- 1Department of Endocrinology, Shengjing Hospital of China Medical University, Shenyang, China
- 2Department of Pediatrics, Shengjing Hospital of China Medical University, Shenyang, China
Patients with type 2 diabetes mellitus (T2DM) have a 2 to 3 times higher risk of cardiovascular disease compared to non-diabetic individuals, and cardiovascular disease has consistently been a leading cause of death among diabetic patients. Therefore, preventing cardiovascular disease in diabetic patients remains a significant challenge. In addition to classic indicators such as cholesterol and lipoproteins, previous studies have demonstrated that plasma level of free fatty acid (FFA) is closely related to the occurrence of atherosclerosis, particularly in T2DM patients. In recent years, with further research and advancements in testing technologies, the FFA profile has garnered widespread attention. The FFA profile includes many different types of FFAs, and changes in the plasma FFA profile and concentrations in T2DM patients may lead to the development of insulin resistance, causing damage to vascular endothelial cells and promoting the occurrence and progression of atherosclerosis. Furthermore, some FFAs have shown potential in predicting cardiovascular complications in T2DM and are associated with the severity of these complications. Here, we aim to review the changes in the FFA profile in T2DM and discuss the relationship between the FFA profile and the occurrence of vascular complications in T2DM.
1 Introduction
Diabetes is a chronic metabolic disorder characterized by insufficient insulin secretion and/or insulin resistance caused by environmental and genetic factors. The prevalence of diabetes is increasing year by year. There are 537 million adults worldwide living with diabetes, and by 2045, the prevalence of diabetes is expected to increase by 16%, affecting 783 million people globally (1). Diabetes is associated with many medical complications, with cardiovascular diseases remaining the leading cause of death and disability among diabetes patients, especially in those with type 2 diabetes mellitus (T2DM) (2). T2DM accounts for more than 90% of diabetes cases (3), and its most common and severe complication is coronary heart disease, which accounts for about two-thirds of deaths in T2DM patients (4, 5).
Diabetes is a group of carbohydrate metabolism disorders characterized by chronic hyperglycemia, resulting from insufficient insulin secretion, defective action, or both. Insulin deficiency can lead to various metabolic abnormalities in proteins, lipids, and carbohydrates. Coronary artery disease primarily progresses due to atherosclerosis, manifested as lesions in the intima of the arterial wall and plaque accumulation, which narrows the lumen and leads to ischemia and metabolic changes in the tissues supplied by the affected arteries. Subsequent erosion and rupture of atherosclerotic plaques can trigger thrombotic events, posing a risk of fatality. Many studies have investigated the potential link between these two chronic diseases (6–9), and the results indicate that diabetes itself can induce the formation of atherosclerotic plaques or further accelerate their development. Elevated blood glucose levels, dyslipidemia, and other metabolic changes associated with the progression of comorbidities are closely related to the pathogenesis of atherosclerosis at nearly every step of the process. In addition to classic risk factors such as cholesterol and lipoproteins, previous studies have demonstrated that level of free fatty acid (FFA) is closely associated with the occurrence of atherosclerosis, particularly in patients with T2DM.
FFA is an important energy substrate for the human body and is involved in many metabolic regulations (10). FFA mainly originates from adipose tissue, released through the breakdown of triglycerides, and is primarily carried in the blood by serum albumin (11). FFA includes saturated fatty acids (SFAs) and unsaturated fatty acids (UFAs), with the latter further divided into monounsaturated fatty acids (MUFAs) and polyunsaturated fatty acids (PUFAs). They perform different functions due to their structural differences. The FFA profile can be regarded as a collective term for different types of FFAs. An important analytical method for detecting and analyzing the FFA profile is liquid chromatography-tandem mass spectrometry (LC-MS/MS), which has advantages over traditional immunoassays and spectroscopic methods in terms of high throughput, high accuracy, high sensitivity, and high specificity. FFA level is associated with insulin resistance and the development of diabetes mellitus (12–14). Higher FFA level can predict the occurrence and severity of atherosclerotic plaques in patients with T2DM (15–19), and may also be related to the prognosis of patients with coronary heart disease (20). Previous studies on the atherosclerosis risk in diabetic patients have focused more on the total level of FFA, but there is less research on the specific composition and concentration changes of FFAs. Circulating FFA concentrations depend not only on dietary intake but also on endogenous synthesis and metabolism. Estimating individual FFA quality intake through dietary assessment is relatively difficult, and measuring circulating FFAs as biomarkers is considered more reliable compared to self-reported assessments of fatty acid intake (104). In recent years, with further research and advancements in testing technology, the FFA profile has attracted widespread attention. The FFA profile contains many different types of FFAs. The total plasma FFA level in patients with T2DM are often elevated, with the most commonly observed increase in their FFA profile being SFA (especially palmitic acid, C16:0) (21). C16:0 is the most abundant fatty acid in the human body, and its abnormal increase within cells can weaken insulin signaling, leading to the development of insulin resistance (22). Insulin resistance alters systemic lipid metabolism, resulting in high levels of triglycerides, low levels of high-density lipoprotein, and the presence of small, dense low-density lipoproteins. These lipid changes, along with endothelial dysfunction induced by abnormal insulin signaling, contribute to the formation of atherosclerotic plaques (23). Although the exact mechanisms behind the changes in blood FFA profiles in diabetic patients are not yet clear, it is believed that they may be related to changes in dietary fats and lipid metabolism (24). Some FFAs have shown potential in predicting cardiovascular complications in T2DM and are associated with the severity of these complications. Here, we aim to review the changes in the FFA profile in T2DM and discuss the relationship between the FFA profile and the occurrence of vascular complications in T2DM.
2 The sources and classification of FFA
Fatty acids (FAs) mainly exist in three ester forms in living organisms: triglycerides, phospholipids, and cholesterol esters. When FAs are not in ester form in plasma, they are referred to as non-esterified FAs or FFAs. FFAs are classified based on their saturation into SFAs and UFAs. SFAs mainly include stearic acid (C18:0) and palmitic acid (C16:0). UFAs are further divided into MUFAs and PUFAs based on the number of double bonds. The MUFAs present in the human body mainly include palmitoleic acid (C16:1) and oleic acid (C18:1), while the PUFAs mainly include linoleic acid (C18:2), alpha-linolenic acid (C18:3), arachidonic acid (C20:4), and docosahexaenoic acid (C22:6), each having different functions and physiological effects. Omega-3 fatty acids and omega-6 fatty acids both belong to PUFAs, and their distinction lies in the position and distance of the double bond from the methyl end of the fatty acid molecule.
SFAs mainly come from animal fats, coconut oil, and palm oil. MUFAs are primarily sourced from tea seed oil and olive oil. Long-chain omega-3 fatty acids, eicosapentaenoic acid (EPA), and docosahexaenoic acid (DHA) are found in fish oil, flaxseed oil, supplements, and concentrated pharmaceutical preparations. Omega-6 fatty acids mainly come from soybean oil, peanut oil, sesame oil, and others.
3 FFA profile and T2DM
3.1 How FFA affects insulin resistance and glucose metabolism
β-cell dysfunction and insulin resistance are fundamental characteristics of T2DM, thus studying the pathogenic mechanisms that lead to these two defects is an important avenue for researching the pathogenesis of T2DM. FFAs are closely related to both pathways. The long-term elevated glucose levels lead to glucotoxicity, which impairs the function of pancreatic β-cells. This is specifically manifested as glucose desensitization, cellular exhaustion, and chronic progressive irreversible damage to β-cells. Excessive systemic FFA and dietary lipids enter non-adipose organs such as the liver, muscle, and pancreas, accumulating in the form of ectopic fat, resulting in lipotoxicity. Toxic lipids disrupt organelles, such as mitochondria, endoplasmic reticulum, and lysosomes. Disrupted organelles release excessive reactive oxygen species (ROS) and pro-inflammatory factors, leading to systemic inflammation. Prolonged low-grade systemic inflammation can hinder insulin’s action in the insulin signaling pathway, disrupt glucose homeostasis, and lead to systemic dysregulation (25). Insulin signaling molecules are also present in β-cells, where they play a role in β-cell function. Lipotoxicity causes a weakening of the insulin signaling pathway, which can result in insulin resistance in β-cells, making it one of the important mechanisms leading to β-cell dysfunction (26). The combination of glucotoxicity and lipotoxicity, known as glucolipotoxicity, is considered particularly harmful to the quality and function of β-cells (27).However, whether the elevated levels of FFAs during the progression to diabetes are pathogenic remains unproven (28).
