- 1Department of Obstetrics and Gynecology, Hefei Maternal and Child Health Hospital, Hefei, China
- 2Department of Obstetrics and Gynecology, Anhui Women and Children’s Medical Center, Hefei, China
- 3Department of Obstetrics and Gynecology, Maternal and Child Medical Center of Anhui Medical University, Hefei, China
- 4Fifth School of Clinical Medicine, Anhui Medical University, Hefei, China
Objective: Gestational diabetes mellitus (GDM) is a common complication during pregnancy and increases the risk of metabolic diseases in offspring. We hypothesize that the poor intrauterine environment in pregnant women with GDM may lead to chromosomal DNA damage and telomere damage in umbilical cord blood cells, providing evidence of an association between intrauterine programming and increased long-term metabolic disease risk in offspring.
Methods: We measured telomere length (TL), serum telomerase (TE) activity, and oxidative stress markers in umbilical cord blood mononuclear cells (CBMCs) from pregnant women with GDM (N=200) and healthy controls (Ctrls) (N=200) and analysed the associations of TL with demographic characteristics, biochemical indicators, and blood glucose levels.
Results: The length of telomeres in umbilical CBMCs in the GDM group was significantly shorter than that in the Ctrl group (P<0.001), and the shortening of telomeres in male infants in the GDM group was more significant than that in the Ctrl group (P<0.001) after adjustment for Pre-pregnancy body mass index (PBMI), Pregnancy weight gain (PGW), and Triglyceride (TG) as confounding factors. In addition, the TE expression level in the GDM group was lower after adjustment. There was no statistically significant difference in oxidative stress hydroxydeoxyguanosine (8-OHdG), malondialdehyde (MDA) and superoxide dismutase (SOD) between the two groups. TL was positively correlated with TE activity, and both were negatively correlated with blood glucose levels. There was no correlation between TL and Gestational age (GA), PBMI, PGW, or TG levels.
Conclusion: The poor intrauterine environment in pregnant women with GDM increases telomere attrition and reduces TE activity, which may be potential genetic risk factors for an increased risk of metabolic diseases in offspring later in life due to intrauterine reprogramming.
Background
In recent years, the global trend towards GDM has significantly increased, especially since the end of the last century (1). According to the International Diabetes Federation (IDF), the global incidence of GDM was approximately 14% in 2021, with standardised prevalences of 12.7%, 9.2%, and 14.2% in low-, middle-, and high-income countries, respectively, with different economic levels (2). Notably, the incidence of GDM in China is approximately 11.91%, which is significantly higher than that in neighbouring Asian countries such as Korea, Japan, and Thailand, where the prevalence of GDM remains below 8% (3).
With advancements in modern medical knowledge, people are becoming increasingly aware of the importance of the unfavourable intrauterine environment in pregnant women with GDM for foetal growth and development. GDM has been shown to be strongly associated with long-term metabolic imbalances in offspring (4–19), including impaired glucose tolerance, obesity, and cardiovascular disease, and these adverse outcomes are proportional to the duration of intrauterine exposure for the foetus (20–24). A variety of factors contribute to the poor intrauterine environment, prompting the foetus to adapt to environmental changes through a “foetal programming” mechanism, a process that may increase the risk of future diseases in the short or long term. Evidence suggests that children of women with diabetes, particularly those with type 1 diabetes, may be at increased risk of glucose intolerance and cardiovascular disease, even in infancy and adolescence (20). Notably, GDM can contribute to an undesirable intrauterine environment; even if the mother successfully controls her blood glucose levels during pregnancy, she may still experience adverse pregnancy outcomes and long-term health problems in her offspring (25). These phenomena may be related to changes in genetic pathways and endocrine regulation, leading to adaptive reprogramming in foetuses exposed to adverse intrauterine environments, which may be a critical pathway for the development of metabolic diseases in adulthood (26, 27). Although these studies have shed light on some of the causes of disease associations, the specific mechanisms underlying the increased risk of chronic diseases caused by intrauterine exposure to adverse environments are still largely being explored and have not been fully elucidated (28). Changes in the telomere/telomerase system in this complex adaptive programming process have long been relatively underappreciated. Telomeres, which are composed of noncoding DNA repeats at the ends of chromosomes (the basic unit of which is TTAGGG), have variable lengths and are responsible for protecting chromosomes and maintaining genome integrity (29, 30). Typically, the length of telomeres in somatic cells decreases with age (31). During cell division and chromosome replication, approximately 150 to 200 base pairs are reduced at a time, and if this process continues, DNA damage accumulates; once telomeres shrink to a critical length, cells begin the ageing process (32). Thus, telomeres are often seen as markers of cumulative cellular damage (29, 30). To date, an abnormal TL has been found to be associated with a wide range of diseases, including impaired glucose tolerance, dyslipidaemia, arteriosclerosis, obesity, diabetes, cardiovascular disease, and many cancers (33).
