- Faculty of Science and Technology, Athabasca University, Athabasca, AB, Canada
Long-term economic and environmental concerns have resulted in a great amount of research on renewable sources of biomass and bioenergy to replace fossil fuels in the past decades. Decentralized biogas technology is one of the most potential technologies of biomass and bioenergy by using agricultural waste materials (e.g., animal manure, crop straw, and by-products from food industries) as feedstocks. By-products from biogas production, called digestate, are nutrient rich, which could potentially be reused as green fertilizers in agriculture, thereby providing a sustainable substitute for synthetic fertilizers for farm ecosystem. Thus, the biogas production of anaerobic digestion is win–win option for livestock and crop producers to address issues of waste management and energy supply, and to avoid contamination of surface and ground waters and emissions of odors and greenhouse gases. In this paper, we review biogas production technology and then evaluate environmental effects of digestate used as fertilizer. Finally, we discuss issues of deployment of decentralized biogas technology for farm ecosystem. Economic and technological barriers still exist for large scale deployment of biogas technology in rural region. Two national scale deployments in China and Nepal showed that the operational status of biogas digesters is not optimal and up to 50% of plants are non-functional after a short operation period regardless of the social and economic factors. Main barriers are a wide variation of feedstocks and environmental conditions (e.g., temperature) over space and time. It becomes clear that the experimental conditions of the pilot plants need to be adjusted and calibrated to the local feedstocks and climate. Also, more research needs to be done in cold fermentation technology. Thus, collaboration of all relevant designers, farmers, stakeholders, and regulators is proposed as the way forward, particularly as their complexity has been identified as the major hurdle to the implementation of decentralized biogas production, which can deliver beneficial synergies for the decentralized biogas production in rural region responsible for food production and waste management.
Introduction
Biogas energy can make a major contribution to climate protection and resource conservation, regardless of whether wastes or specially cultivated crops are used as feedstocks. There are lots of biomass and bioenergy technologies (Chung, 2013, 2014; Tonini et al., 2013). Biogas production using anaerobic digestion (AD) is one of the most potential technologies in bioenergy portfolios. AD biogas can be produced of nearly all kinds of organic materials and wastes from agriculture and food industry.
The UK generates some 30 million dry tonnes of this waste material a year, capable of producing some 6.3 million tonnes of oil equivalent of methane (CH4) gas (NSCA, 2006). It has been estimated that only 68% of the weight of a chicken, 62% of a pig, 54% of a bovine animal, and 52% of a sheep or goat are directly consumed (Swisher, 2006). This means that, every year, 34 ~ 44% of the weight of livestock are waste (millions of tons of animal by-products are produced worldwide). In Canada, the energy potential of the five sources of biogas energy (agricultural, landfill gas, municipal/residential organics, organics from commercial source, and wastewater residual) is estimated at 810 MW or 2420 Mm3/year of renewable natural gas in which the contribution of agriculture is 68% (550 MW or 1650 Mm3/year) (Biogas Association, 2013). The production of Canadian livestock manure increased by 16% (an estimated 25 million tonnes) from 156 million tonnes in 1981 to 181 million tonnes in 2006 (Hofmann, 2006). Canadian cattle population raised just over 12.5 million cattle in January 1, 2012, up 0.5% from the same date a year earlier; the first year-over-year increase in 7 years (Statistics Canada, 2012). The potential biogas recovery estimated for 8200 U.S. dairy and swine operations is more than 13 million megawatt-hours (MWh) per year, replacing about 1670 MW of fossil fuel-fired generation (EPA, 2012).
The rate of biogas production depends on the rate of removed soluble chemical oxygen demand (sCOD) and the temperature, which is also related to the dry matter (DM) or organic dry matter (ODM) of the feedstock material. In human feces, organic matter makes up to 86% of DM. Depending on the digester type, retention time and biodegradability, about 40–90% of organic matter could be converted to biogas (Mang and Li, 2010). Thus, there are rich waste resources for AD biogas production without competition of land use with food crop production. These significant feedstock resources are available for the production of biogas in the worldwide allowing us both to manage a waste issue and to provide a source of renewable energy.
There are two basic biogas technologies: centralized and decentralized plant. A centralized biogas plant is large scale for the treatment of waste and biomass. This technology has advantages due to its large scale. Waste can be pretreated, processes can be adjusted and operators can be trained. However, due to low energy density of biomass and waste, there is an issue of a centralized biogas plant for large size energy production, for which huge raw feedstocks needs to be transported and stored. The feedstock supply network needs guarantee under long-term contracts. Thus, energy production using AD may be cost except for those sites with availability of local feedstocks, such as livestock farms. Huopana et al. (2013) investigated sustainable biogas electricity production in a Finnish province. They showed that the biogas production potential is marginal from biodegradable waste of the energy consumption (e.g., transportation). Manure should be considered as a raw material for waste management rather than those for biogas production due to negative contribution to the net energy balance between the biogas production and the energy consumption of manure transportation. In the UK, a life cycle assessment of biogas infrastructure was carried out by Patterson et al. (2011) for centralized and decentralized options in a regional scale. An 80% heat utilization had the least environmental impact, followed by transport fuel use. A 32% difference of transportation between centralized and decentralized options had a relatively small effect on the overall environmental impact. For treatment of sewage sludge, AD has advantage for the main objectives of the degradation and destruction of organic feedstocks, with consequent sludge stabilization and pathogen reduction since sewage sludge supply is stable (Aiyuk et al., 2006; Tomei et al., 2009; Abe et al., 2011). A decentralized biogas plant is small size which may be more attractive since raw feedstocks are available in certain distance for small farm scale. This is very suitable for small farm without the key issue of transport and storage. Therefore, a decentralized AD plant of biogas production has clear advantages on islands, farm, and even rural region on the mainland – far away from power sources, where the cost of transporting the power (electricity, gas, diesel, propane) is better spent building smaller local plants to produce energy to off-set them.
It is well-known that farmers routinely used manure to complement a good fertility program due to its rich nitrogen (N) and phosphorus (P), which are important nutrients for crop growth. Manure is also a source of organic matter and can increase porosity of soils, which can help improve soil’s water-holding capacity and reduce soil erosion. However, manure can also become a source of pollution leading impacts on the environment and human health (Petersen et al., 2007). For instance, bacteria found in manure have been found both in municipal and private drinking water supplies (Hofmann and Beaulieu, 2001). Excessive buildup of phosphorus-based nutrients in farmland where too much manure is applied over time is also a problem of watershed protection (Manitoba Government, 2014). An over-enrichment concentration of phosphorus and other nutrients in surface water is called eutrophication. The eutrophication is a widespread problem for aquatic life in rivers and lakes due to growth and blooms of algae. Blooms of some algae may release toxins to a harmful level into surface water for wildlife, livestock, and humans if they drink the water. Manure can also be a source of nuisance odor. Today, a major challenge in manure management is the sheer volume and health risks posed by raw manure because of increasing emphasis on manure management to protect water quality, and the need to safely dispose of this resource as it accumulates. Furthermore, because of rising costs of commercial fertilizers and demands of organic foods, renewed interest has been focused on maximizing the fertilizer returns of organic manures. Imagine if the pathogen, bacteria, and odor could be removed from livestock manure, the remaining solids or liquid are converted to pathogen-free digestate as fertilizer (Johansen et al., 2013). Biogas energy presents not only opportunities for farmers who can enhance the value of their agricultural waste (animal manure and crop residues) through biogas production and digestate used as fertilizer but also benefits for the climate change through reduction of greenhouse gas emission and odor emissions. Therefore, biogas has the potential to offering cleaner, more-efficient alternatives to the fossil energy for our heat and electricity demand and it will be an important part of the comprehensive and balanced technology portfolio needed to address two most important challenges of food production – significantly reducing carbon and odor emissions and providing organic foods (Zheng et al., 2010).
A green farm ecosystem is an integrated system of AD biogas plant for waste treatment and digestate organic fertilizer for crop growth (Figure 1). AD biogas production plays a pivotal role in such a farm ecosystem. In the AD biogas production, the waste biomass is fed into a sealed tank called digester, where biomass is heated and agitated. In the absence of oxygen, anaerobic microorganisms consume the biomass to multiply and produce biogas. AD captures CH4 and carbon dioxide (CO2) from the manure and food by-products, reducing the greenhouse gas emissions (GHGs) in agricultural nutrient management. The by-products from the digester are known as digestate. AD treatment can reduce the pathogen, bacteria, and odors levels in the raw manure and food waste by up to 99%. Schievano et al. (2011) carried out an on-field calculation of mass, carbon, and nutrients balance at full-scale plants to improve AD performance and evaluate efficiency. AD digestate are nutrient rich, which could potentially be reused as green fertilizers in farms, thereby providing a sustainable substitute for synthetic fertilizers (Wang et al., 2011; Vaneeckhaute et al., 2013) and recycling nutrients for crop production to off-set the use of synthetic fertilizers. Searchinger et al. (2008) confirmed the AD value using waste products. Livestock producers can address waste management issues and avoid contamination of surface and ground waters, and emissions of odors, ammonia, and carbon greenhouse gases. In a short word, biogas production is multi-win option for current energy, climate change, water and food security strategy in advanced farm management.