The harmful effects of lipotoxicity are mediated by SFAs (mainly C16:0). C16:0 is the most abundant fatty acid in the human body. An abnormal increase of C16:0 within cells, exceeding the oxidative capacity of mitochondria, may lead to the conversion into harmful complex fatty acid-derived lipids, such as diacylglycerol (DAG) and ceramides. DAG activates protein kinase C (PKC), which primarily weakens the insulin signaling pathway by phosphorylating the serine residues of insulin receptor substrate (IRS-1) (22). Ceramides act as mediators of programmed cell death and are involved in the apoptosis of pancreatic β cells. Ceramides also interfere with the expression of IRS-1 and phosphoinositide 3-kinase and protein kinase B (PKB or AKT), further weakening insulin signal transduction (29). Additionally, excessive C16:0 can impair the function of organelles (endoplasmic reticulum and mitochondria) and promote the activation of pro-inflammatory transcription factors (such as NF-kB), triggering chronic inflammation (22). UFAs can mitigate the harmful effects of SFAs to some extent by increasing the mitochondrial oxidation of SFAs and promoting their accumulation in the form of triacylglycerol (TAG) (30).
High levels of FFAs are associated with insulin resistance and can even lead to it. As early as 1963, Randle et al. suggested a correlation between elevated blood FFAs and insulin resistance, proposing the glucose-fatty acid cycle theory. They demonstrated that the oxidation of blood FFAs increases the acetyl CoA content in muscles, and the allosteric inhibition of pyruvate dehydrogenase activity by acetyl CoA reduces glucose oxidation (31). Subsequent research has shown that the aforementioned lipotoxic effects caused by excessive lipid production can directly affect intracellular signaling pathways. For example, in the gastrocnemius muscle of rats fed a high-fat diet, the level of PKC increased, leading to a weakening of insulin signaling (32). A recent clinical case-control study found that total FFA and SFA increased the homeostatic model assessment of insulin resistance (HOMA-IR), thereby increasing the risk of developing T2DM (33).
3.2 Changes in FFA profile in T2DM
In patients with T2DM, the plasma total FFA level is elevated (34, 35). The most commonly observed pattern in T2DM patients is the increase in SFAs, especially C16:0 (21). Impaired insulin secretion, reduced insulin sensitivity, and poor glucose tolerance are closely related to the increased plasma FFA level, particularly SFAs, including C16:0 and C18:0 (36). Additionally, in T2DM patients, the level of palmitic acid is positively correlated with the level of glycosylated hemoglobin (HbA1c), while in patients with poor diabetes control, the level of oleic acid among MUFAs is only correlated with HbA1c levels (37).
The correlation between plasma FFA and T2DM varies depending on the type of fatty acid. A large cohort study involving 95,854 participants indicates that plasma SFAs and MUFAs concentrations are associated with a higher risk of T2DM, while plasma omega-3 PUFA and omega-6 PUFA are associated with a lower risk (38). Even within the same category of fatty acids, such as SFAs or UFAs, the correlation of specific individual fatty acids with T2DM can differ. Systematic reviews and large cohort studies indicate that an increase in odd-chain SFAs is associated with a reduced risk of T2DM events, while an increase in even-chain SFAs is associated with an increased risk of T2DM events (39, 40). A large-scale study measuring plasma phospholipid PUFA in 12,132 new cases of T2DM and 15,919 control participants indicated a significant negative correlation with plant-derived omega-3 PUFA (alpha-linolenic acid, ALA), while no significant association was found between marine-derived omega-3 PUFAs (EPA and DHA) and T2DM (41), although there are opposing viewpoints (42). According to a recent meta-analysis of prospective cohort studies, high dietary intake of linoleic acid (LA, 18:2n-6) and increased levels of LA in the body are significantly associated with a reduced risk of T2DM (43).
4 FFA profile and T2DM complicated by coronary heart disease
4.1 Mechanisms of FFA profile inducing atherosclerosis
Endothelial dysfunction (ED) is a key event in the pathogenesis of diabetes-related atherosclerosis and vascular complications (44). Excessive FFA have been shown to lead to endothelial dysfunction, vascular hypertrophy, and vascular wall stiffness, all of which are important triggers for hypertension and atherosclerosis (45). FFA-mediated endothelial dysfunction involves multiple mechanisms, including impaired insulin signaling and nitric oxide production, oxidative stress, inflammation, activation of the renin-angiotensin system, and endothelial cell apoptosis (46).
NO is a vasodilator released by endothelial cells, and impaired production of NO is a major characteristic of ED. Elevated FFA promote insulin resistance, inhibiting the insulin signaling pathway PI3K/Akt, which leads to a reduction in NO production regulated by this pathway (46). High concentrations of FFA cause dysfunction in the electron transport chain of mitochondria, increasing the generation of reactive oxygen species (ROS) and inducing oxidative stress in the body, which can lead to apoptosis of endothelial cells (47). In healthy subjects, FFA-induced vascular risk factors can be observed, characterized by elevated levels of endothelial markers (18). Additionally, FFA also induces inflammation through the activation of NF-κB (48).
Several factors can affect plasma FFA level; for instance, intense physical activity or emotional fluctuations under physiological conditions can temporarily elevate FFA level, which is a natural response of the body to meet energy demands. Additionally, certain lifestyle and clinical factors, such as obesity, chronic sleep deprivation, sleep apnea, and smoking, can increase FFA level. Conversely, weight loss, regular exercise, and treatment of sleep apnea can lower FFA level, potentially alleviating the adverse effects caused by elevated FFA (49). Figure 1 summarizes the mechanisms by which FFA induce atherosclerosis and the factors influencing FFA level.
Researchers believe that long-chain omega-3 fatty acids are beneficial for cardiovascular health, including EPA and DHA, which mainly come from oily fish, as well as alpha-linolenic acid (ALA), which mainly comes from plants (50). Increased consumption of EPA and DHA, or elevated levels of them, is linked to a reduced risk of cardiovascular diseases, especially coronary heart disease, and lower rates of cardiovascular-related deaths (51–54).
4.2 The role of FFA profile in T2DM complicated by coronary heart disease
In the population with T2DM, previous studies have focused more on the relationship between the total level of FFA and cardiovascular diseases, while research on FFA profiles has been limited. In Table 1, we list the characteristics of studies and survey populations regarding FFA and FFA profiles, while specific changes in FFA profiles are detailed in Table 2. Total FFA level is considered a risk factor for the development of coronary heart disease and other arterial vascular lesions in T2DM, with previous research results being consistent (15, 19, 56, 58–61). In addition, high level of FFA indicate a poor prognosis for coronary heart disease (20), suggesting a more severe condition (16, 19, 61). The findings concerning FFA profiles differ, which can be attributed to some factors, such as the ethnic backgrounds of the study groups, differences in the chosen control groups, the necessity of fasting prior to blood sampling, and whether the results are shown as absolute concentrations or as a percentage of the total plasma FFA measured.
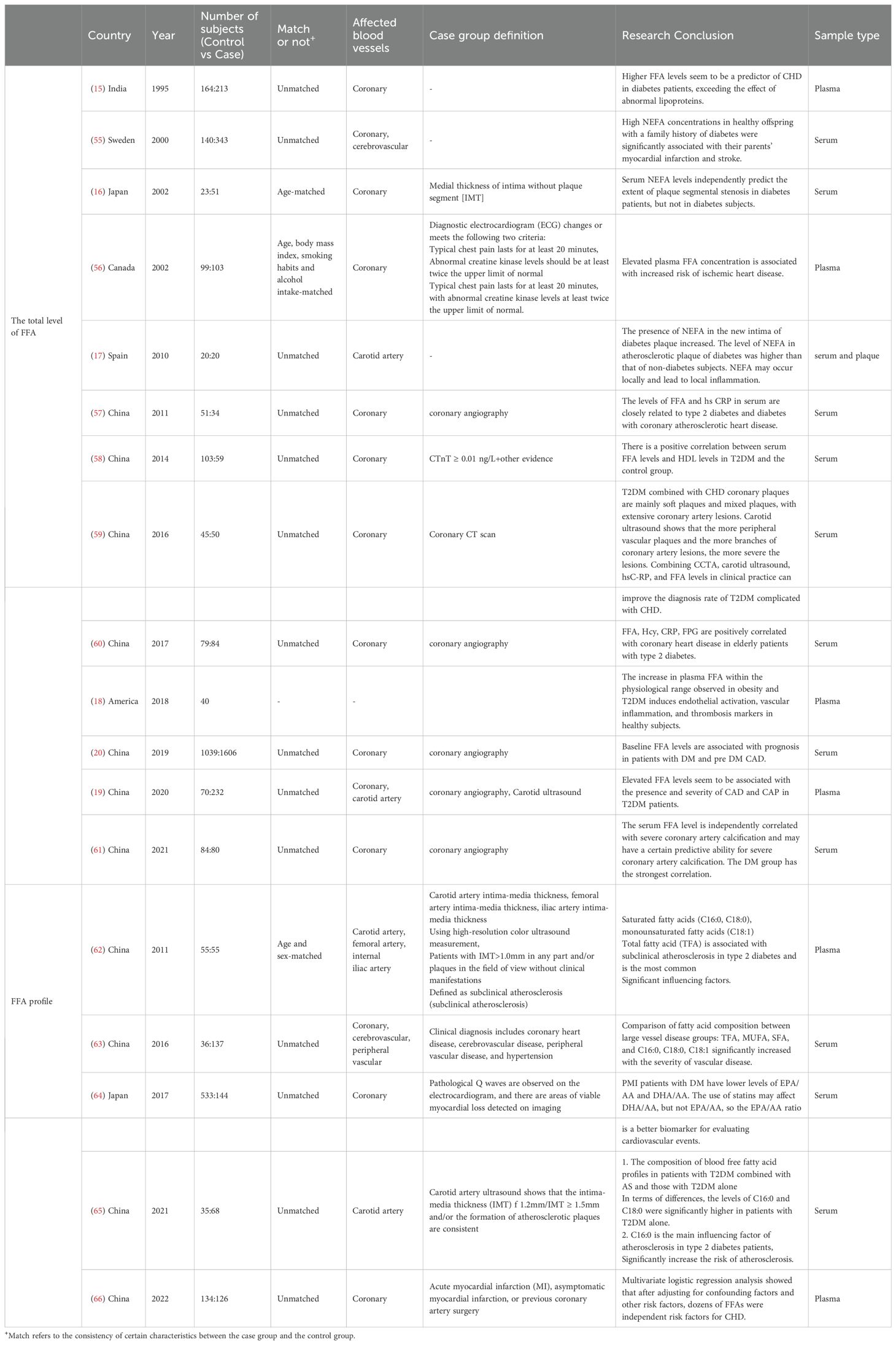
Table 1. Studies on population characteristics and blood concentrations of individual FFA species in cardiovascular subjects and controls of patients with T2DM.