TL adjustment is a complex process influenced by multiple factors that involves internal and external elements. Among these factors, TE activity plays a key role in the regulation of TL (34). Studies have shown that the rate of telomere shortening in populations of African descent exceeds that in populations of European descent (34). Additionally, single-nucleotide polymorphisms in the TE reverse transcriptase (hTERT) promoter region unique to the Japanese population lead to effective resistance to the increasing phenomenon of telomere loss with age (35), an effect not reported in other ethnic groups (36). TE is responsible for telomere elongation and is a basic ribonucleic acid-dependent DNA polymerase. TE uses its own telomerase RNA component (TERC) to cooperate with telomerase-associated proteins (human) via its RNA template under the catalysis of the TE catalytic subunit human telomerase reverse transcriptase (hTERT). Together with telomerase-associated protein 1 (TEP1), a complex reverse transcriptase complex that uses an RNA template to reverse transcribe DNA repeats catalysed by TERT to produce DNA repeats is formed, which are added to telomere ends to compensate for shortening due to the deletion of replication ends (37, 38). Previous sporadic studies on the effects of gestational diabetes on the telomere system in neonates have been inconclusive. Considering the adverse intrauterine environment caused by GDM, we speculate that GDM may have a certain effect on TL and TE activity in newborns, and we conducted our research on the basis of this hypothesis.
Materials and methods
Study participants
This study adopted a prospective cohort study design and focused on a group of pregnant women whose files were established and who received regular prenatal check-ups at Hefei Maternal and Child Health Hospital from November 2022 to October 2023. We systematically collected the basic information of these pregnant women (including their demographic and clinical characteristics) and built an electronic health record database. Over the course of the study, we continued to follow up on the pregnancy progress, pregnancy outcomes, and newborn birth status of the participating pregnant women. This research protocol was successfully approved by the Ethics Review Committee of Hefei Maternal and Child Health Hospital. Before each pregnant woman was enrolled in the study cohort, we detailed the purpose and importance of the study and ensured that they signed a written informed consent form on the basis of their full understanding.
The inclusion criteria were as follows: ≥18 years of age, singleton pregnancy, establishment of a file at 8-12 weeks of gestation, prenatal examination and delivery at Hefei Maternal and Child Health Hospital, and oral glucose tolerance test (OGTT) with 75 grams of glucose conducted between 24 and 28 weeks of gestation. The exclusion criteria were as follows: pregnant women with multiple pregnancies; pregnant women who underwent therapeutic labour induction because the foetus was malformed or stillborn; pregnant women with a history of drug use, malignancy, essential hypertension, type 1 or type 2 diabetes, liver insufficiency, renal insufficiency, autoimmune diseases, long-term use of drugs that affect blood sugar, etc.; and pregnant women with incomplete information provided in their individual medical records.