Anaerobic Digestion
There are lots of publications on principle and processes of AD, which are taken as matured technologies during AD deployment. First of all, let’s revisit major factors of AD processes in use of these matured technologies.
Processes of Anaerobic Digestion
Anaerobic digestion is a collection of processes by which microorganisms break down biodegradable material in the absence of oxygen. There are three basic AD processes: psychrophilic, mesophilic, and thermophilic (Bisschops et al., 2009), which take place over different temperature ranges. Psychrophilic digestion is a low temperature (<20°C) processes. Mesophilic digestion takes place between 20 and 45°C, which can take a month or two to complete, and thermophilic digestion between 45 and 65°C, which is faster, but its microorganisms are more sensitive. AD processes includes four steps (Demirbas and Balat, 2009): hydrolysis, fermentation, acetogenesis, and methanogenesis, as shown in Figure 2. In the hydrolysis step, the feedstocks of insoluble large polymers are broken down into soluble substrates (e.g., sugar and amino acids) by enzymes. Fermentations of the monomeric products are most important in which sugar, amino acids, and fatty acids are converted into ammonia, organic acids, hydrogen (H2), and CO2. Volatile fatty acids are also produced along with CO2 and H2. In acetogenesis step, volatile fatty acids are broken down into acetic acids, CO2 and H2. Finally, methanogenesis step converts acetate, formaldehyde, and H2 to CH4 and water. Thus, the AD consumes carbon of waste to produce biogas (CH4, H2, and CO2) and digestate.
Operating Conditions of Anaerobic Digestion
In Figure 2, there are many products, by-products and intermediate products produced during the digestion process of feedstocks to the final product (CH4 and CO2). Microorganisms break down biomass materials in anaerobic conditions and produce CH4 and other gases in the process of completing their life cycle. There are many species of methanogens. The different CH4 microorganisms have physiological properties in common and their characteristics vary along with the micro-climate within the digester. As living organisms, they tend to prefer their own certain conditions. The balance between microbial populations must be maintained for optimal biogas production. However, there are many facilitating and inhibiting factors that affect the microbial processes, such as temperature, type of feedstock, pH, toxicity, and hydraulic retention time (HRT) since microorganisms are sensitive to the micro-climate. Thus, the stability and reliability of the anaerobic process are very fragile because methanogenic microorganisms develop slowly and are sensitive to sudden change of biological, physical, and chemical conditions in the digester. Some of these factors are discussed below.
Temperature
Temperature is a very critical factor, which affects activities of the methanogens. Sudden changes in temperature adversely affect the biogas production. The methanogens are inactive in extreme high and low temperatures. The optimal temperature for AD process is 30–40°C for mesophilic and 50–60°C for thermophilic processes. The rate of methanogen bacterial growth is faster under thermophilic conditions, resulting in a faster waste degradation. When the ambient temperature goes down to 10°C, gas production virtually stops. Since unheated plants and digesters without insulation do not work satisfactorily when the mean temperature is below 15°C, proper insulation of digester helps keep temperature and increase gas production in the cold season. For cold climate, proper heating may be necessary to maintain the optimal temperature condition in the digester. Some laboratory anaerobic digesters were carried out over the temperature range of 10–23°C (Safley and Westerman, 1994). Dairy and swine manure were fed into the digesters at the rates of 0.1 and 0.2 kg volatile solids (VS)/m3-day. The digesters were operated successfully with little indication of instability.
pH value and alkalinity
Microorganisms producing CH4 prefer a neutral to slightly alkaline environment. Methanogenic bacteria are very sensitive to pH value and do not thrive at a very high or low pH. General range of pH value in the digester is between 6.4 and 7.4. The optimal biogas production is achieved when the pH value is between 7 and 7.1. The pH in a biogas digester also is a function of the retention time. In the initial period of fermentation, acid-producing microorganisms grow quickly; they may produce large amounts of organic acids, which cannot be consumed by the methane-forming microorganisms. The pH inside the digester can drop quickly. This inhibits or even stops the digestion or fermentation process.
Retention time
Retention time is also known as hydraulic retention time (HRT). The time is the number of days that the feedstock stays in the digester. HRT is depending on digester structure, loading rate, feedstock types, and temperature. The retention time of a digester is calculated by dividing the total volume of the digester by the volume of inputs added daily. Furthermore, a digester should have a volume of 50–60 times the slurry added daily. Thus, a retention time is of 8–100 days, depending on local climatic conditions (FAO, 1996; Kim et al., 2006; Bond and Templeton, 2011). The HRT is also dependent on the temperature and up to 40–50°C. Thermophilic digesters showed higher rate of sCOD removal than mesophilic ones. The rates of biogas production by thermophilic digesters were generally higher than those by mesophilic digesters, which means a shorter HRT for thermophilic. A higher temperature than 55°C or a lower temperature than 40°C decreased the removal rates of sCOD (Kim et al., 2006). But for a biogas digester in cold climate, a longer retention time is needed so that the pathogens present in human feces are destroyed (FAO, 1996).
Loading rate
Loading rate is the amount of raw materials fed per unit volume of digester capacity per day. The loading rate units are kilograms of feedstock per cubic meter of digester volume per day (kg/m3/d), which will be depending on digester volume, concentration of feedstock, HRT and the ratio of Carbon to Nitrogen (C/N). Loading rate is also expressed in sCOD or VS as the fraction of organic matter in raw materials, which is biodegradable. The loading rate can be calculated using influent concentration and HRT. The digester size reduces as the loading rate decreases. If the digester is overfed, acids will accumulate and CH4 production will be inhibited because microorganism cannot survive in acidic situation. Similarly, if the digester is underfed, the gas production will also decrease. Santosh et al. (2004) reported that when loading rate varied at 346–1030 kg VS per day biogas yield was at 67–202 m3/day for a 100-m3 biogas plant operating on manure. Higher loading rates are recommended only in cases where mean ambient temperature is high.
Toxicity and inhibition
There are many toxic materials to AD such as fungicides, antibacterial agent, mineral ions, heavy metals, and the detergents (Chen et al., 2008). Some of the toxic materials inhibit the normal growth of pathogens in the digester. The anaerobic process can handle small quantity of mineral ions (e.g., sodium, potassium, calcium, magnesium, ammonium, and sulfur) and heavy metal (e.g., copper, nickel, chromium, zinc, and lead) without difficulty. However, very heavy concentration of these ions will have toxic effect as well as the heavy metal. For instance, slight increase of NH4 will benefit the microorganism growth, whereas its high concentration will be toxic to microorganisms. Sung and Liu (2003) reported that NH3 concentrations of 4.92, and 5.77 g/l caused a drop in CH4 production by as much as 39% and 64% because nitrogen is an essential nutrient for microorganisms. As NH3 concentrations increased in the range of 8–13 g/l, NH3 concentration causing 100% inhibition occurred, depending on acclimation condition and system pH. Acidogenic populations in the sludge were hardly affected (Koster and Lettinga, 1988). There are significant differences of inhibiting ammonia concentrations due to the differences in feedstocks, inocula, environmental conditions (temperature, pH), and acclimation periods (Chen et al., 2008).
Process Modeling, Monitoring, and Control of Biogas Production
A digester is a physical structure, made of various construction materials in different shape and size. It should be air and water tight, commonly known as the biogas reactor. Its main function is to provide anaerobic condition. The efficiency of biogas digester is strongly affected by operating conditions, feedstock, and digester design. Therefore, the critical stage of developing high biogas productivity is establishing an effective microbial community containing methanogens during inoculation with organisms from an operating digester. Once established, the digester will perform well and have a more robust performance even when difficult or contaminated feedstock is introduced. More importantly inoculation appears to set the operational production capacity. Inoculation environment to build microbial capacity or density of the consortia is used to determine the ultimate performance and robustness to difficult feedstock in a digester. To upscale this optimal biogas production to digester scale, the process modeling, monitoring, and control do improve functionality, reliability, and efficiency. There are five key processes to be controlled in the digester: (1) primary inoculation of both hydrophobic or immobilization surfaces and the formation of biogas granules, (2) an effective microbial community containing methanogens during inoculation with organisms from an operating digester, (3) the relevant kinetic parameters driving fermentation and productivity in digester, (4) predictive control model to cope with the large delay in the process, and (5) a robust adaptive control strategy that is robust to small changes in operation conditions and feedstock but adaptive to significant changes.