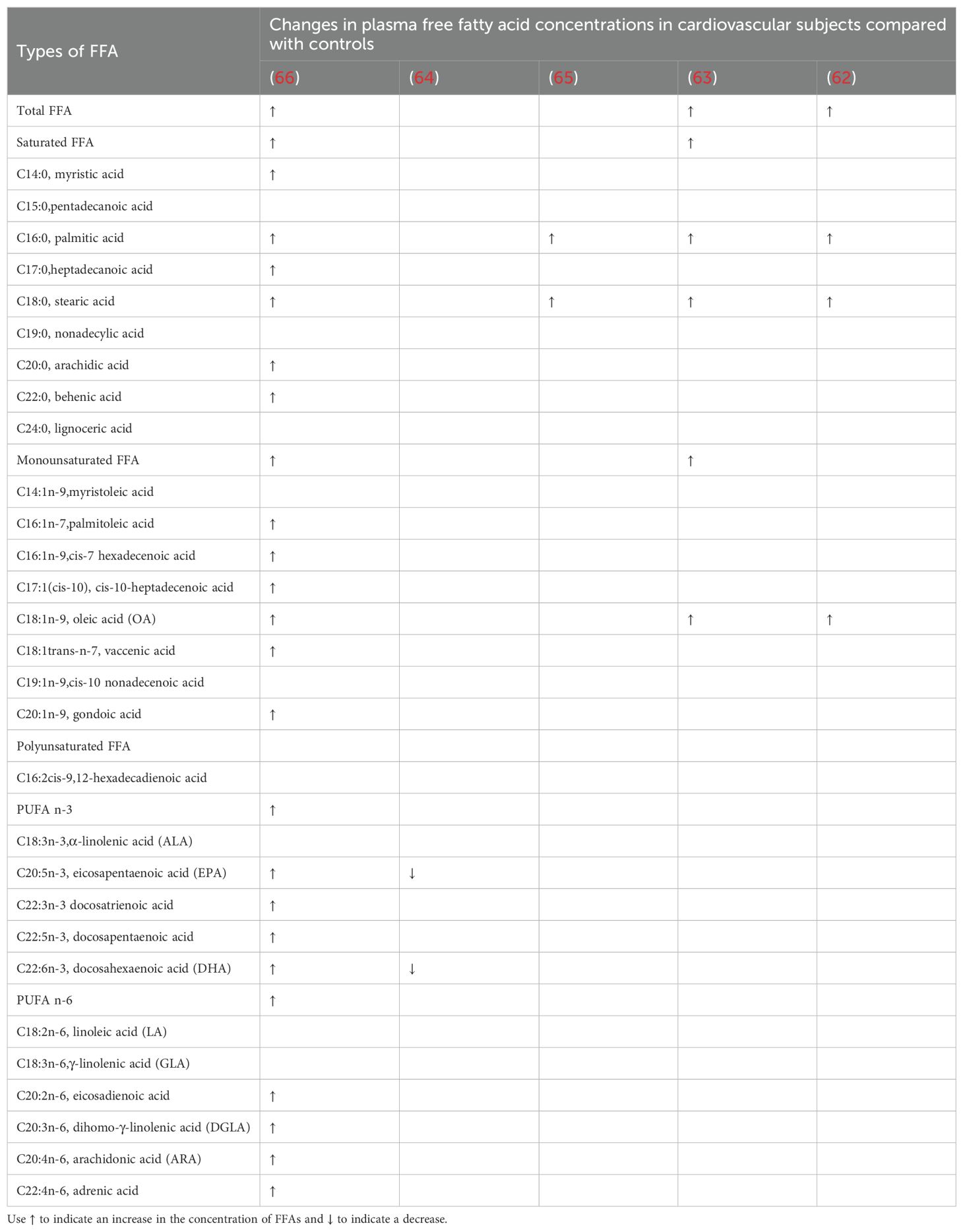
Table 2. Changes in the concentration of FFAs in the blood of cardiovascular subjects and controls of patients with T2DM.
In T2DM, the levels of C16:0 and C18:0 in the blood are significantly increased in patients with concurrent cardiovascular disease, as noted in multiple studies (62, 63, 65, 66). This is consistent with previous research, as C16:0, the most abundant SFA in the human body, is associated with an increased cardiovascular risk in T2DM patients (67). A 2017 multicenter cross-sectional study from Japan only assessed the association between PUFA levels and risk factors in patients with prior myocardial infarction, finding that the levels of C20:5 (EPA) and C22:6 (DHA) were lower in the DM group with coronary heart disease, which aligns with findings from numerous previous studies (68). Additionally, this study had a larger sample size compared to four others and excluded subjects taking omega-3 PUFA supplements, which may have led to more accurate measurements. The research conducted by Hu et al. analyzed the plasma FFA profile of 134 patients with T2DM and 126 patients with T2DM complicated by coronary heart disease (CHD). The results indicated that nearly all types of FFAs, regardless of their carbon chain length or unsaturation, were significantly elevated in the plasma of patients with CHD-T2DM compared to those with uncomplicated T2DM. Additionally, the study highlighted differences in the levels of omega-3 fatty acids, specifically C20:5 (EPA) and C22:6 (DHA), when compared to earlier research findings (66). The reasons for the differences may be related to the discrepancies found in previous randomized controlled trials. Although numerous epidemiological and clinical studies have indicated that supplementation with Omega-3 PUFAs is associated with reduced inflammation, the levels of Omega-3 PUFAs are generally lower in patients with cardiovascular diseases (68). However, there are also large randomized controlled trials that show that supplementation with Omega-3 fatty acids does not reduce the incidence of cardiovascular events in high-risk patients with T2DM (69). Based on the above research, the changes in the concentrations of FFAs associated with T2DM are complex and require further studies.
4.3 The relationship between dietary intake of fatty acids and T2DM complicated by coronary heart disease
As early as the 1970s, epidemiological studies of Greenland Eskimos linked their diet, rich in omega-3 PUFAs from fish and fish oil, to low cardiovascular mortality and effective treatment of hypertriglyceridemia (70). Subsequently, the health benefits of fish oil and its content of omega-3 long-chain PUFAs (omega-3 LC-PUFAs) have garnered widespread attention in the scientific community over the past four decades. Fish oil contains high levels of but variable omega-3 LC-PUFAs, including EPA and DHA, as well as small amounts of docosapentaenoic acid (DPA; 22:5n-3) (71). However, the use of omega-3 PUFAs for the prevention of coronary heart disease remains controversial. The data results from classic large-scale RCT studies show that, supplementation with larger doses of pure EPA demonstrates cardiovascular benefits (72, 73), while supplementation with a mixture of EPA and DHA yields negative results (74, 75). The types of fish oil prescription drugs (pure EPA or EPA+DHA mixture) and dosage differences may be important factors affecting clinical outcomes. In addition to marine derived omega-3 LC-PUFA, plants synthesize short chain omega-3 fatty acids such as alpha linolenic acid (ALA; 18:3, n-3) and linoleic acid (18:2, n-6), which are present in many seeds, nuts, and seed oils (76). The sustained effects of ALA intake on cardiovascular health also exhibit heterogeneity (50, 77), which may be related to the dosage used (78).
