
Xiong, S., Xie, K., Diao, Y., and Hong, X. (2012). Properties of surface film on lithium anode with LiNO3 as lithium salt in electrolyte solution for Li/S batteries. Electrochim. Acta 83, 78–86. doi:10.1016/j.electacta.2012.07.118
Yamin, H., and Peled, E. (1983). Electrochemistry of a non-aqueous lithium/sulfur cells. J. Power Sources 9, 281–287. doi:10.1016/0378-7753(83)87029-3
Yang, Y., Yu, G., Cha, J. J., Wu, H., Vosgueritchian, M., Yao, Y., et al. (2011). Improving the performance of lithium-sulfur batteries by conductive polymer coating. ACS Nano 5, 9187–9193. doi:10.1021/nn203436j
Yeon, J.-T., Jang, J.-Y., Han, J.-G., Cho, J., Lee, K. T., and Choi, N.-S. (2012). Raman spectroscopic and X-ray diffraction studies of sulfur composite electrodes during discharge and charge. J. Electrochem. Soc. 159, A1308–A1314. doi:10.1149/2.080208jes
Yuan, L., Yuan, H., Qiu, X., Chen, L., and Zhu, W. (2009). Improvement of cycle property of sulphur-coated multi-walled carbon nanotubes composite cathode for lithium sulphur batteries. J. Power Sources 189, 1141–1146. doi:10.1016/j.jpowsour.2008.12.149
Yuan, L. X. (2006). Improved dischargeability and reversibility of sulfur cathode in a novel ionic liquid electrolyte. Electrochem. Commun. 8, 610–614. doi:10.1016/j.elecom.2006.02.007
Zhang, S. S. (2006). A review on electrolyte additives for lithium-ion batteries. J. Power Sources 162, 1379–1394. doi:10.1016/j.jpowsour.2006.07.074
Zhang, S. S. (2013a). Liquid electrolyte lithium/sulfur battery: fundamental chemistry, problems and solutions. J. Power Sources 231, 153–162. doi:10.1016/j.jpowsour.2012.12.102
Zhang, S. S. (2013b). A concept for making PEO based composite gel polymer electrolyte lithium/sulfur battery. J. Electrochem. Soc. 160, A1421–A1424. doi:10.1149/2.058309jes
Zhang, S. S. (2013c). New insight into liquid electrolyte of rechargeable lithium/sulfur battery. Electrochim. Acta 97, 226–230. doi:10.1016/j.electacta.2013.02.122
Zhang, S. S., and Read, J. A. (2012). A new direction for the performance improved of rechargeable lithium/sulfur batteries. J. Power Sources 200, 77–82. doi:10.1016/j.jpowsour.2011.10.076
Keywords: lithium–sulfur batteries, poly sulfides, polymer electrolytes, charge–discharge studies, interfacial properties, ionic liquids, super ionic conductors, electrolyte additives
Citation: Angulakshmi N and Stephan AM (2015) Efficient electrolytes for lithium–sulfur batteries. Front. Energy Res. 3:17. doi: 10.3389/fenrg.2015.00017
Received: 15 July 2014; Accepted: 26 March 2015;
Published online: 21 May 2015.
Edited by:
Mariusz Walkowiak, Institute of Non-Ferrous Metals, PolandReviewed by:
Xiqing Wang, Nanotek Instruments Inc., USAJijeesh Ravi Nair, Politecnico di Torino, Italy
Mariusz Walkowiak, Institute of Non-Ferrous Metals, Poland
Copyright: © 2015 Angulakshmi and Stephan. This is an open-access article distributed under the terms of the Creative Commons Attribution License (CC BY). The use, distribution or reproduction in other forums is permitted, provided the original author(s) or licensor are credited and that the original publication in this journal is cited, in accordance with accepted academic practice. No use, distribution or reproduction is permitted which does not comply with these terms.
*Correspondence: Arul Manuel Stephan, Central Electrochemical Research Institute (CSIR-CECRI), Karaikudi 630 006, India e-mail: arulmanuel@gmail.com; amstephan@cecri.res.in