There are various types of digesters, such as fixed dome in China, floating dome in India, covered lagoon, and bag design in Taiwan (Bisschops et al., 2009). Analysis of biogas models will depend on digester types to assist process monitoring and control, which in turn can help optimize digester or fermenter operation and maximize biogas production. Therefore, application of the mathematical models is a prerequisite to improve performance of digester, and various models have been developed for the AD process from steady to dynamic models (Batstone et al., 2000; Bernard et al., 2001; Mu et al., 2008; Donoso-Bravo et al., 2009, 2011; Tomei et al., 2009). Mu et al. (2008) developed no. 1-based distributed parameter model of an anaerobic reactor for upflow anaerobic sludge bet rector. Balmanta et al. (2014) demonstrated the existence of optimal HRT and inlet mass flow rate of feedstocks for maximum biogas production through numerical simulations performed with a general transient mathematical model of an anaerobic digester introduced in this study.
Since various chemical and microbiological reactions take place in the digester, it is desirable to maintain its operating conditions in an optimal range of parameters (e.g., volatile acids, alkalinity, temperature, percent CO2, pH, and HRT). Objectives of process monitoring and control are to control food supply, temperature, pH, and feed rate to maintain a proper balance between the acid-forming and the methane-forming bacteria. Gelegenis et al. (2007) carried out a series of laboratory experiments in continuously stirred tank reactors at mesophilic conditions. Semi-continuously various mixtures of diluted poultry manure and whey were fed for co-digestion, which was proved to be possible without any need of chemical addition up to 50% participation of whey (by volume) to the daily feed mixture. In this regard, specific biogas production remained roughly unchanged at the various whey fractions added in the feed mixture, mainly due to the lower sCOD of whey compared to that of manure. Cáceres et al. (2012) developed a thermodynamic model of AD for predicting the potential production of biogas and its composition and a dynamic model of a biogas-fueled microturbine system for distributed generation applications has been developed using the MATLAB/Simulink software. However, due to different feedstocks and digester structures, it is difficult to determine a general optimal operating condition.
Biogas produced by AD of animal manure is as early as 3000 years ago. There are well-document knowledge of principle and technology of biogas production. However, with reliability and durability requiring, we still face a huge of problems. As mentioned above Section “Operating Conditions of Anaerobic Digestion,” all the parameters, such as temperature, C/N ratio, pH, loading rate, HRT, and inhibition, are depending on types of feedstocks and environmental conditions. Thus, the design of digester systems is still performed by rule-of-thumb (Baerel, 2006). This is mainly due to the fact that AD process is not yet fully understood due to complex feedstocks and environmental conditions. It is clear that we do not address single issues in isolation, but through a systems approach that delivers integrated solutions. Most of the above modeling and experiments are limited in certain feedstocks and environmental conditions. There is still lack of comprehensive data for mixture of various feedstocks. More research is necessary to further optimize AD processes. Due to variation of feedstocks and environmental conditions, there are currently no general optimal parameters and models available for an accurate evaluation of performance. The complexity of the microbial activity is seen as one of the main reasons for the lack of basic knowledge on digestion systems. Further development and optimization of AD requires fundamental knowledge on micro-scale, which should in turn be linked to the macroscale system performance (Appels et al., 2011). Optimal parameters and AD processes under local conditions could be a solution.
Feedstock Resources in Farm Ecosystem
Economic Factors
A wide range of agricultural crops, residues, manure, and organic wastes are potentially available for use as feedstocks of AD in agricultural farms. Generally speaking these feedstocks can be categorized into two type of feedstock supply in a whole-farm context: (i) livestock waste (e.g., manure, and food litter), and (ii) general cropping (e.g., forage, silage, energy crops, grass, straw, wood chip). In terms of choice of feedstocks, a farm ecosystem based on livestock manure is appropriated on livestock farms, and on forage appropriate on arable farms. If biodegradable wastes are easily available as feedstocks, the benefits in dealing with the biodegradable waste could be of twofold: (i) economic value of biogas and its digestate; and (ii) environmental cost avoided in some other ways such as disposal in landfill (Jones, 2010). However, the economic benefits of outputs (both biogas and digestate) may become low if the feedstock are costly such as transport and storage or have to be purchased. For instance, processing residues/waste, like manure and straw, are great cheap source of biomass, but if not just onsite use, it may not always economic viable if consider supply variance [e.g., technology, associated investment costs (e.g., transport and storage), and output product prices]. Therefore, for economic and technical reasons, some materials are more preferred as feedstocks than others, depending on availability of feedstocks and climate conditions in local farms.
A small-scale plants can produce lower cost power, increased employment, environmental improvements. However, broad judgments about the economic viability of AD at the farm scale are difficult to make because of the heterogeneous context in which AD operates, e.g., differences in farm size and system, income, availability of feedstocks, and the scale at which AD is operated (Jones, 2010; Jones and Salter, 2013). Yang et al. (2012) showed that clear regional differences for the potential of biogas development, and special funds were distributed scientifically in the process of biogas development. Governmental policy support is the major driving force for the deployment of biogas in China. A total of US$3.8 billion is invested by the government for biogas deployment from 2003 to 2010 (Feng et al., 2012). In Kenya, it was difficult for farmers to afford biogas systems without subsides and respectively 46 and 57% of biogas plant owners received subsidies covering over 25% of the construction costs (Mwirigi et al., 2009). Therefore, what scales are optimal economically on farm type and size? What are the practical requirements to deploy AD biogas technology (e.g., digestate disposal, income, labor availability, etc.) and in fact the supply variance and seasonal nature of feedstocks are major factors in managing systems for most biomass resources in a farm scale. In the cold climate, heating and insulation of digesters add extra cost. Essentially this requires developing a business model of biogas resource to be flexible as plant equipment. It is necessary to assess AD economical competition with alternative uses of crops to secure a supply of feedstocks, and economic viability of AD at the farm scale.
Feedstock Characteristics
Theoretically any biodegradable biomass can be used as feedstock for biogas production processing inside the digester. However, waste constituents are not equally degraded or converted to gas through AD and their potential to biogas production varies in terms of C/N ratios. Biomass with either excessive carbon or nitrogen can result in poor digester performance and biogas with high CO2 content. The digestion of biomass with high N and sulfur concentrations can produce high concentrations of ammonia and hydrogen sulfide. The high concentration of ammonia can increase the pH value to above 8 which will have toxic influence on CH4 microorganisms. On the other hand, if the C/N ratio is very high, methanogens will consume N rapidly for meeting their protein needs and will no longer react on the left over C content. The plant feedstock (e.g., straw, grass, and sawdust) have a high C/N ratio of 60–200 and animal manure (e.g., pig, sheep, and cattle dung) have a higher C/N ratio of 18–30. However, chicken and duck manure has a lower C/N ratio of 8–10. Biomass with a C/N ratio between 20 and 30 has been reported to produce optimal biogas composition (FAO, 1996; das Neves et al., 2009). Furthermore, wastes that are not particularly water-soluble will breakdown slowly and anaerobic microorganisms do not degrade lignin and some other hydrocarbons. Thus, cattle and dairy wastes will degrade slower due to a high C/N ratio than swine or poultry manure.
Feedstocks containing high C/N ratio could be mixed with those containing low C/N ratio to average C/N ratio of the feedstock input to a desirable level. The feedstocks fed into the digesters will not be totally feedlot manure but will also consist of other organic materials (e.g., such as stillage, straw, grass, ground-up pallets, hog mortalities, and food wastes) (Clemens et al., 2006; AG ANNEX News, 2011). A specific mixture will achieve the optimal biogas production. Thus, a homogenous mixture of two or more feedstocks applied for digestion is digested at the same time, so-called co-digestion. It has been realized that co-digestion can improve biogas production and stability. A Spain team (AG ANNEX News, 2011) has tested in vitro the combination of pig slurry with agricultural by-products (e.g., peppers, tomatoes, peaches, and kaki) to study biogas production and the optimal combination of feedstocks. It has been found that peppers increased biogas production by 44% compared with slurry-only; tomatoes, by 41%; and peaches, by 28% (AG ANNEX News, 2011). There is no any difference observed for kaki. In co-digestion of crop straw and manures (Lehtomäki et al., 2007), manures provide buffering capacity and a wide range of nutrients, while the addition of straw with high carbon content balances the C/N ratio of the feedstocks, thereby decreasing the risk of ammonia inhibition. In China, as a means to balance C/N ratio, it is customary to load rice straw at the bottom of the digester upon which latrine waste is discharged. Similarly, in Chitawan district of Nepal, elephant dung was fed in the digester in conjunction with human waste to balance C/N ratio for optimal biogas production (Karki et al., 1994). Risberg et al. (2013) studied co-digestion of steam exploded or non-pretreated wheat straw with cattle manure. They had not found improved biogas yield when co-digesting wheat straw with manure. Panyadee et al. (2013) showed the methane production increased by 34% when the substrate was switched from food waste to the mixed food waste, and no signs of inhibition were observed. Heaven et al. (2011) investigated co-digestion with cattle slurry or with vegetable waste. They found acceptable specific and volumetric methane productions, and a digestate low in potentially toxic elements. Therefore, most of biogas plants make co-digestion between three and five feedstocks concurrently, leading ~10% higher biogas yield compared to single feedstock digestion (Pöschl et al., 2010; Galante et al., 2012).