Multiple large systematic reviews indicate that the Mediterranean diet, which is rich in PUFAs and MUFAs, can improve blood glucose and lipid levels in patients with T2DM (79, 80). However, the evidence does not support the recommendation for all diabetes patients to take EPA and DHA supplements to prevent or treat cardiovascular events. In the ASCEND trial (81), supplementation with omega-3 fatty acids (EPA, DHA) at a dose of 1 g/day did not provide cardiovascular benefits compared to placebo in diabetic patients without evidence of cardiovascular disease. However, the results of the REDUCE-IT study found that supplementation with 4 g/day of pure EPA significantly reduced the risk of adverse cardiovascular events. This trial involved 8,179 participants, more than 50% of whom had diabetes. In patients with cardiovascular disease who were receiving statin treatment, had achieved low-density lipoprotein cholesterol targets, and had elevated triglyceride levels (135-499 mg/dL), there was an absolute reduction of 5% in cardiovascular events (82). Figure 2 shows the association between dietary intake of fatty acids and T2DM with coronary heart disease.
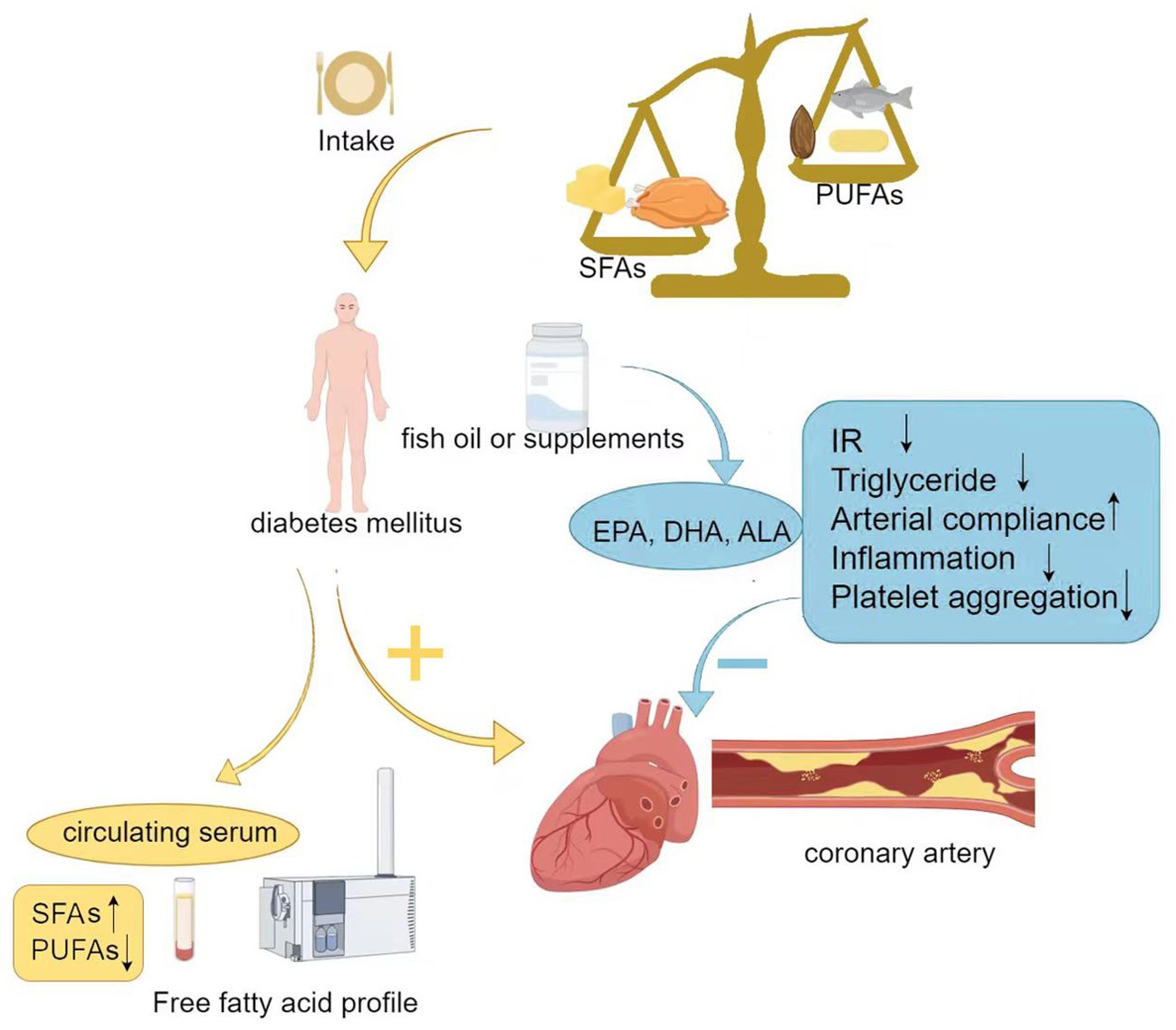
Figure 2. Association between dietary intake of fatty acids and T2DM with coronary heart disease. SFAs, Saturated fatty acids; PUFAs, Polyunsaturated fatty acids; DHA, Docosahexaenoic acid; EPA, Eicosapentaenoic acid; ALA, α-Linolenic acid; IR, Insulin resistance.
Many countries’ dietary guidelines advocate for limiting the intake of SFAs, especially for individuals with clinical atherosclerotic cardiovascular disease (ASCVD), dyslipidemia, or diabetes, such as in China, Europe, and the United States (83–86). The results of observational studies indicate that dietary patterns with lower average SFA intake are associated with good cardiovascular outcomes (87). In addition, although the number of randomized controlled trials testing the impact of reducing SFAs intake on ASCVD outcomes is limited, existing evidence supports the view that replacing SFAs with UFAs, especially PUFAs, may reduce the risk of ASCVD (88). In addition to increasing the concentration of LDL-C and atherogenic lipoprotein particles, higher SFAs intake may also affect the pathways that affect inflammation, rhythm, hemostasis, apolipoprotein CIII production and high-density lipoprotein function (87). However, the impact of these effects on ASCVD risk remains uncertain.
As the most abundant saturated fatty acid in the human body, C16:0 has a higher content in palm oil, animal fat, and cocoa butter. A recent study that combines human cohort studies and animal models has identified key mechanistic pathways through which C16:0 reduces plaque stability, which is associated with increased cardiovascular risk in patients with T2DM (67). And C18:1 oleic acid, one of the MUFAs, may have a protective effect on T2DM (89), while also reducing the proportion of low-density lipoprotein cholesterol and total cholesterol, and lowering cardiovascular risk. C18:1 reduces insulin resistance by increasing the mitochondrial oxidation of SFAs and promoting their accumulation in the form of TAG, thereby decreasing the synthesis of DAG and ceramides (22).
Currently, there is no unified conclusion regarding the optimal daily total fat energy ratio for patients with T2DM. The 2020 CDS guidelines recommend that the energy provided by fat in the diet should account for 20% to 30% of total energy (90). If the fat is of high quality (such as MUFAs and ω-3 PUFAs), the fat energy ratio can be increased to 35%. It is advised to limit the intake of SFAs and trans fatty acids as much as possible.
4.4 The relationship between obesity and T2DM, coronary heart disease
Obesity is a chronic positive energy balance disease caused by a complex interaction between abnormal neurohumoral responses and an individual’s socioeconomic, environmental, behavioral, and genetic factors, leading to a state of chronic inflammation. The World Health Organization defines individuals with a body mass index (BMI) >25 kg/m² as overweight and those with a BMI >30 kg/m² as obese. Obesity directly or indirectly increases the incidence and mortality of cardiovascular disease (CVD). The direct effects mainly include two aspects: one is the structural and functional adaptations of the cardiovascular system induced by obesity (91). The second is that adipocytes synthesize and secrete a large number of proteins and hormones known as adipokines, which play important roles in endocrine regulation, immune function, and inflammation. In obesity, adipose tissue undergoes maladaptive expansion, leading to impaired secretion of adipokines and an imbalance of adipokines. There is an increase in pro-inflammatory adipokines and a decrease in anti-inflammatory adipokines, resulting in the development of a chronic, low-grade inflammatory state that negatively impacts vascular homeostasis (92). Additionally, abnormal fat deposition, epicardial adipose tissue, and non-alcoholic fatty liver disease are also pathophysiological factors through which obesity leads to or exacerbates cardiovascular diseases (93). The indirect effects are that obesity directly leads to the development of diabetes, hypertension, and hyperlipidemia, which are also risk factors for CVD.