The diagnostic criteria for GDM were based on the criteria of the International Diabetes and Pregnancy Study Group For the OGTT conducted between 24 and 28 weeks of gestation, 75 grams of glucose were taken orally, and fasting blood glucose (FPG), 1-hour blood glucose, and 2-hour blood glucose levels were measured. If any of the following criteria were met, GDM was diagnosed: a FPG level ≥ 5.1 mmol/L, a 1-hour plasma glucose level ≥ 10.0 mmol/L, or a 2-hour plasma glucose level ≥8.5 mmol/L.
Specimen collection
After delivery of the foetus and placenta, a sample of umbilical cord blood was collected by a specialist obstetrician via an EDTA anticoagulant tube according to standard protocols in the field of obstetrics. The umbilical cord blood sample was subsequently left in its native state for two hours and centrifuged at 3000 rpm for ten minutes to achieve effective separation of plasma from monocytes. The isolated plasma and blood cells were carefully aliquoted into 2 mL EP tubes and stored at a cryogenic temperature of -80 degrees Celsius prior to DNA extraction. Total DNA was isolated from monocytes via the use of ATL, proteinase K, and RNase A buffers (Qiagen, Inc., Valencia, CA) for phenol–chloroform extraction and ethanol precipitation.
Determination of TL
TL is determined via a method based on fluorescence quantitative PCR proposed by Cawthon, which is low-cost and relatively simple method that is commonly used for determining TL. In this method, the relative TL of different samples was obtained by measuring the T/S ratio of the telomere copy number (T) and single-copy gene (S) of the sample and reference DNA, which is proportional to the average TL. In this study, the reference gene was selected as a single copy of 36B4, the sequence of which is reported in the reference article (39, 40), and the primers used for telomere detection are reported in the reference report (40). Amplification was performed on an ABI 7900 real-time PCR instrument (Applied Biosystems, Foster City, California) via an optimised program, and the melting curve program was used. The telomeric gene primers were as follows: tel F1: CGGTTTGTTTGGGTGGGTTTGGGTTTGGGTTTGGGTTTGGGTT; tel R1: GGCTTGCCTTACCCTTACCCTTACCCTCTTACCCTTCT. The primers for the internal control genes were as follows: 36B4-F: CAGCAAGTGGGAAGGTGTAATCC; and 36B4-R: CCCATTCTATCATCAACGGGTACAAA. The quantitative PCR procedure was as follows: 95°C for 1 min; 40 cycles at 95°C for 5 s and 55°C for 45 s. The data quality control measures were as follows: The final Ct value of each detected gene was taken as the average of the Ct values of the two replicate wells. For the internal control gene, if the coefficient of variation of the Ct value of the two replicates was greater than 2.5%, the data of this locus were excluded. For telomere genes, if the coefficient of variation of the Ct value of two double wells was greater than 5%, the data of this locus were excluded. If there was a large difference in the Ct values between the two replicates, the data for that locus were invalidated. For the final Ct value of each detected gene, the difference between the CT value of the negative parameter and the CT value of the sample should be greater than 4 (that is, the Ct negative parameter - Ct sample≥4); otherwise, the data of this locus were considered invalid. The relative telomere length was calculated as follows: TL (T/S) = 2-ΔΔCt, where ΔCt = Mean Ct (telomere) - Mean Ct (internal reference gene) and ΔΔCt =ΔCt (sample to be tested) -ΔCt (corrected sample).
Determination of TE activity and oxidative stress indicators
An ELISA kit (ELISA, China) was used to measure the TE activity and oxidative stress (SOD, 8-OHdG, and MDA) levels. The absorbance (OD value) was determined with a microplate reader at a wavelength of 450 nm, and the amount of protein of interest in the sample was calculated from a standard curve. The data analysis steps were as follows: 1) By default, each detected protein was read in 2 parallels; each parallel well was read 3 times and corrected with blank wells; the average of the 3 readings was calculated as the absorbance value of each parallel well; and the average value and coefficient of variation of the 2 parallels were calculated. If the coefficient of variation exceeded >15%, the data were highly variable and may be biased. 2) A standard curve was drawn according to the concentration and absorbance value of the standard, and the protein concentration of the sample to be tested in the corresponding plate was calculated according to the standard curve of each plate. 3) When the absorbance value of the sample to be measured exceeded the highest point of the standard curve, the sample was diluted to the linear range and remeasured, and the corresponding dilution factor was multiplied when the concentration was calculated.