Digestate Used as Fertilizer
Both fluid and solid digestates are rich in N, phosphorus (P), and potassium (K) as an organic fertilizer, thereby providing a sustainable substitute for synthetic fertilizers (Vaneeckhaute et al., 2013) and recycling nutrients for organic crop production to off-set the use of synthetic fertilizers. All organic fertilizers stimulated potential organic food production for ecosystem farm. However, different feedstocks and AD treatment generate digestate of differing chemical composition compared to undigested animal manures, which may affect the soil microbial ecosystem differently and plant growth when used as fertilizers (Abubaker et al., 2012, 2013). Bertora et al. (2008) showed that N2O and CO2 emissions as well as denitrification processes and nitrate availability are depending on AD processes with an equal amount of total N supplied, the C slurry and NH4+ content, and the complexity of the organic compounds. In order to fully exploit biogas digestate in crop production, it is necessary to investigate the effect of different soil types and biogas digestate types on crop growth and GHGs and to devote more effort to developing biogas processes that not only produce biogas but also organic fertilizers.
Soil Amendment of Digestate
The AD process produces three valuable components from the manure and food by-products: biogas, fluid digestate, and solid digestate. Biogas consists of 50–60% CH4, 40–45% CO2, and trace amounts of hydrogen sulfide (H2S). As a result of the AD process a number of changes in slurry composition can be expected. Both fluid and solid digestates are nutrient rich in N, phosphorus (P), and potassium (K) as an organic fertilizer with free of seed, pathogens, and odors. That is because the digestate resulting from the anaerobic process uses only the C, but the N, P, K, and micronutrient components remain intact (Kryzanowski, 2013). Therefore, the nutrient profile of the digestate is much the same as the nutrient profile of the feedstock sources and digestate are excellent sources of nutrients. There are a substantial reduction of 25% in solids content and a consequential increase in ash content, due to the conservation of minerals and reduction of slurry organic matter (ADAS and SAC, 2007). Increases in slurry pH are estimated at about 0.5 pH units and increases in ammonium N content is up to one-quarter. These changes are less consistent than the reduction in solids content and organic matter content, depending on digester operating conditions, and the analysis of the feedstock slurries (ADAS and SAC, 2007). However, Total N is obviously increases after AD treatment. The digestate can continue to be land applied as an organic fertilizer on the farm owner’s land. One of the possible consequences of the increase in slurry pH and NH4-N content following AD is an increased risk of NH3 losses during storage, and ammonia and N2O emissions after land application. The increase in slurry ammonium N content, usually with increase in pH and reduction in solids content, may result in a risk of increase during post-digestion storage (Smith et al., 2007). Such increased emissions during storage can be effectively controlled by a range of store coverings. Sanchez et al. (2008) studied the degree of stability attained by livestock farm wastes through AD. The stability of digestate is related to manure type and temperature. An important but little understood aspect of biogas production is its overall impact on soil C and N cycling. Increased biogas production from organic waste will inevitably lead to higher input of its digestate to the soil amendments as fertilizers (Arthurson, 2009).
Möller and Stinner (2009) showed that N2O–N emissions increase remarkably after shallow injection of slurry in closed slots, particularly if digested slurry was applied. This indicated that enhanced denitrification from nitrification, related to a higher supply of readily available NH4–N for the nitrifying organisms, was the main driving force for course and amounts of emitted N2O (Moller, 2009). Huang et al. (2004) showed that the N2O–N emission fraction for mixture of residues and urea amendment is dependent on the C/N ratio when plant residue was incorporated with lower C/N ratio of the residues inducing higher concentration of C and larger amount of N2O emission. Cumulative emissions of N2O and CO2 were negatively correlated with the C/N ratio in plant residues. High concentration of N in digestate is valuable to help promote crop growth and has potentials to play a key role in improving soil sustainability. However, overuse may contribute to soil, water, and air pollution. Therefore, to a large extent the sustainability of a particular biogas production chain will depend on the possibilities for its digestate disposal (Taheripour et al., 2010). Digestate fertilizer may have a large environmental footprint due to the emissions of GHGs during both AD and use, mainly as CO2 evolved during AD and N2O evolved as the soil fertilizer. N2O is a particularly potent GHG with a global warming potential almost 300 times that of CO2. Emissions of NH3, N2O, and CH4 have been compared among undigested and digested pig and cattle slurry applications in Finland (Regina and Perälä, 2006). Pig slurry was applied on barley with target soluble-N application rates of 100 kg/ha. When undigested or digested pig slurries were injected, no NH3 emissions were detectable in field experiments in 2005–2006. Where the slurries were land spread before sowing the barley crop NH3 emissions continued until the slurry was incorporated (1 h after application). Compared to fertilizer properties, a final byproduct (digestate) through AD was obtained with very good fertilizing properties because of the high nutrient content (N, P, K) in available form. In this regard, the digestate appears to be a very good substitute of synthetic fertilizers, also contributing, to the short-term soil organic matter turnover (Tambone et al., 2010; Chen et al., 2012). However, it is still unclear how these digestate will affect microbial activity and transformation processes in soil, and thereby its GHG balance and organic C sequestration. For instance, little is known about their effects on the GHG balance of the soil, in particular on N2O emissions for many animal by-products approved by organic farming regulations (Cayuela et al., 2010a,b).
Modeling of Digestate Used as Fertilizer
A more natural approach to evaluate the quality and influence of digestate is to test their performance in the soil–plant system. The plant is the ultimate integrator and the quality of the fertilizer can be assessed by observing the growth and yield of plant under fully controlled conditions. Attempts were carried out to model soil-plant interaction and GHGs when digestate was used as fertilizer (Wang et al., 2011) (Figure 3). The model was a framework of modified DeNitrification and DeComposition (DNDC) (Li et al., 1992; Wang et al., 2012). When digestate, fertilizer, or manure is inputted into the soil, N turnover is regulated by denitrifiers or nitrifiers. N2O production/consumption is controlled by three factors, namely soil redox potential (Eh), dissolved organic C (DOC) concentration and available N, i.e., ammonium (NH4+) or nitrate (NO3−) concentration. When Eh = 250−350 mV, DeNitrification is dominant due to oxygen depletes. When Eh = 350−700 mV, Nitrification is dominant due to rich oxygen. Natural processes (e.g., rainfalls and temperature) or management practices (e.g., tillage and irrigation) can alter availability of one or more of the three driving factors and hence affect N2O production. The N2O production will be reduced or stopped if any of the three factors becomes limiting (Figure 3). The production and consumption of CO2, N2O, and CH4 are calculated based on the soil redox potential evolution sequentially for grassland, arable, or forest ecosystems (Li et al., 1992; Wang et al., 2012).
The modeling has shown that N2O emissions of the digestate are dependent on C/N ratio. The emissions increase as C/N ratio decreases (Figure 4). When C/N is under a value, N2O emissions of the digestate can be much lower than those of the synthetic fertilizer. The digestate may be replacement of the fertilizer for sustainable agriculture if proper applications. These nutrients recycle through the timely application of the digestate to land. This largely eliminates the need for synthetic nutrient purchases. As a result, only modest fertilizer purchases would be required (whether synthetic, inorganic or otherwise) to replace nutrients lost from the soil, for example, through leaching into ground water, during the cultivation and growing period.
Economic and Technological Barriers for Deployment of Biogas Technology in Rural Region
Despite many opportunities for farm biogas development in rural regions and some successful pilot plants (ADAS and SAC, 2007), the widespread adoption of decentralized biogas technology has yet to take off. Decentralized biogas production from manure and crop residues is not currently economical and reliable because of its low energy content, reliability, durability, high capital costs, and low efficiency. There are clear regional differences for the potential of biogas deployment, and special funds were distributed scientifically in the process of biogas deployment (Yang et al., 2012). AD in developing countries has far greater relevance to short energy supply than that in developed countries. Thus, many efforts have been made for energy production via biogas plants, particularly in China and India. In developed countries AD was used but primarily as a process for treating high-sCOD waste rather than as a means of generating energy (biogas). As mentioned before, all the parameters are depending on feedstocks and environmental conditions. Therefore, lots of practical problems in deployment of biogas technology are due to local conditions rather than technology itself, such as low-quality construction, national financial subsidies, the availability of biogas feedstocks, low biogas production, optimal operating conditions, the buildup of sand, leakage of pipelines, easy operation and maintenance, and farmer skills (Chen et al., 2010; Cheng et al., 2014).