Obese patients who consume excessive calories for a long time lead to an excess storage of fat, surpassing the limited storage capacity of adipose tissue for fatty acids, which results in an increase in circulating FFAs. The lipotoxicity of fatty acids in circulation and their storage in key metabolic regulatory organs leads to systemic oxidative stress, inflammation, and metabolic dysregulation. FFA can cause insulin resistance in all major insulin target organs (skeletal muscle, liver, endothelial cells) (94) and has become a major link between obesity, T2DM, and atherosclerotic vascular diseases. Figure 3 illustrates the connection between them.
4.5 The relationship among statins, FFA, type 2 diabetes, and coronary heart disease
Elevated plasma FFA level associated with T2DM can lead to various pathologies, making it clinically important to control their concentrations. In addition to antidiabetic medications, lipid-lowering drugs are also used for the management of T2DM. However, most of these lipid-lowering drugs primarily control plasma cholesterol, LDL, and HDL levels rather than FFA level. There is evidence that statins (used for coronary artery disease) have anti-inflammatory effects and may also impact FFA level, potentially reducing plasma FFA concentrations (95).
Dyslipidemia in diabetes is characterized by elevated fasting and postprandial triglycerides, low levels of high-density lipoprotein cholesterol, elevated low-density lipoprotein cholesterol, and a predominance of small, dense low-density lipoprotein particles. Lifestyle changes and blood glucose control may improve lipid levels, but lowering LDL is the cornerstone of treating diabetic dyslipidemia, with statins being the primary therapeutic agents (96). They have beneficial effects on dyslipidemia associated with coronary artery disease (97). Therefore, most diabetic patients should receive statin therapy (98). However, it is worth noting that statins seem to have negative effects on non-diabetic patients, with a higher risk of developing new-onset T2DM (99).
5 The potential of FFA as biomarkers
Changes in FFA are more sensitive than changes in triglycerides and cholesterol esters in reflecting the body’s lipid metabolism (100). Circulating FFA levels are closely related to insulin sensitivity and the decline of β-cell function (101). Serum FFA levels are useful biomarkers for improving the management of patients with T2DM, as they can reflect the severity of coronary artery disease (CAD) and carotid atherosclerotic plaques (CAP) in T2DM patients (19). Additionally, FFA is closely associated with metabolic syndrome (MS) and is a predictor of non-alcoholic fatty liver disease (NAFLD) (102). In patients with high fasting blood glucose, a decrease in arachidonic acid (AA) and an increase in the EPA/AA ratio have been observed. This suggests that they have the potential to serve as biomarkers for blood glucose control (103).
6 Conclusion and future directions
Elevated levels of FFAs in plasma are associated with the presence and severity of cardiovascular events in patients with T2DM. However, previous studies have primarily focused on the total FFA level in plasma and the effects of dietary interventions, with little research on the levels of various FFA components in the FFA profile. Exploring the FFA profile is of great value for further research into the metabolic mechanisms of coronary heart disease and diabetes, predicting disease risk, and guiding prognosis and interventions. However, coronary heart disease combined with diabetes is very complex, and assessing the blood FFA profile alone cannot provide a complete picture of individual risk; it must also consider diet, lifestyle, and other factors. Therefore, exploration of the FFA profile should continue, with the hope of achieving more effective translation and application in clinical practice.
Author contributions
XL: Writing – review & editing, Writing – original draft. MG: Writing – review & editing, Supervision. NW: Writing – review & editing, Supervision, Resources, Project administration, Funding acquisition.
Funding
The author(s) declare that financial support was received for the research, authorship, and/or publication of this article. This research was supported by the National Natural Science Foundation of China (No.81700706), the Science Foundation of Liaoning science and technology Department(No. 2023JH2/101700125), the Clinical research project of Liaoning Diabetes Medical Nutrition Prevention Society (No.LNSTNBYXYYFZXH-RS01B).
Acknowledgments
We gratefully acknowledge NW for providing intellectual support and technical assistance.
Conflict of interest
The authors declare that the research was conducted in the absence of any commercial or financial relationships that could be construed as a potential conflict of interest.
Generative AI statement
The author(s) declare that no Generative AI was used in the creation of this manuscript.
Publisher’s note
All claims expressed in this article are solely those of the authors and do not necessarily represent those of their affiliated organizations, or those of the publisher, the editors and the reviewers. Any product that may be evaluated in this article, or claim that may be made by its manufacturer, is not guaranteed or endorsed by the publisher.
References
1. International Diabetes Federation. IDF Diabetes Atlas. Hagerstown, MD: International Diabetes Federation (2021). Available at: https://www.diabetesatlas.org.
2. Yun JS, Ko SH. Current trends in epidemiology of cardiovascular disease and cardiovascular risk management in type 2 diabetes. Metabolism. (2021) 123:154838. doi: 10.1016/j.metabol.2021.154838
3. Chatterjee S, Khunti K, Davies MJ. Type 2 diabetes. Lancet. (2017) 389:2239–51. doi: 10.1016/s0140-6736(17)30058-2
4. International Hypoglycaemia Study Group. Hypoglycaemia, cardiovascular disease, and mortality in diabetes: epidemiology, pathogenesis, and management. Lancet Diabetes Endocrinol. (2019) 7:385–96. doi: 10.1016/s2213-8587(18)30315-2
5. Newman JD, Schwartzbard AZ, Weintraub HS, Goldberg IJ, Berger JS. Primary prevention of cardiovascular disease in diabetes mellitus. J Am Coll Cardiol. (2017) 70:883–93. doi: 10.1016/j.jacc.2017.07.001
6. Summerhill VI, Grechko AV, Yet SF, SoBenin IA, Orekhov AN. The atherogenic role of circulating modified lipids in atherosclerosis. Int J Mol Sci. (2019) 20(14):3561. doi: 10.3390/ijms20143561
7. Osawa S, Katakami N, Kuroda A, Takahara M, Sakamoto F, Kawamori D, et al. Skin autofluorescence is associated with early-stage atherosclerosis in patients with type 1 diabetes. J Atheroscler Thromb. (2017) 24:312–26. doi: 10.5551/jat.35592
8. Di Marco E, Jha JC, Sharma A, Wilkinson-Berka JL, Jandeleit-Dahm KA, de Haan JB. Are reactive oxygen species still the basis for diabetic complications? Clin Sci (Lond). (2015) 129:199–216. doi: 10.1042/cs20150093
9. Lee HM, Kim JJ, Kim HJ, Shong M, Ku BJ, Jo EK. Upregulated NLRP3 inflammasome activation in patients with type 2 diabetes. Diabetes. (2013) 62:194–204. doi: 10.2337/db12-0420
10. Bianchi VE. Impact of nutrition on cardiovascular function. Curr Probl Cardiol. (2020) 45:100391. doi: 10.1016/j.cpcardiol.2018.08.003
11. Du H, Li D, Molive LM, Wu N. Advances in free fatty acid profiles in gestational diabetes mellitus. J Transl Med. (2024) 22:180. doi: 10.1186/s12967-024-04922-4
12. Ormseth MJ, Swift LL, Fazio S, Linton MF, Chung CP, Raggi P, et al. Free fatty acids are associated with insulin resistance but not coronary artery atherosclerosis in rheumatoid arthritis. Atherosclerosis. (2011) 219:869–74. doi: 10.1016/j.atherosclerosis.2011.09.005
13. Schauer IE, Snell-Bergeon JK, Bergman BC, Maahs DM, Kretowski A, Eckel RH, et al. Insulin resistance, defective insulin-mediated fatty acid suppression, and coronary artery calcification in subjects with and without type 1 diabetes: The CACTI study. Diabetes. (2011) 60:306–14. doi: 10.2337/db10-0328
14. Johnston LW, Harris SB, Retnakaran R, Giacca A, Liu Z, Bazinet RP, et al. Association of NEFA composition with insulin sensitivity and beta cell function in the Prospective Metabolism and Islet Cell Evaluation (PROMISE) cohort. Diabetologia. (2018) 61:821–30. doi: 10.1007/s00125-017-4534-6
15. Thulaseedharan N, Augusti KT. Risk factors for coronary heart disease in noninsulin dependent diabetes mellitus (NIDDM). Indian Heart J. (1995) 47:471–6.