Statistical methods
SPSS 26.0 statistical software (IBM, New York, USA) was used for analysis. For a distribution that conforms to normality, it is expressed as a mean ± standard deviation. When the data does not conform to a normal distribution, median and quartile ranges are used to describe trends in the dataset and dispersion. Independent-samples T-test for variables that conform to normal distribution and satisfy the assumption of variance homogeneity. When the non-normal distribution or the homogeneity assumption of variance is not satisfied, the Mann-Whitney U test is used for comparison between groups, and the analysis of covariance (ANCOVA) is used to control for confounders. The results of the analysis were visualized using R software (R-4.3.2, UoA, New Zealand). Spearman was used to analyse the correlation between TL and clinical indicators. The difference is considered statistically significant when the calculated P < 0.05.
Results
The flowchart is shown in Figure 1. This study included a group of pregnant women whose files were established at Hefei Maternal and Child Health Hospital and who received regular prenatal check-ups from November 2022 to October 2023. Initially, 2956 pregnant women were surveyed, and these women were included in the study. Among these women, 2895 completed an oral glucose tolerance test (OGTT) and had documented results. A total of 216 patients were diagnosed with GDM, with an incidence of 7.24% in this population. Sixteen patients were subsequently excluded because of factors such as incomplete data, loss of tracking, and unsuccessful DNA extraction. Finally, a total of 200 GDM patients were enrolled in the study cohort, and 200 Ctrls who visited during the same period were selected.