Economic Barriers
An essential barrier is the high cost and low performance of biogas digester for the biogas commercialization. For digesters with electricity-generating potential, it is suggested that a rough threshold size of 300 cows or 2000 swine is required due to economies of scale in construction and operation (Minnesota Project, 2014). Improved technologies may reduce this threshold size. While the construction costs of biogas plants vary between different countries they are often high relative to the income of farmers and other potential users. The stories in the developing countries such as China and Nepal are many thousands of simple, small-scale digesters that produce biogas for heating, cooking, and lighting needs, so-called decentralized biogas technology. A plant of short life could also be cost effective but such a plant may not be reconstructed once its useful life ends. In China, the farm biogas development was mainly affected by combined effects of climatic conditions and economic level (Yang et al., 2012). This results in inadequate financial returns and practical benefits if without government intervention. Especially, while people are yet to be motivated for the adoption of this technology or the necessary skill and materials are not readily available, high quality and durability biogas plants can demonstrate good benefits although this may require a higher initial investment. Therefore, large scale deployment of decentralized biogas technology requires governmental policy support as the major driving force. The government invested a total of US$3.8 billion for biogas development from 2003 to 2010 (Feng et al., 2012). A number of digesters (186) currently operate commercially in the U.S. agriculture in which about 30% of these digesters co-digest other feedstocks with manure. The number of community digesters is growing under USDA funding support (EPA, 2010, 2012). Although most of digesters are centralized in U.S., the main barriers to widespread digester use are still on high capital costs, investor risk, variability in feedstock and byproduct markets, and policy (EPA, 2012).
Variability of Feedstocks and Environmental Conditions
Problems (e.g., low biogas yield and process instability) are often encountered in decentralized AD biogas production, preventing this technique from being widespread (Chen et al., 2008). Considerable research efforts have been made to identify the mechanism and the controlling factors of biogas production and digester inhibition. There is a large discrepancy of biogas production performance between pilot plants and practical plants regardless of the social and economic factors. These discrepancies arise, because in most cases, due to uncertainty of feedstocks and environmental conditions in the specific area, where the AD biogas production processes take place. The pilot digesters are designed usually according to anticipated feedstocks and climate conditions. However, it is difficult to keep main parameters of scalable digesters consistent with those of the pilot digester due to unavailability of feedstocks. For instance, manure productions were not uniformly distributed geographically in Canada concentrated at three major regions in 2006 as shown in Figure 5 (Hofmann, 2006). There was similar non-uniform distribution of livestock production in 2012 (Statistics Canada, 2012). The uneven distribution of manure causes a difficulty for suitability of co-digestion to achieve the optimal biogas production that the biogas plant requires. Due to the difference in waste feedstock composition, optimal operating conditions in pilot plant needs to be adjusted for some local complex feedstocks and climate. For instance, due to change of feedstock availability, C/N ratio of feedstock mixture will be changed. This means that all the optimal parameters in pilot plant must be calibrated to the new conditions as well. However, this is not common practice in deployment of biogas technology due to lack of local data now. For instances, it has been reported that biomass with a C/N ratio between 20 and 30 has been reported to produce optimal biogas composition (FAO, 1996; das Neves et al., 2009). However, it is difficult for farmers to keep the mixture of feedstocks in designed types due to a wide variation of feedstocks. Practical co-digestion is dependent on availability of feedstocks as well as farmers’ knowledge. In this regard, this variation of inhibitions can be attributed to feedstocks change. Thus, many digesters became defunct after a short operation period due to sudden variation of feedstocks or environmental conditions (Bond and Templeton, 2011). As a result, the operational status of biogas digesters is not optimal and well-operation ratio is about 53% in practice in Nepal (Cheng et al., 2014). In China the total number of digesters had reached seven million by 1978, but only three million of them were actually in operation (He, 2010). The complex feedstocks supplies result in a wide variety of inhibitory substances which are the primary cause of anaerobic digester upset or failure since they are present in substantial concentrations in wastes. This results essentially from the root causes of suitable feedstocks shortage.
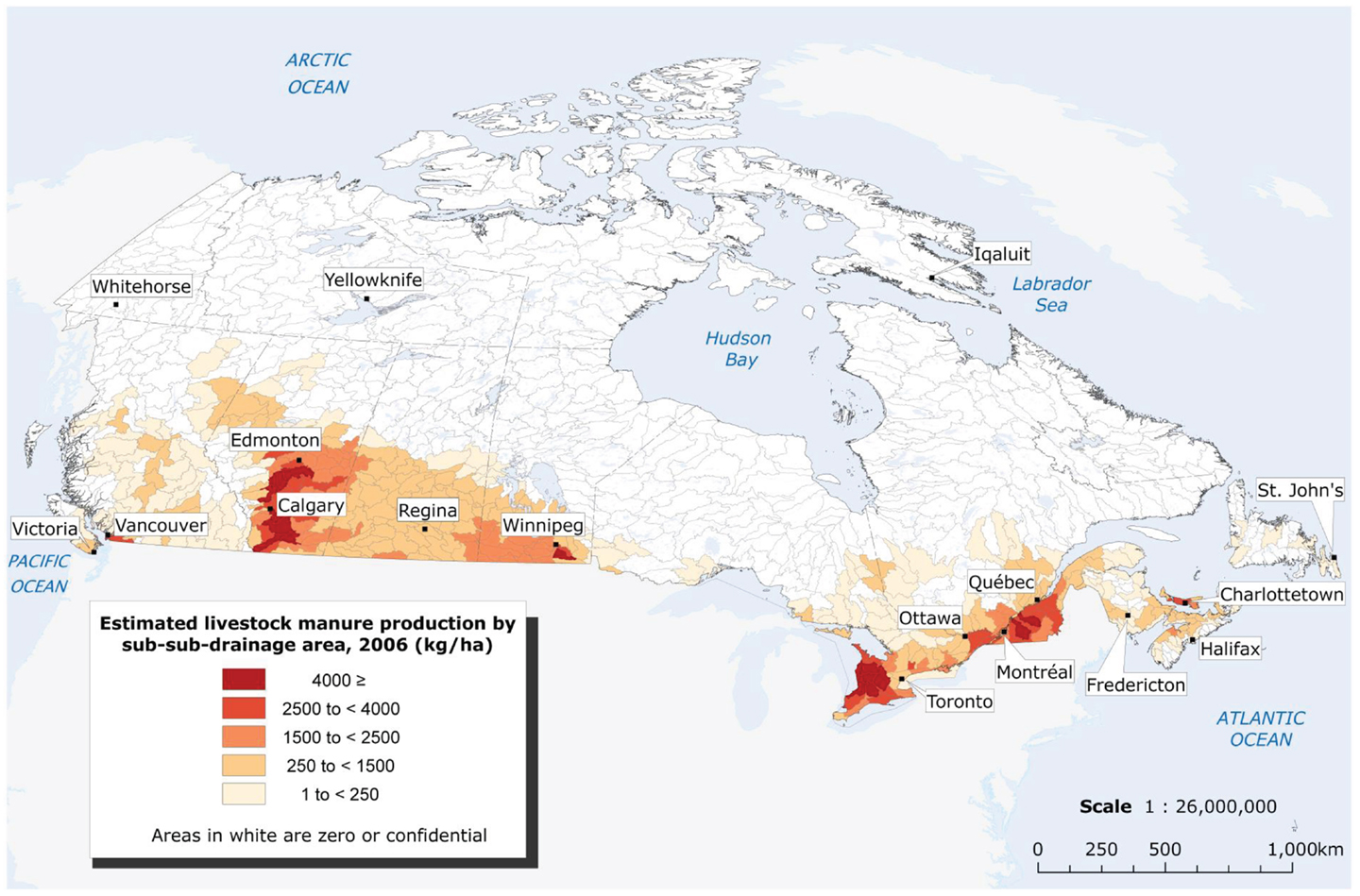
Figure 5. A geographical profile of livestock manure production in Canada (Hofmann, 2006).
Variability of Climate Zones
Also, more research needs to be done in cold fermentation technology (Balasubramaniyam et al., 2008). Biogas production is suitable for countries where the ambient average temperature ranges above 15°C. AD ability is significantly reduced below 8°C. The process is also sensitive to temperature variations of more than 3°C; therefore temperature variations have to be kept in a limited range to ensure a steady biogas production. Biogas production decreases in cold weather or at higher altitude, ironically, in a time and place when the farm energy requirement substantially increases. However, AD at cold psychrophilic temperature (<20°C) has not been as extensively explored as those mesophilic or thermophilic digestion. Generally a lower temperature needs a longer HRT to achieve a similar gas production. Most of the anaerobic digesters require heating and insulation in the cold weather for optimum biogas production in Canada. Therefore, digesters with good insulation can keep the digester in optimum operating temperature, which results in extra cost.