16. Taniguchi A, Nakai Y, Fukushima M, Teramura S, Hayashi R, Hama K, et al. Ultrasonographically assessed carotid atherosclerosis in Japanese type 2 diabetic patients: Role of nonesterified fatty acids. Metabolism. (2002) 51:539–43. doi: 10.1053/meta.2002.31970
17. Mas S, Martínez-Pinna R, Martín-Ventura JL, Perez R, Gomez-Garre D, Ortiz A, et al. Local non-esterified fatty acids correlate with inflammation in atheroma plaques of patients with type 2 diabetes. Diabetes. (2010) 59:1292–301. doi: 10.2337/db09-0848
18. Mathew M, Tay E, Cusi K. Elevated plasma free fatty acids increase cardiovascular risk by inducing plasma biomarkers of endothelial activation, myeloperoxidase and PAI-1 in healthy subjects. Cardiovasc Diabetol. (2010) 9:9. doi: 10.1186/1475-2840-9-9
19. Zhang MH, Cao YX, Wu LG, Guo N, Hou BJ, Sun LJ, et al. Association of plasma free fatty acids levels with the presence and severity of coronary and carotid atherosclerotic plaque in patients with type 2 diabetes mellitus. BMC Endocr Disord. (2020) 20:156. doi: 10.1186/s12902-020-00636-y
20. Jin JL, Cao YX, Liu HH, Zhang HW, Guo YL, Wu NQ, et al. Impact of free fatty acids on prognosis in coronary artery disease patients under different glucose metabolism status. Cardiovasc Diabetol. (2019) 18:134. doi: 10.1186/s12933-019-0936-8
21. Sobczak ISA, Blindauer AC, Stewart JA. Changes in plasma free fatty acids associated with type-2 diabetes. Nutrients. (2019) 11(9):2022. doi: 10.3390/nu11092022
22. Palomer X, Pizarro-Delgado J, Barroso E, Vázquez-Carrera M. Palmitic and oleic acid: the yin and yang of fatty acids in type 2 diabetes mellitus. Trends Endocrinol Metab. (2018) 29:178–90. doi: 10.1016/j.tem.2017.11.009
23. Ormazabal V, Nair S, Elfeky O, Aguayo C, Salomon C, Zuñiga FA. Association between insulin resistance and the development of cardiovascular disease. Cardiovasc Diabetol. (2018) 17:122. doi: 10.1186/s12933-018-0762-4
24. Poreba M, Rostoff P, Siniarski A, Mostowik M, Golebiowska-Wiatrak R, Nessler J, et al. Relationship between polyunsaturated fatty acid composition in serum phospholipids, systemic low-grade inflammation, and glycemic control in patients with type 2 diabetes and atherosclerotic cardiovascular disease. Cardiovasc Diabetol. (2018) 17:29. doi: 10.1186/s12933-018-0672-5
25. Ahmed B, Sultana R, Greene MW. Adipose tissue and insulin resistance in obese. BioMed Pharmacother. (2021) 137:111315. doi: 10.1016/j.biopha.2021.111315
26. Ivovic A, Yung JHM, Oprescu AI, Vlavcheski F, Mori Y, Rahman SMN, et al. [amp]]beta;-cell insulin resistance plays a causal role in fat-induced β-cell dysfunction in vitro and in vivo. Endocrinology. (2024) 165(5):bqae044. doi: 10.1210/endocr/bqae044
27. Prentki M, Joly E, El-Assaad W, Roduit R. Malonyl-CoA signaling, lipid partitioning, and glucolipotoxicity: role in beta-cell adaptation and failure in the etiology of diabetes. Diabetes. (2002) 51 Suppl 3:S405–13. doi: 10.2337/diabetes.51.2007.s405
28. Weir GC. Glucolipotoxicity, β-cells, and diabetes: the emperor has no clothes. Diabetes. (2020) 69:273–8. doi: 10.2337/db19-0138
29. Kuzmenko DI, Klimentyeva TK. Role of ceramide in apoptosis and development of insulin resistance. Biochem (Mosc). (2016) 81(9):913–27. doi: 10.1134/S0006297916090017
30. Liu W, Zhu M, Liu J, Su S, Zeng X, Fu F, et al. Comparison of the effects of monounsaturated fatty acids and polyunsaturated fatty acids on the lipotoxicity of islets. Front Endocrinol (Lausanne). (2024) 15:1368853. doi: 10.3389/fendo.2024.1368853
31. Li J, Stillman JS, Clore JN, Blackard WG. Skeletal muscle lipids and glycogen mask substrate competition (Randle cycle). Metabolism. (1993) 42:451–6. doi: 10.1016/0026-0495(93)90102-t
32. Schmitz-Peiffer C, Browne CL, Oakes ND, Watkinson A, Chisholm DJ, Kraegen EW, et al. Alterations in the expression and cellular localization of protein kinase C isozymes epsilon and theta are associated with insulin resistance in skeletal muscle of the high-fat-fed rat. Diabetes. (1997) 46:169–78. doi: 10.2337/diab.46.2.169
33. Shiri H, Fallah H, Abolhassani M, Fooladi S, Ramezani Karim Z, Danesh B, et al. Relationship between types and levels of free fatty acids, peripheral insulin resistance, and oxidative stress in T2DM: A case-control study. PloS One. (2024) 19:e0306977. doi: 10.1371/journal.pone.0306977
34. Mahendran Y, Cederberg H, Vangipurapu J, Kangas AJ, Soininen P, Kuusisto J, et al. Glycerol and fatty acids in serum predict the development of hyperglycemia and type 2 diabetes in Finnish men. Diabetes Care. (2013) 36:3732–8. doi: 10.2337/dc13-0800
35. Spiller S, Blüher M, Hoffmann R. Plasma levels of free fatty acids correlate with type 2 diabetes mellitus. Diabetes Obes Metab. (2018) 20:2661–9. doi: 10.1111/dom.13449
36. Hodge AM, English DR, O'Dea K, Sinclair AJ, Makrides M, Gibson RA, et al. Plasma phospholipid and dietary fatty acids as predictors of type 2 diabetes: interpreting the role of linoleic acid. Am J Clin Nutr. (2007) 86:189–97. doi: 10.1093/ajcn/86.1.189
37. Mandal S, Causevic A, Dzudzevic-Cancar H, Semiz S. Free Fatty Acid Profile in Type 2 Diabetic Subjects with Different Control of Glycemia. In: Badnjevic A, editor. CMBEBIH 2017. IFMBE Proceedings, vol. 62 . Springer, Singapore (2017).