Comparison of demographic and clinical data between pregnant women with GDM and Ctrls without GDM
Table 1 shows that the PBMI, PWG and TG levels of the GDM group were significantly greater than those of the Ctrl group (P<0.05). The results of the OGTT revealed that the 1-hour plasma glucose and 2-hour plasma glucose levels in the GDM group were significantly greater than those in the Ctrl group. The gestational age at delivery was earlier in the GDM group than in the Ctrl group.
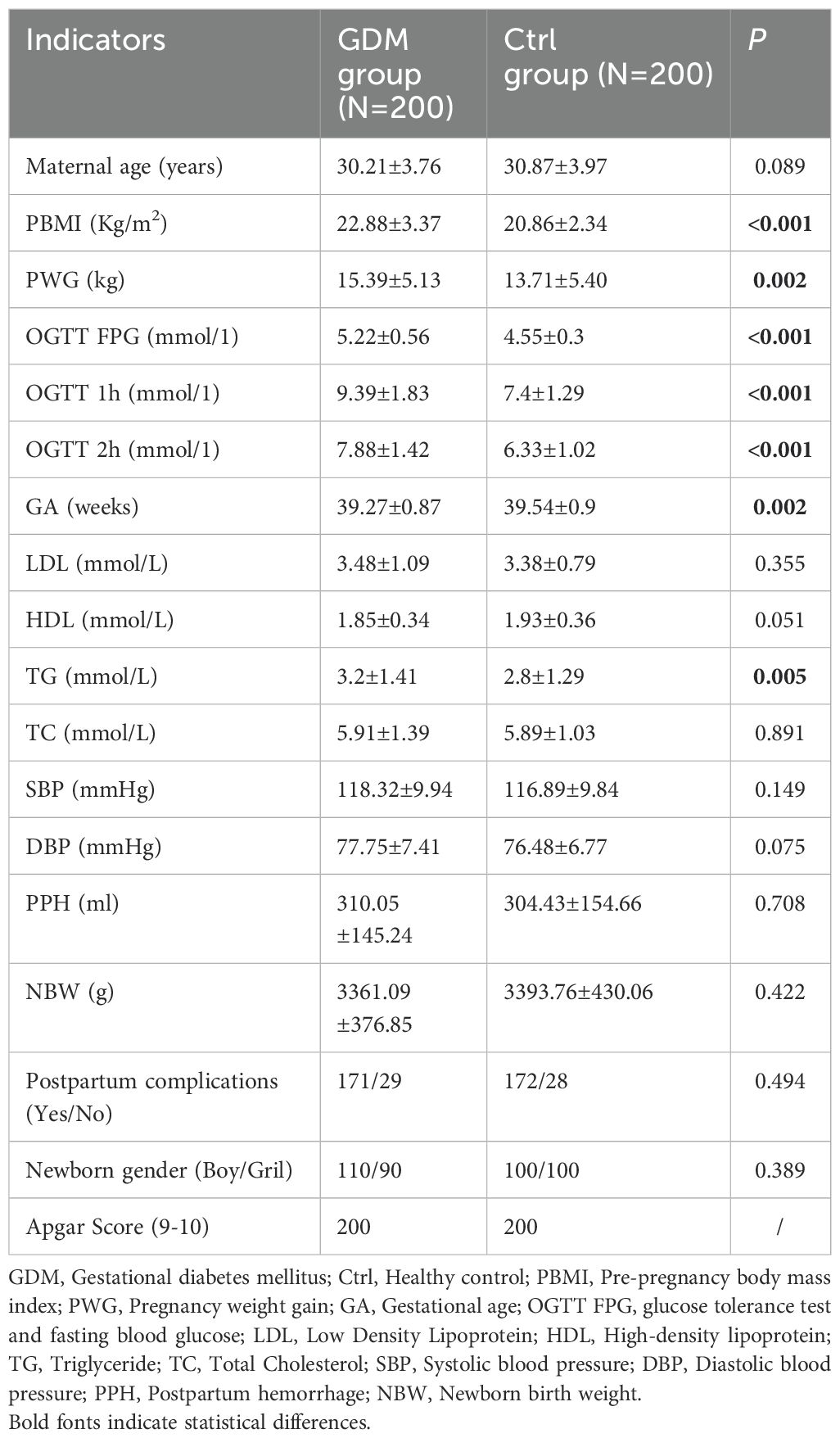
Table 1. Comparison of demographic information and clinical indicators between pregnant women with GDM and Ctrl group.
Comparison of the relative TL of umbilical CBMCs in the GDM and Ctrl groups
As shown in Figure 2, the relative TL of CBMCs in the GDM group was significantly shorter than that in the Ctrl group, and the difference between the two groups was statistically significant (0.69 ± 0.22 vs. 0.77 ± 0.19, P < 0.001). However, after correction for PBMI, PWG, and TG levels, the difference in TL between the two groups remained statistically significant (P < 0.001). According to the stratification analysis of infants, as shown in Figures 3, 4, there was a statistically significant difference in the relative TL of umbilical cord blood cells between the GDM group and the Ctrl group (0.68 ± 0.22 vs. 0.78 ± 0.17, P < 0.001), and the difference remained statistically significant after adjustment for PBMI, PWG, and TG levels (P < 0.001). There was a statistically significant difference in the TL of umbilical cord blood cells before correction, but no difference was observed after adjustment (P = 0.322).