Solid Inorganic Rubbish
Since waste might be contaminated with sand, rock, plastics, rubber, grit, and dirt, these sands or plastics in the digesters had to be cleared out regularly. The cleaning out of sand buildup is a hard job and it was not a good option to shut down the digesters to clean out the sand at regular intervals. It may be difficult for farmers to find a supplier with experience disposing of the buildup of sand in the digester on a continuous basis (Kryzanowski, 2013). Thus, it is desirable that a digester needs to be designed on a potential site that would be able to use some dirty manure and feedstocks. Certain degree of specialized skill is required for construction of a biogas plant, which may not be easily available for farmers. Furthermore, operators need know the appropriate range of the main parameters under monitoring and control, such as alkalinity, volatile acids, pH, loading rate, and temperature. All these are linked to inadequate emphasis on training, operation, maintenance, and repair of existing facilities. Biogas users need to be trained on operation and maintenance including some minor repairs.
Concluding Remarks
Long-term economic and environmental concerns have resulted in a great amount of research in the past couple of decades on renewable sources of biomass and bioenergy to replace fossil fuels. Decentralized biogas technology uses agricultural waste materials (e.g., animal manure, crop straw, and by-products from food industries) as feedstocks. By-products from biogas production, called digestate, are nutrient rich, which could potentially be reused as green fertilizers in farms, thereby providing a sustainable candidate for synthetic fertilizers for farm ecosystem. The modeling has shown that N2O emissions of the digestate are dependent on C/N ratio and the emissions increase as C/N ratio decreases. The digestate can be replacement of the synthetic fertilizer for sustainable agriculture if proper applications. The recycling of these nutrients through the timely application of the digestate to land largely eliminates the need for synthetic fertilizer purchases. Thus, the AD biogas production is win–win option for livestock producers to address issues of energy production and waste management, and avoid contamination of surface and ground waters and emissions of odors, ammonia, and greenhouse gases. However, there are still economic and technological barriers to be addressed during large scale deployment of biogas technology. Main barriers are a wide variation of feedstocks and environmental conditions (e.g., temperature) over space and time. Particularly, there are main challenges to design universal digesters and optimize AD process parameters due to variation of feedstocks and environmental conditions. Two national scale deployments in China and Nepal showed that the operational status of biogas digesters is not optimal and up to 50% of plants are non-functional after a short operation period regardless of the social and economic factors. It becomes clear that the experimental conditions of the pilot plants are not sufficient for complex variability of feedstocks and climate for a wide range of decentralized biogas technology. A digester need to be adjusted and calibrated carefully, even redesign, to fit the local feedstocks and climate. Digesters with heating or insulation need to be developed for cold climate as well. Furthermore, operators need know the appropriate range of main parameters for monitoring and control, such as alkalinity, volatile acids, pH, feed rate, and temperature. All these are linked to inadequate emphasis on design, training, maintenance, and repair of existing facilities. Biogas users need to be trained on operation and maintenance including some minor repairs. Thus, collaboration of all relevant designers, farmers, stakeholders, and regulators is proposed as the way forward, particularly as their complexity has been identified as the major hurdle to the implementation of decentralized biogas production, which can deliver beneficial synergies for the decentralized biogas production in rural region responsible for food production and waste management.
Conflict of Interest Statement
The author declares that the research was conducted in the absence of any commercial or financial relationships that could be construed as a potential conflict of interest.
References
Abe, N., Tang, Y. Q., Iwamura, M., Ohta, H., Morimura, S., and Kida, K. (2011). Development of an efficient process for the treatment of residual sludge discharged from an anaerobic digester in a sewage treatment plant. Bioresour. Technol. 102, 7641–7644. doi: 10.1016/j.biortech.2011.05.030
Abubaker, J., Cederlund, H., Arthurson, V., and Pell, M. (2013). Bacterial community structure and microbial activity in different soils amended with biogas residues and cattle slurry. Appl. Soil Ecol. 72, 171–180. doi:10.2134/jeq2012.0247
Abubaker, J., Risberg, K., and Pell, M. (2012). Biogas residues as fertilisers – effects on wheat growth and soil microbial activities. Appl. Energy 99, 126–134. doi:10.1016/j.apenergy.2012.04.050
ADAS. and SAC. (2007). Nutrient value of digestate from farm-based biogas plants in Scotland. Defra Report DA/009/06. Available at: http://www.scotland.gov.uk/Resource/Doc/1057/0053041.pdf
AG ANNEX News. (2011). Available at: http://www.agannex.com/anaerobic-digestion/in-the-news-september-october-2011
Aiyuk, S., Forrez, I., Lieven, D. K., van Haandel, A., and Verstraete, W. (2006). Anaerobic and complementary treatment of domestic sewage in regions with hot climates – a review. Bioresour. Technol. 97, 2225–2241. doi:10.1016/j.biortech.2005.05.015
Appels, L., Lauwers, J., Degrève, J., Helsen, L., Lievens, B., Willems, K., et al. (2011). Anaerobic digestion in global bio-energy production: potential and research challenges. Renew. Sustain. Energy Rev. 15, 4295–4301. doi:10.1016/j.rser.2011.07.121
Arthurson, V. (2009). Closing the global energy and nutrient cycles through application of biogas residue to agricultural land – potential benefits and drawbacks. Energies 2, 226–242. doi:10.3390/en20200226
Baerel, D. (2006). Will anaerobic digestion of solid waste survive in the future? Water Sci. Technol. 53, 187–194. doi:10.2166/wst.2006.249
Balasubramaniyam, U., Zisengwe, L. S., Meriggi, N., and Buysman, E. (2008). Biogas Production in Climates with Long Cold Winters. Available at: http://www.sswm.info/sites/default/files/
reference_attachments/BALASUBRAMANIYAM%202008%20Biogas%20Production%20in%20
Climates%20with%20long%20cold%20Winters.pdf
Balmanta, W., Oliveiraa, B. H., Mitchella, D. A., Vargasb, J. V. C., and Ordonez, J. C. (2014). Optimal operating conditions for maximum biogas production in anaerobic bioreactors. Appl. Therm. Eng. 62, 197–206. doi:10.1016/j.applthermaleng.2013.09.033
Batstone, D. J., Keller, J., Newell, R. B., and Newland, M. (2000). Modelling anaerobic degradation of complex wastewater. I: model development. Bioresour. Technol. 75, 67–74. doi:10.1016/S0960-8524(00)00018-3
Bernard, O., Hadj-Sadok, Z., Dochain, D., Genovesi, A., and Steyer, J.-P. (2001). Dynamical model development and parameter identification for an anaerobic wastewater treatment process. Biotechnol. Bioeng. 75, 424–438. doi:10.1002/bit.10036