38. Zhuang P, Liu X, Li Y, Li H, Zhang L, Wan X, et al. Circulating fatty acids and genetic predisposition to type 2 diabetes: gene-nutrient interaction analysis. Diabetes Care. (2022) 45:564–75. doi: 10.2337/dc21-2048
39. Huang L, Lin JS, Aris IM, Yang G, Chen WQ, Li LJ. Circulating saturated fatty acids and incident type 2 diabetes: A systematic review and meta-analysis. Nutrients. (2019) 11(5):998. doi: 10.3390/nu11050998
40. Forouhi NG, Koulman A, Sharp SJ, Imamura F, Kroger J, Schulze MB, et al. Differences in the prospective association between individual plasma phospholipid saturated fatty acids and incident type 2 diabetes: the EPIC-InterAct case-cohort study. Lancet Diabetes Endocrinol. (2014) 2:810–8. doi: 10.1016/s2213-8587(14)70146-9
41. Forouhi NG, Imamura F, Sharp SJ, Koulman A, Schulze MB, Zheng J, et al. Association of Plasma Phospholipid n-3 and n-6 Polyunsaturated Fatty Acids with Type 2 Diabetes: The EPIC-InterAct Case-Cohort Study. PloS Med. (2016) 13:e1002094. doi: 10.1371/journal.pmed.1002094
42. Qian F, Ardisson Korat AV, Imamura F, Marklund M, Tintle N, Virtanen JK, et al. n-3 fatty acid biomarkers and incident type 2 diabetes: an individual participant-level pooling project of 20 prospective cohort studies. Diabetes Care. (2021) 44:1133–42. doi: 10.2337/dc20-2426
43. Mousavi SM, Jalilpiran Y, Karimi E, Aune D, Larijani B, Mozaffarian D, et al. Dietary intake of linoleic acid, its concentrations, and the risk of type 2 diabetes: A systematic review and dose-response meta-analysis of prospective cohort studies. Diabetes Care. (2021) 44:2173–81. doi: 10.2337/dc21-0438
44. Piro S, Spampinato D, Spadaro L, Oliveri CE, Purrello F, Rabuazzo AM. Direct apoptotic effects of free fatty acids on human endothelial cells. Nutr Metab Cardiovasc Dis. (2008) 18:96–104. doi: 10.1016/j.numecd.2007.01.009
45. Yu F, Zong B, Ji L, Sun P, Jia D, Wang R. Free fatty acids and free fatty acid receptors: role in regulating arterial function. Int J Mol Sci. (2024) 25(14):7853. doi: 10.3390/ijms25147853
46. Ghosh A, Gao L, Thakur A, Siu PM, Lai CWK. Role of free fatty acids in endothelial dysfunction. J BioMed Sci. (2017) 24:50. doi: 10.1186/s12929-017-0357-5
47. Sorrentino SA, Bahlmann FH, Besler C, Muller M, Schulz S, Kirchhoff N, et al. Oxidant stress impairs in vivo reendothelialization capacity of endothelial progenitor cells from patients with type 2 diabetes mellitus: restoration by the peroxisome proliferator-activated receptor-gamma agonist rosiglitazone. Circulation. (2007) 116:163–73. doi: 10.1161/circulationaha.106.684381
48. Li H, Li H, Bao Y, Zhang X, Yu Y. Free fatty acids induce endothelial dysfunction and activate protein kinase C and nuclear factor-κB pathway in rat aorta. Int J Cardiol. (2011) 152:218–24. doi: 10.1016/j.ijcard.2010.07.019
49. Henderson GC. Plasma free fatty acid concentration as a modifiable risk factor for metabolic disease. Nutrients. (2021) 13(8):2590. doi: 10.3390/nu13082590
50. Abdelhamid AS, Brown TJ, Brainard JS, Biswas P, Thorpe GC, Moore HJ, et al. Omega-3 fatty acids for the primary and secondary prevention of cardiovascular disease. Cochrane Database Syst Rev. (2018) 7:Cd003177. doi: 10.1002/14651858.CD003177.pub3
51. Bang HO, Dyerberg J, Hjøorne N. The composition of food consumed by Greenland Eskimos. Acta Med Scand. (1976) 200:69–73. doi: 10.1111/j.0954-6820.1976.tb08198.x
52. Calder PC. n-3 Fatty acids and cardiovascular disease: evidence explained and mechanisms explored. Clin Sci (Lond). (2004) 107:1–11. doi: 10.1042/cs20040119
53. Newman WP, Middaugh JP, Propst MT, Rogers DR. Atherosclerosis in alaska natives and non-natives. Lancet. (1993) 341:1056–7. doi: 10.1016/0140-6736(93)92413-n
54. Yano K, MacLean CJ, Reed DM, Shimizu Y, Sasaki H, Kodama K, et al. A comparison of the 12-year mortality and predictive factors of coronary heart disease among Japanese men in Japan and Hawaii. Am J Epidemiol. (1988) 127:476–87. doi: 10.1093/oxfordjournals.aje.a114824
55. Carlsson M, Wessman Y, Almgren P, Groop L. High levels of nonesterified fatty acids are associated with increased familial risk of cardiovascular disease. Arterioscler Thromb Vasc Biol. (2000) 20:1588–94. doi: 10.1161/01.atv.20.6.1588
56. Pirro M, Mauriège P, Tchernof A, Cantin B, Dagenais GR, Despres JP, et al. Plasma free fatty acid levels and the risk of ischemic heart disease in men: prospective results from the Québec Cardiovascular Study. Atherosclerosis. (2002) 160:377–84. doi: 10.1016/s0021-9150(01)00588-3
57. Dongliang W, Yongmei S. The significance of detecting free fatty acids and high-sensitivity C-reactive protein in type 2 diabetes combined with coronary heart disease. Int J Lab Med. (2011) 32:2. doi: 10.3969/j.issn.1673-4130.2011.21.052
58. Lv ZH, Ma P, Luo W, Xiong H, Han L, Li SW, et al. Association between serum free fatty acid levels and possible related factors in patients with type 2 diabetes mellitus and acute myocardial infarction. BMC Cardiovasc Disord. (2014) 14:159. doi: 10.1186/1471-2261-14-159
59. Yiqin W, Bo C, Changqing Z, Tianqiong W, Qinghua X. The clinical significance of coronary CT angiography combined with carotid ultrasound and serological markers in evaluating type 2 diabetes complicated by coronary heart disease. Chin J Diabetes. (2016) 24:6. doi: 10.3969/j.issn.1006-6187.2016.10.07
60. Hao L. Analysis of the risk factors associated with coronary heart disease in elderly patients with type 2 diabetes and serum free fatty acids. Int J Lab Med. (2017) 38(15):3. doi: CNKI:SUN:GWSQ.0.2017-15-058
61. Xin Y, Zhang J, Fan Y, Wang C. Serum free fatty acids are associated with severe coronary artery calcification, especially in diabetes: a retrospective study. BMC Cardiovasc Disord. (2021) 21:343. doi: 10.1186/s12872-021-02152-w
62. Tianyu L. A study on the relationship between plasma free fatty acid metabolism profile and subclinical atherosclerosis in type 2 diabetes. Doctoral dissertation, Central South University (2011).
63. Zhe L, Ruiying W, Zhicheng Z. The relationship between serum free fatty acid metabolism and component changes and macrovascular complications in patients with type 2 diabetes. J Trans Med. (2016) 5:219–23. doi: 10.3969/j.issn.2095-3097.2016.04.008
64. Takahashi M, Ando J, Shimada K, Nishizaki Y, Tani S, Ogawa T, et al. The ratio of serum n-3 to n-6 polyunsaturated fatty acids is associated with diabetes mellitus in patients with prior myocardial infarction: a multicenter cross-sectional study. BMC Cardiovasc Disord. (2017) 17:41. doi: 10.1186/s12872-017-0479-4
65. Chensi S. Study on the relationship between free fatty acid profile and atherosclerosis in patients with type 2 diabetes. Doctoral dissertation, Jilin University (2021).
66. Hu T, Zhang W, Han F, Zhao R, Liu L, An Z. Plasma fingerprint of free fatty acids and their correlations with the traditional cardiac biomarkers in patients with type 2 diabetes complicated by coronary heart disease. Front Cardiovasc Med. (2022) 9:903412. doi: 10.3389/fcvm.2022.903412
67. Wang X, Zhu L, Liu J, Ma Y, Qiu C, Liu C, et al. Palmitic acid in type 2 diabetes mellitus promotes atherosclerotic plaque vulnerability via macrophage Dll4 signaling. Nat Commun. (2024) 15:1281. doi: 10.1038/s41467-024-45582-8
68. Jaca A, Durão S, Harbron J. Omega-3 fatty acids for the primary and secondary prevention of cardiovascular disease. S Afr Med J. (2020) 110:1158–9. doi: 10.7196/SAMJ.2020.v110i12.14730
69. Bosch J, Gerstein HC, Dagenais GR, Díaz R, Dyal L, Jung H, et al. n-3 fatty acids and cardiovascular outcomes in patients with dysglycemia. N Engl J Med. (2012) 367:309–18. doi: 10.1056/NEJMoa1203859
70. Harris WS, Miller M, Tighe AP, Davidson MH, Schaefer EJ. Omega-3 fatty acids and coronary heart disease risk: clinical and mechanistic perspectives. Atherosclerosis. (2008) 197:12–24. doi: 10.1016/j.atherosclerosis.2007.11.008
71. Ghasemi Fard S, Wang F, Sinclair AJ, Elliott G, Turchini GM. How does high DHA fish oil affect health? A systematic review of evidence. Crit Rev Food Sci Nutr. (2019) 59:1684–727. doi: 10.1080/10408398.2018.1425978
72. Bhatt DL, Steg PG, Miller M, Brinton EA, Jacobson TA, Ketchum SB, et al. Cardiovascular risk reduction with icosapent ethyl for hypertriglyceridemia. N Engl J Med. (2019) 380:11–22. doi: 10.1056/NEJMoa1812792
73. Yokoyama M, Origasa H, Matsuzaki M, Matsuzawa Y, Saito Y, Ishikawa Y, et al. Effects of eicosapentaenoic acid on major coronary events in hypercholesterolaemic patients (JELIS): a randomised open-label, blinded endpoint analysis. Lancet. (2007) 369:1090–8. doi: 10.1016/s0140-6736(07)60527-3
74. Kalstad AA, Myhre PL, Laake K, Tveit SH, Schmidt EB, Smith P, et al. Effects of n-3 fatty acid supplements in elderly patients after myocardial infarction: A randomized, controlled trial. Circulation. (2021) 143:528–39. doi: 10.1161/circulationaha.120.052209
75. Nicholls SJ, Lincoff AM, Garcia M, Bash D, Ballantyne CM, Barter PJ, et al. Effect of High-Dose Omega-3 Fatty Acids vs Corn Oil on Major Adverse Cardiovascular Events in Patients at High Cardiovascular Risk: The STRENGTH Randomized Clinical Trial. Jama. (2020) 324:2268–80. doi: 10.1001/jama.2020.22258
76. Shahidi F, Ambigaipalan P. Omega-3 polyunsaturated fatty acids and their health benefits. Annu Rev Food Sci Technol. (2018) 9:345–81. doi: 10.1146/annurev-food-111317-095850
77. Abdelhamid AS, Brown TJ, Brainard JS, Biswas P, Thorpe GC, Moore HJ, et al. Omega-3 fatty acids for the primary and secondary prevention of cardiovascular disease. Cochrane Database systematic Rev. (2020) 3:Cd003177. doi: 10.1002/14651858.CD003177.pub5
78. Tenenbaum A, Fisman EZ. Omega-3 polyunsaturated fatty acids supplementation in patients with diabetes and cardiovascular disease risk: does dose really matter? Cardiovasc Diabetol. (2018) 17:119. doi: 10.1186/s12933-018-0766-0
79. Sleiman D, Al-Badri MR, Azar ST. Effect of mediterranean diet in diabetes control and cardiovascular risk modification: a systematic review. Front Public Health. (2015) 3:69. doi: 10.3389/fpubh.2015.00069
80. Becerra-Tomás N, Blanco Mejía S, Viguiliouk E, Khan T, Kendall CWC, Kahleova H, et al. Mediterranean diet, cardiovascular disease and mortality in diabetes: A systematic review and meta-analysis of prospective cohort studies and randomized clinical trials. Crit Rev Food Sci Nutr. (2020) 60:1207–27. doi: 10.1080/10408398.2019.1565281
81. Bowman L, Mafham M, Stevens W, Haynes R, Aung T, Chen F, et al. ASCEND: A Study of Cardiovascular Events iN Diabetes: Characteristics of a randomized trial of aspirin and of omega-3 fatty acid supplementation in 15,480 people with diabetes. Am Heart J. (2018) 198:135–44. doi: 10.1016/j.ahj.2017.12.006
82. Sayah N, Bhatt DL, Miller M, Brinton EA, Jacobson TA, Ketchum SB, et al. Icosapent ethyl following acute coronary syndrome: the REDUCE-IT trial. Eur Heart J. (2024) 45:1173–6. doi: 10.1093/eurheartj/ehad889
83. SACN (Scientific Advisory Committee on Nutrition). Saturated fats and health (2019). Available online at: https://www.gov.uk/government/publications/saturated-fats-and-health-sacn-report (Accessed 2022).