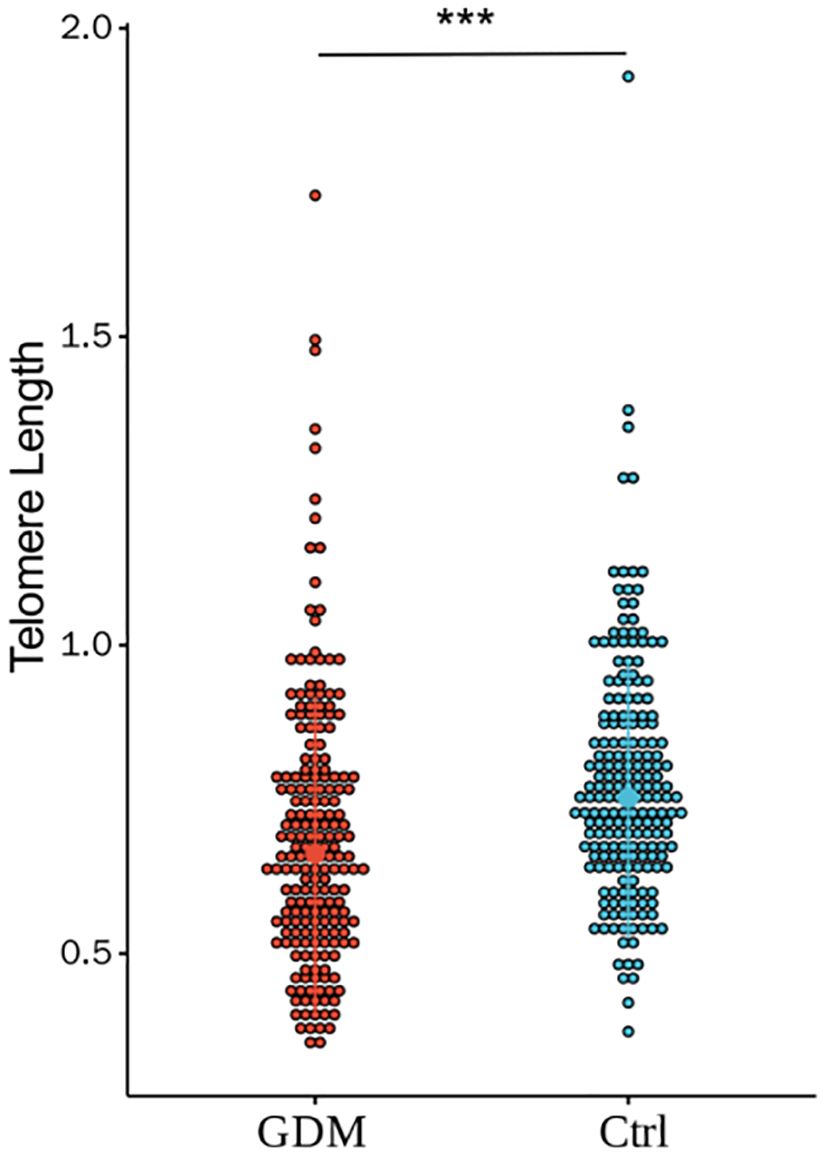
Figure 2. Comparison of the relative TL of umbilical CBMC in GDM group and Ctrl group.(*** for P<0.001) There was a significant statistical difference in relative telomere length between the two groups (0.69±0.22 vs. 0.77±0.19, P < 0.001). By adjusting for PBMI, PWG, and TG, there were still differences between the two groups (P<0.001).(*** for P<0.001, Ctrl for healthy control).
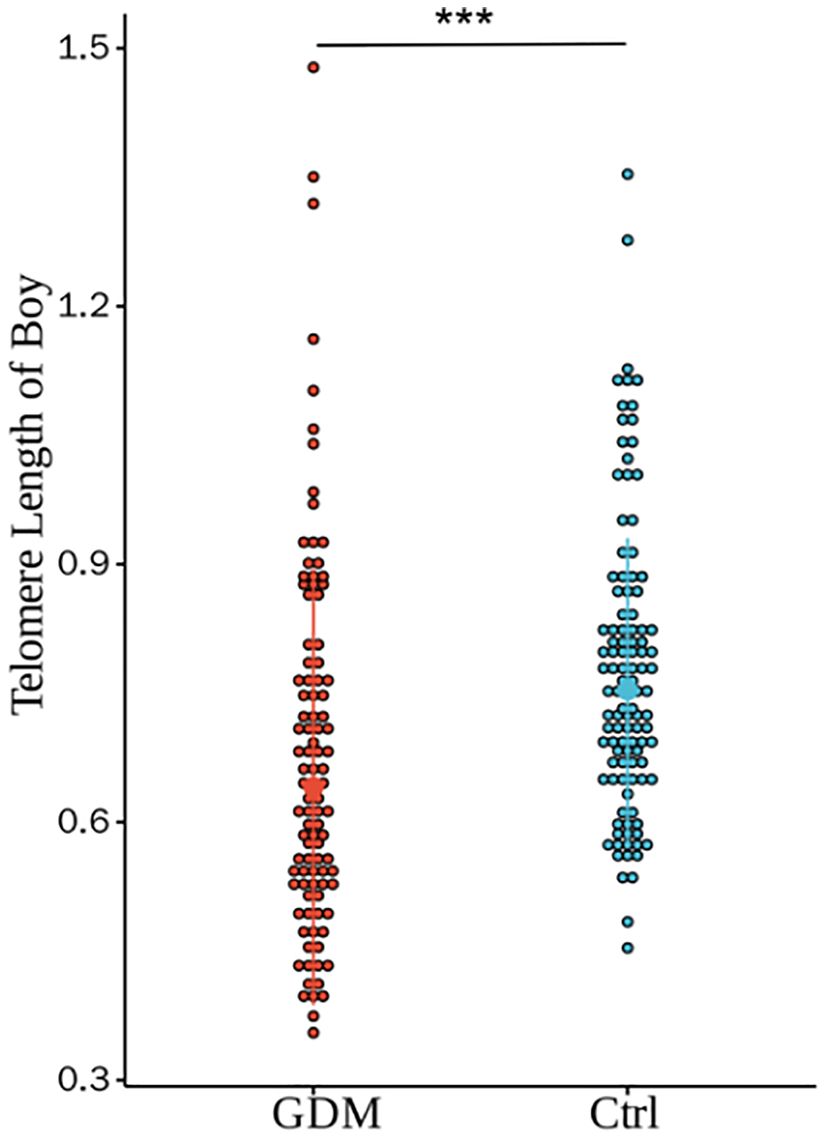
Figure 3. Comparison of umbilical cord blood relative TL between GDM group and Ctrl group offspring male infants. There were statistically significant differences in the relative TL between the two groups (0.68±0.22 vs. 0.78±0.17, P < 0.001), and the differences were still statistically significant after adjusting for PBMI, PWG, and TG (P<0.001).(*** for P<0.001,Ctrl for healthy control).
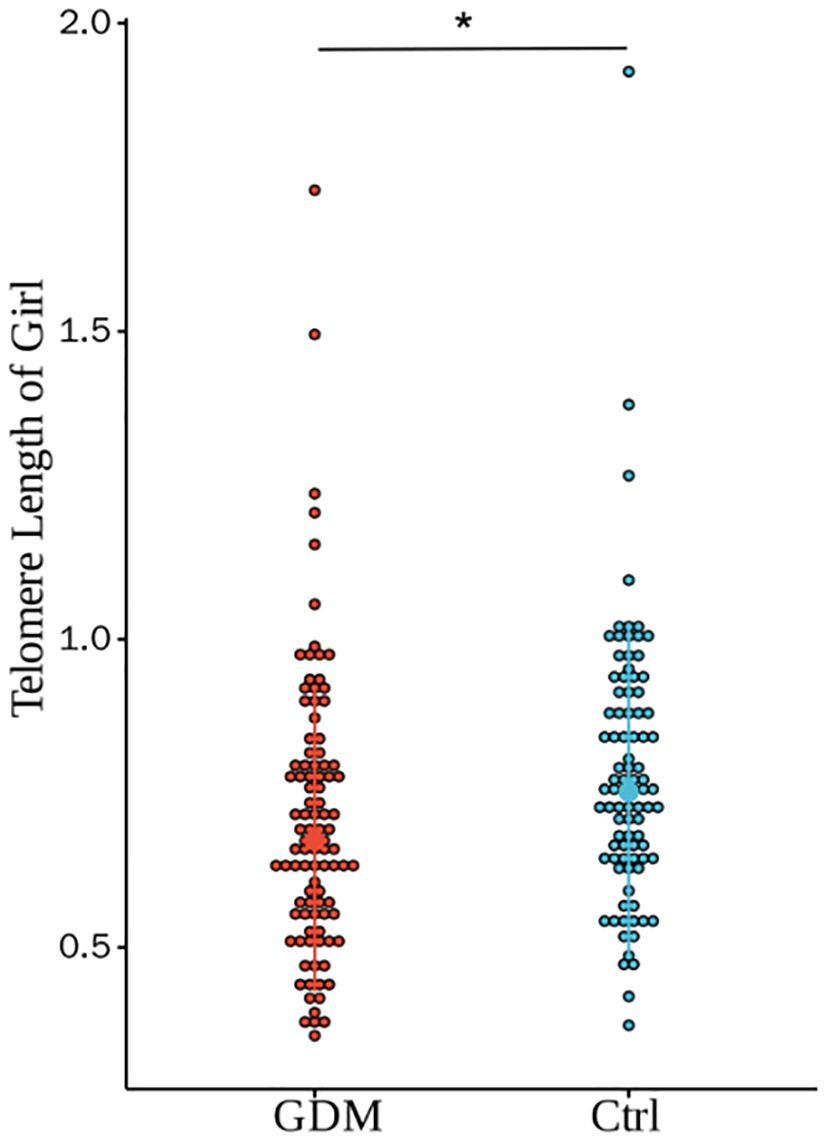