Bertora, C., Alluvione, F., Zavattaro, L., van Groenigen, J. W., Velthof, G., and Grignani, C. (2008). Pig slurry treatment modifiers slurry composition, N2O and CO2 emissions after soil incorporation. Soil Biol. Biochem. 40, 1999–2006. doi:10.1016/j.soilbio.2008.03.021
Biogas Association. (2013). Canadian Biogas Study: Benefits to the Economy, Environment and Energy. Available at: http://www.biogasassociation.ca/bioExp/images/uploads/documents/2014/biogas_study/
Canadian_Biogas_Study_Technical_Document_Dec_2013.pdf
Bisschops, I., Spanjers, H., and Schuman, E. (2009). Development of Decentralised Anaerobic Digestion Systems for Application in the UK: Phase 1 – Final Report. Available at: http://www.communitycompost.org/CCN_documents/Small_scale_AD_for_CCN_Phase1_report.pdf
Bond, T., and Templeton, M. R. (2011). History and future of domestic biogas plants in the developing world. Energy Sustain. Dev. 15, 347–354. doi:10.1016/j.esd.2011.09.003
Cáceres, C. X., Cáceres, R. E., Hein, D., Molina, M. G., and Pia, J. M. (2012). Biogas production from grape pomace: thermodynamic model of the process and dynamic model of the power generation system. Int. J. Hydrogen Energy 37, 10111–10117. doi:10.1016/j.ijhydene.2012.01.178
Cayuela, M. L., Oenema, O., Kuikman, P. J., Bakker, R. R., and van Groenigen, J. W. (2010a). Bioenergy by-products as soil amendments? Implications for carbon sequestration and greenhouse gas emissions. Glob. Change Biol. Bioenergy 2, 201–213. doi:10.1016/j.geoderma.2010.04.026
Cayuela, M. L., Velthof, G. L., Mondini, C., Sinicco, T., and van Groenigen, J. W. (2010b). Nitrous oxide and carbon dioxide emissions during initial decomposition of animal by-products applied as fertilisers to soils. Geoderma 157, 235–242. doi:10.1016/j.geoderma.2010.04.026
Chen, R. R., Blagodatskaya, E., Senbayram, M., Blagodatsky, S., Myachina, O., Dittert, K., et al. (2012). Decomposition of biogas residues in soil and their effects on microbial growth kinetics and enzyme activities. Biomass Bioenergy 45, 221–229. doi:10.1016/j.biombioe.2012.06.014
Chen, Y., Cheng, J. J., and Kurt, S. (2008). Creamer inhibition of anaerobic digestion process: a review. Bioresour. Technol. 99, 4044–4064. doi:10.1016/j.biortech.2007.01.057
Chen, Y., Yang, G., Sweeney, S., and Feng, Y. (2010). Household biogas use in rural China: a study of opportunities and constraints. Renew. Sustain. Energy Rev. 14, 545–549. doi:10.1016/j.rser.2009.07.019
Cheng, S. K., Li, Z. F., Mang, H. P., Neupane, K., Wauthelet, M., and Huba, E. M. (2014). Application of fault tree approach for technical assessment of small-sized biogas systems in Nepal. Appl. Energy 113, 1372–1381. doi:10.1016/j.apenergy.2013.08.052
Chung, J. N. (2013). Grand challenges in bioenergy and biofuel research: engineering and technology development, environmental impact, and sustainability. Front. Energy Res. 1:4. doi:10.3389/fenrg.2013.00004
Chung, J. N. (2014). A theoretical study of two novel concept systems for maximum thermal-chemical conversion of biomass to hydrogen. Front. Energy Res. 1:12. doi:10.3389/fenrg.2013.00012
Clemens, J., Trimborn, C. J., Weiland, P., and Amon, B. (2006). Mitigation of greenhouse gas emissions by anaerobic digestion of cattle slurry. Agric. Ecosyst. Environ. 112, 171–177. doi:10.1016/j.agee.2005.08.016
das Neves, L. C. M., Converti, A., and Penna, T. C. V. (2009). Biogas production: new trends for alternative energy sources in rural and urban zones. Chem. Eng. Technol. 32, 1147–1153. doi:10.1002/ceat.200900051
Demirbas, M. F., and Balat, M. (2009). Progress and recent trends in biogas processing. Int. J. Green Energy 6, 117–142. doi:10.1080/15435070902784830
Donoso-Bravo, A., Mailier, J., Martin, C., Rodríguez, J., Aceves-Lara, C. A., and Wouwer, A. V. (2011). Model selection, identification and validation in anaerobic digestion: a review. Water Res. 45, 5347–5364. doi:10.1016/j.watres.2011.08.059
Donoso-Bravo, A., Rosenkranz, F., Ruiz-Filippi, G., and Chamy, R. (2009). Development and validation of a simplified model for the anaerobic degradation of phenol. Water Sci. Technol. 60, 37–45. doi:10.2166/wst.2009.299
EPA. (2010). U.S. Anaerobic Digester Status Report. Available at: http://www.epa.gov/agstar/documents/digester_status_report2010.pdf
EPA. (2012). Case Study Primer for Participant Discussion: Biodigesters and Biogas. Available at: http://www.epa.gov/agstar/documents/biogas_primer.pdf
FAO. (1996). Biogas Technology: A Training Manual for Extension. Available at: http://www.fao.org/docrep/008/ae897e/ae897e00.HTM
Feng, Y. Z., Guo, Y., Yang, G. H., Qin, X. W., and Song, Z. L. (2012). Household biogas development in rural China: on policy support and other macro sustainable conditions. Renew. Sustain. Energy Rev. 16, 5617–5624. doi:10.1016/j.rser.2012.06.019
Galante, C. G., Pezzola, L., Priano, N., Scaramellini, S., and Sottocornola, A. (2012). Methane from Biogas: The Process, Cleaning and Projects, TPG 4140 Natural Gas Semester Report. Available at: http://www.ipt.ntnu.no/~jsg/undervisning/naturgass/oppgaver/Oppgaver2012/12Galante.pdf
Gelegenis, J., Georgakakis, D., Angelidaki, I., and Mavris, V. (2007). Optimization of biogas production by co-digesting whey with diluted poultry manure. Renew. Energy 32, 2147–2160. doi:10.1016/j.renene.2006.11.015
He, P. J. (2010). Anaerobic digestion: an intriguing long history in China. Waste Manag. 30, 549–550. doi:10.1016/j.wasman.2010.01.002
Heaven, S., Salter, A. M., and Banks, C. J. (2011). Integration of on-farm biodiesel production with anaerobic digestion to maximise energy yield and greenhouse gas savings from process and farm residues. Bioresour. Technol. 102, 7784–7793. doi:10.1016/j.biortech.2011.06.029
Hofmann, N., and Beaulieu, M. S. (2001). A Geographical Profile of Manure Production in Canada, 2001. Available at: http://www.statcan.gc.ca/pub/21-601-m/21-601-m2006077-eng.pdf
Hofmann, N. (2006). A geographical profile of livestock manure production in Canada, 2006. Available at: http://www.statcan.gc.ca/pub/16-002-x/2008004/article/10751-eng.htm#a4
Huang, Y., Zou, J. W., Zheng, X. H., Wang, Y., and Xu, X. K. (2004). Nitrous oxide emissions as influenced by amendment of plant residues with different C:N ratios. Soil Biol. Biochem. 36, 973–981. doi:10.1016/j.soilbio.2004.02.009
Huopana, T., Song, H., Kolehmainen, M., and Niska, H. (2013). A regional model for sustainable biogas electricity production: a case study from a Finnish province. Appl. Energy 102, 676–686. doi:10.1016/j.apenergy.2012.08.018
Johansen, A., Nielsen, H. B., Hansen, C. M., Andreasen, C., Carlsgart, J., Hauggard-Nielsen, H., et al. (2013). Survival of weed seeds and animal parasites as affected by anaerobic digestion at meso- and thermophilic conditions. Waste Manag. 33, 807–812. doi:10.1016/j.wasman.2012.11.001
Jones, P. (2010). Modelling the Commercial Profitability of AD Energy Production at the Farm Level within Arable and Dairy Systems. Available at: http://www.ad4rd.soton.ac.uk/publications/Farm_modelling_AD_finalreport.pdf
Jones, P., and Salter, A. (2013). Modelling the economics of farm-based anaerobic digestion in a UK whole-farm context. Energy Policy 62, 215–225. doi:10.1016/j.enpol.2013.06.109
Karki, A. B., Gautam, K. M., and Karki, A. (1994). Biogas installation from elephant dung at Machan Wildlife Resort, Chitwan, Nepal. Biogas Newsletter, Issue No. 45.