84. U.S. Department of Health and Human Services and U.S. Department of Agriculture. Dietary guidelines for americans (2015-2020). Available online at: http://health.gov/dietaryguidelines/2015/guidelines/ (Accessed 15 April 2020).
85. The French Food Safety Agency (AFSSA). Available online at: https://www.anses.fr/en/content/fats (accessed March 02, 2022).
86. Nutrition and Metabolic Management Branch of China International Exchange and Promotive Association for Medical and Health Care, Clinical Nutrition Branch of Chinese Nutrition Society, Chinese Diabetes Society, Chinese Society for Parenteral and Enteral Nutrition, Chinese Clinical Nutritionist Center of Chinese Medical Doctor Association. Guidelines for medical nutrition therapy for diabetes in China (2022 edition). Chin J Diabetes. (2022) 14:881–933. doi: 10.3760/cma.j.cn115791-20220704-00324
87. Maki KC, Dicklin MR, Kirkpatrick CF. Saturated fats and cardiovascular health: Current evidence and controversies. J Clin Lipidol. (2021) 15:765–72. doi: 10.1016/j.jacl.2021.09.049
88. Kris-Etherton PM, Krauss RM. Public health guidelines should recommend reducing saturated fat consumption as much as possible: YES. Am J Clin Nutr. (2020) 112:13–8. doi: 10.1093/ajcn/nqaa110
89. Kwon B, Querfurth HW. Palmitate activates mTOR/p70S6K through AMPK inhibition and hypophosphorylation of raptor in skeletal muscle cells: Reversal by oleate is similar to metformin. Biochimie. (2015) 118:141–50. doi: 10.1016/j.biochi.2015.09.006
90. Chinese Diabetes Society. Guidelines for the prevention and treatment of type 2 diabetes in China (2020 edition). Chin J Pract Internal Med. (2021) 41:668–95. doi: 10.19538/jnk2021080106
91. Klein S, Burke LE, Bray GA, Blair S, Allison DB, Pi-Sunyer X, et al. Clinical implications of obesity with specific focus on cardiovascular disease: a statement for professionals from the American Heart Association Council on Nutrition, Physical Activity, and Metabolism: endorsed by the American College of Cardiology Foundation. Circulation. (2004) 110:2952–67. doi: 10.1161/01.Cir.0000145546.97738.1e
92. Nakamura K, Fuster JJ, Walsh K. Adipokines: a link between obesity and cardiovascular disease. J Cardiol. (2014) 63:250–9. doi: 10.1016/j.jjcc.2013.11.006
93. Koliaki C, Liatis S, Kokkinos A. Obesity and cardiovascular disease: revisiting an old relationship. Metab Mar. (2019) 92:98–107. doi: 10.1016/j.metabol.2018.10.011
94. Boden G. Obesity, insulin resistance and free fatty acids. Curr Opin Endocrinol Diabetes Obes. (2011) 18:139–43. doi: 10.1097/MED.0b013e3283444b09
95. Sahebkar A, Simental-Mendía LE, Pedone C, Ferretti G, Nachtigal P, Bo S, et al. Statin therapy and plasma free fatty acids: a systematic review and meta-analysis of controlled clinical trials. Br J Clin Pharmacol. (2016) 81:807–18. doi: 10.1111/bcp.12854
96. Bahiru E, Hsiao R, Phillipson D, Watson KE. Mechanisms and treatment of dyslipidemia in diabetes. Curr Cardiol Rep. (2021) 23:26. doi: 10.1007/s11886-021-01455-w
97. Vavlukis M, Kedev S. Effects of high intensity statin therapy in the treatment of diabetic dyslipidemia in patients with coronary artery disease. Curr Pharm Des. (2018) 24:427–41. doi: 10.2174/1381612824666171227215708
98. Wu L, Parhofer KG. Diabetic dyslipidemia. Metabolism. (2014) 63:1469–79. doi: 10.1016/j.metabol.2014.08.010
99. Galicia-Garcia U, Jebari S, Larrea-Sebal A, Uribe KB, Siddiqi H, Ostolaza H, et al. Statin treatment-induced development of type 2 diabetes: from clinical evidence to mechanistic insights. Int J Mol Sci. (2020) 21(13):4725. doi: 10.3390/ijms21134725
100. Yanling Z, Jianjun X, Zhongchao C. Research progress on the correlation between serum free fatty acids and cardiovascular diseases. Chin J Cardiovasc Dis. (2021) 26:585–8. doi: 10.3969/j.issn.1007-5410.2021.06.019
101. Shitole SG, Biggs ML, Ix JH, Fretts AM, Tracy RP, Siscovick DS, et al. Fasting and postload nonesterified fatty acids and glucose dysregulation in older adults. Am J Epidemiol. (2022) 191:1235–47. doi: 10.1093/aje/kwac044
102. Li F, Ye J, Sun Y, Lin Y, Wu T, Shao C, et al. Distinct dose-dependent association of free fatty acids with diabetes development in nonalcoholic fatty liver disease patients. Diabetes Metab J. (2021) 45:417–29. doi: 10.4093/dmj.2020.0039
103. Alfhili MA, Alsughayyir J, BaSudan A, Alfaifi M, Awan ZA, Algethami MR, et al. Blood indices of omega-3 and omega-6 polyunsaturated fatty acids are altered in hyperglycemia. Saudi J Biol Sci. (2023) 30:103577. doi: 10.1016/j.sjbs.2023.103577
Keywords: free fatty acid profile, polyunsaturated fatty acids, coronary heart disease, type 2 diabetes, atherosclerosis
Citation: Liu X, Gong M and Wu N (2024) Research progress on the relationship between free fatty acid profile and type 2 diabetes complicated by coronary heart disease. Front. Endocrinol. 15:1503704. doi: 10.3389/fendo.2024.1503704
Received: 29 September 2024; Accepted: 14 November 2024;
Published: 06 December 2024.
Edited by:
Aleksandra Klisic, Primary Health Care Center Podgorica, MontenegroReviewed by:
Dragos-Florin Baba, George Emil Palade University of Medicine, Pharmacy, Sciences and Technology of Târgu Mureş, RomaniaYin Kang, Guangdong Provincial People’s Hospital, China
Copyright © 2024 Liu, Gong and Wu. This is an open-access article distributed under the terms of the Creative Commons Attribution License (CC BY). The use, distribution or reproduction in other forums is permitted, provided the original author(s) and the copyright owner(s) are credited and that the original publication in this journal is cited, in accordance with accepted academic practice. No use, distribution or reproduction is permitted which does not comply with these terms.
*Correspondence: Na Wu, MzQ0MTUzNTIyM0BxcS5jb20=