Kim, J. K., Oh, B. R., Chun, Y. N., and Kim, S. W. (2006). Effects of temperature and hydraulic retention time on anaerobic digestion of food waste. J. Biosci. Bioeng. 102, 328–332. doi:10.1263/jbb.102.328
Koster, I. W., and Lettinga, G. (1988). Anaerobic digestion at extreme ammonia concentrations. Biol. Wastes 25, 51–59. doi:10.1016/j.watres.2012.08.028
Kryzanowski, T. (2013). “Full circle,” in Manure Manager – March/April 2013. Available at: http://www.bluetoad.com/display_article.php?id=1341333
Lehtomäki, A., Huttunen, S., and Rintala, J. A. (2007). Laboratory investigations on co-digestion of energy crops and crop residues with cow manure for methane production: effect of crop to manure ratio. Resourc. Conservat. Recycl. 51, 591–609. doi:10.1016/j.resconrec.2006.11.004
Li, C. S., Frolking, S., and Frolking, T. A. (1992). A model of nitrous oxide evolution from soil driven by rainfall events: 1. Model structure and sensitivity. J. Geophys. Res. 97, 9759–9776. doi:10.1029/92JD00509
Mang, H. P., and Li, Z. (2010). Technology Review of Biogas Sanitation. Available at: http://www.sswm.info/sites/default/files/reference_attachments/technology%20review%20of%20biogas%20sanitation.pdf
Manitoba Government. (2014). Agriculture, Food and Rural Development. Available at: http://www.gov.mb.ca/agriculture/environment/soil-management/soil-management-guide/nutrient-management.html
Minnesota Project. (2014). Profits from Manure Power? The Economics of Anaerobic Digesters On-Farm. Available at. http://www.agmrc.org/media/cms/AD_economics_232C193A1CA1B.pdf
Moller, K. (2009). Influence of different manuring systems with and without biogas digestion on soil organic matter and nitrogen inputs, flows and budgets in organic cropping systems. Nutr. Cycl. Agroecosys. 84, 179–202. doi:10.1007/s10705-008-9236-5
Möller, K., and Stinner, W. (2009). Effects of different manuring systems with and without biogas digestion on soil mineral nitrogen content and on gaseous nitrogen losses (ammonia, nitrous oxides). Eur. J. Agron. 30, 1–16. doi:10.1016/j.eja.2008.06.003
Mu, S. J., Zeng, Y., Wu, P., Lou, S. J., and Tartakovsky, B. (2008). Anaerobic digestion model no. 1-based distributed parameter model of an anaerobic reactor. I. Model development. Bioresour. Technol. 99, 3665–3675. doi:10.1016/j.biortech.2007.07.060
Mwirigi, J. W., Makenzi, P. M., and Ochola, W. O. (2009). Socio-economic constraints to adoption and sustainability of biogas technology by farmers in Nakuru Districts, Kenya. Energy Sustain. Dev. 13, 106–115. doi:10.1016/j.esd.2009.05.002
NSCA. (2006). Biogas as a Road Transport Fuel – An Assessment of the Potential Role of Biogas as a Renewable Transport Fuel. Available at: http://www.cleanvehicle.eu/fileadmin/downloads/UK/nsca_biogas_as_a_road_transport__084926300_1011_24042007.pdf
Panyadee, S., Petiraksakul, A., and Phalakornkule, C. (2013). Biogas production from co-digestion of Phyllanthus emblica residues and food waste. Energy Sustain. Dev. 17, 515–520. doi:10.1016/j.esd.2013.07.003
Patterson, T., Esteves, S., Dinsdale, R., and Guwy, A. (2011). Life cycle assessment of biogas infrastructure options on a regional scale. Bioresour. Technol. 102, 7313–7323. doi:10.1016/j.biortech.2011.04.063
Petersen, S. O., Sommer, S. G., Béline, F., Burton, C., Dach, J., Dourmad, J. Y., et al. (2007). Recycling of livestock manure in a whole-farm perspective. Livest. Sci. 112, 180–191. doi:10.1016/j.livsci.2007.09.001
Pöschl, M., Ward, S., and Owende, P. (2010). Evaluation of energy efficiency of various biogas production and utilization. Appl. Energy 97, 3305–3321. doi:10.1016/j.apenergy.2010.05.011
Regina, K., and Perälä, P. (2006). “Ammonia and greenhouse gas emissions from pig slurry – the effect of slurry fermentation, separation of the fermentation product and application technique,” in 12th RAMIRAN International Conference on Technology for Recycling of Manure and Organic Residues in a Whole Farm Perspective, ed. S. O. Petersen (Denmark: Danish Institute of Agricultural Sciences), Vol. II, 241–243. DIAS report Plant production no. 123.
Risberg, K., Sun, L., Levén, L., Horn, S. J., and Schnürer, A. (2013). Biogas production from wheat straw and manure – impact of pretreatment and process operating parameters. Bioresour. Technol. 149, 232–237. doi:10.1016/j.biortech.2013.09.054
Safley, L. M. Jr., and Westerman, P. W. (1994). Low-temperature digestion of dairy and swine manure. Bioresour. Technol. 47, 165–171. doi:10.1016/0960-8524(94)90116-3
Sanchez, M., Gomez, X., Barriocanal, G., Cuetos, M. J., and Moran, A. (2008). Assessment of the stability of livestock farm wastes treated by anaerobic digestion. Int. Biodeterior. Biodegradation 62, 421–426. doi:10.1016/j.ibiod.2008.04.002
Santosh, Y., Sree, K. T. R., Kohli, S., and Rana, V. (2004). Enhancement of biogas production from solid substrates using different techniques – a review. Bioresour. Technol. 95, 1–10. doi:10.1016/j.biortech.2004.02.010
Schievano, A., D’Imporzano, G., Salati, S., and Adani, F. (2011). On-field study of anaerobic digestion full-scale plants (part I): an on-field methodology to determine mass, carbon and nutrients balance. Bioresour. Technol. 102, 7737–7744. doi:10.1016/j.biortech.2011.06.006
Searchinger, T., Heimlich, R., Houghton, R. A., Dong, F. X., Elobeid, A., Fabiosa, J., et al. (2008). Use of U.S. croplands for biofuels increases greenhouse gases through emissions from land use change. Science 319, 1238–1240. doi:10.1126/science.1151861
Smith, K., Cumby, T., Lapworth, J., Misselbrook, T., and Williams, A. (2007). Natural crusting of slurry storage as an abatement measure for ammonia emissions on dairy farms. Biosys. Eng. 97, 464–471. doi:10.1016/j.biosystemseng.2007.03.037
Statistics Canada. (2012). Cattle Statistics. Available at: http://www.statcan.gc.ca/pub/23-012-x/23-012-x2011002-eng.pdf
Sung, S., and Liu, T. (2003). Ammonia inhibition on thermophilic anaerobic digestion. Chemosphere 53, 43–52. doi:10.1016/S0045-6535(03)00434-X
Swisher, K. J. (2006). “The global market for rendering products,” in Essential Rendering: All about the Animal By-products Industry (ISBN: 0965466035), ed. D. L. Meeker (Virginia: Kirby Lithographic Company, Inc.), 213–228.
Taheripour, F., Hertel, T., Tyner, W. E., and Beckman, J. F. (2010). Biofuels and their by-products: global economic and environmental implications. Biomass Bioeng. 34, 278–289. doi:10.1016/j.biombioe.2009.10.017
Tambone, F., Scaglia, B., D’Imporzano, G., Schievano, A., Orzi, V., Salati, S., et al. (2010). Assessing amendment and fertilizing properties of digestates from anaerobic digestion through a comparative study with digested sludge and compost. Chemosphere 81, 577–583. doi:10.1016/j.chemosphere.2010.08.034
Tomei, M. C., Braguglia, C. M., Cento, G., and Mininni, G. (2009). Modeling of anaerobic digestion of sludge. Crit. Rev. Environ. Sci. Technol. 39, 1003–1051. doi:10.1080/10643380801977818
Tonini, D., Martinez-Sanchez, V., and Astrup, T. F. (2013). Material resources, energy, and nutrient recovery from waste: are waste refineries the solution for the future? Environ. Sci. Technol. 47, 8962–8969. doi:10.1021/es400998y
Vaneeckhaute, C., Meers, E., Michels, E., Ghekiere, G., Accoe, F., and Tack, F. M. G. (2013). Closing the nutrient cycle by using bio-digestion waste derivatives as synthetic fertilizer substitutes: a field experiment. Biomass Bioenergy 55, 175–189. doi:10.1016/j.biombioe.2013.01.032
Wang, J. Y., Cardenas, L. M., Misselbrook, T. H., Cuttle, S., Thorman, R. E., and Li, C. S. (2012). Modelling nitrous oxide emissions in grazed grassland systems. Environ. Pollut. 162, 223–233. doi:10.1016/j.envpol.2011.11.027
Wang, J. Y., Hobbs, P., Chadwick, D., and Butler, A. (2011). Modelling of N2O Emissions of Biogas Digestate for Use as Fertiliser Using DNDC. London: Agricultural Research Modellers’ Group.
Yang, Y. L., Zhang, P. D., and Li, G. Q. (2012). Regional differentiation of biogas industrial development in China. Renew. Sustain. Energy Rev. 16, 6686–6693. doi:10.1016/j.rser.2012.07.016
Zheng, Y. H., Li, Z. F., Feng, S. F., Lucas, M., Wu, G. L., Li, Y., et al. (2010). Biomass energy utilization in rural areas may contribute to alleviating energy crisis and global warming: a case study in a typical agro-village of Shandong, China. Renew. Sustain. Energy Rev. 14, 3132–3139. doi:10.1016/j.rser.2010.07.052
Keywords: biogas, digestive system, anaerobic digestion, waste management, greenhouse gas emissions, crop residues, digestate, digestate used as fertilizer
Citation: Wang J (2014) Decentralized biogas technology of anaerobic digestion and farm ecosystem: opportunities and challenges. Front. Energy Res. 2:10. doi: 10.3389/fenrg.2014.00010
Received: 16 December 2013; Accepted: 21 February 2014;
Published online: 26 March 2014.
Edited by:
Jalel Labidi, University of the Basque Country, SpainReviewed by:
Subba Rao Chaganti, University of Windsor, CanadaMaria Gonzalez Alriols, University of the Basque Country, Spain
Copyright: © 2014 Wang. This is an open-access article distributed under the terms of the Creative Commons Attribution License (CC BY). The use, distribution or reproduction in other forums is permitted, provided the original author(s) or licensor are credited and that the original publication in this journal is cited, in accordance with accepted academic practice. No use, distribution or reproduction is permitted which does not comply with these terms.
*Correspondence: Junye Wang, Faculty of Science and Technology, Athabasca University, 1 University Drive, Athabasca, AB T9S 3A3, Canada e-mail:anVueWV3QGF0aGFiYXNjYXUuY2E=