Figure 4. Comparison of umbilical cord blood relative TL of GDM group and Ctrl group offspring of female infants. There was a statistically significant difference in the relative TL between the two groups (0.71±0.23 vs. 0.76±0.22, P=0.028), and the difference was still statistically significant after adjusting for PBMI, PWG, and TG (P=0.322).(* for P<0.05, Ctrl for healthy control).
Correlation analysis between relative TL and clinical indicators
As shown in Figure 5, the relative TL of umbilical CBMCs was significantly negatively correlated with FPG levels determined via an OGTT (r = -0.140, P = 0.021), but it was not correlated with the following indicators: PBMI (r = -0.062, P = 0.311), PWG (r = 0.044, P = 0.469), GA (r = 0.112, P = 0.067), or TG levels (r = -0.049, P = 0.415).
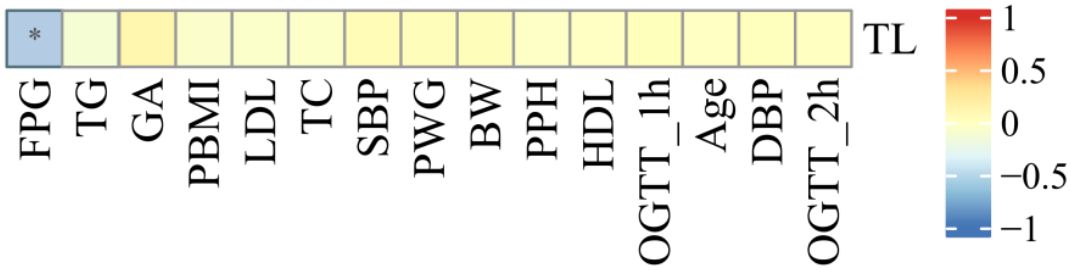
Figure 5. Correlation analysis between cord blood relative TL and clinical indicators. As shown in figure , the relative TL in umbilical cord blood was negatively correlated with FPG (r = -0.140, P = 0.021), and there was no correlation with the statistical differences between PBMI, Maternal age, PWG and TG (all p>0.05). (* for P<0.05).
Comparison of TE expression levels and oxidative stress indices in umbilical cord blood between the GDM group and the Ctrl group
As shown in Figures 6, 7, the expression level of TE in the umbilical cord blood of the GDM group was significantly lower than that in the cord blood of the Ctrl group (P < 0.001), and the difference was still statistically significant after adjustment for different factors (P < 0.001). Although the levels of 8-OHdG, MDA and SOD were significantly increased in the umbilical cord blood of the GDM group, there was no significant difference after adjustment for different factors (all P > 0.05).
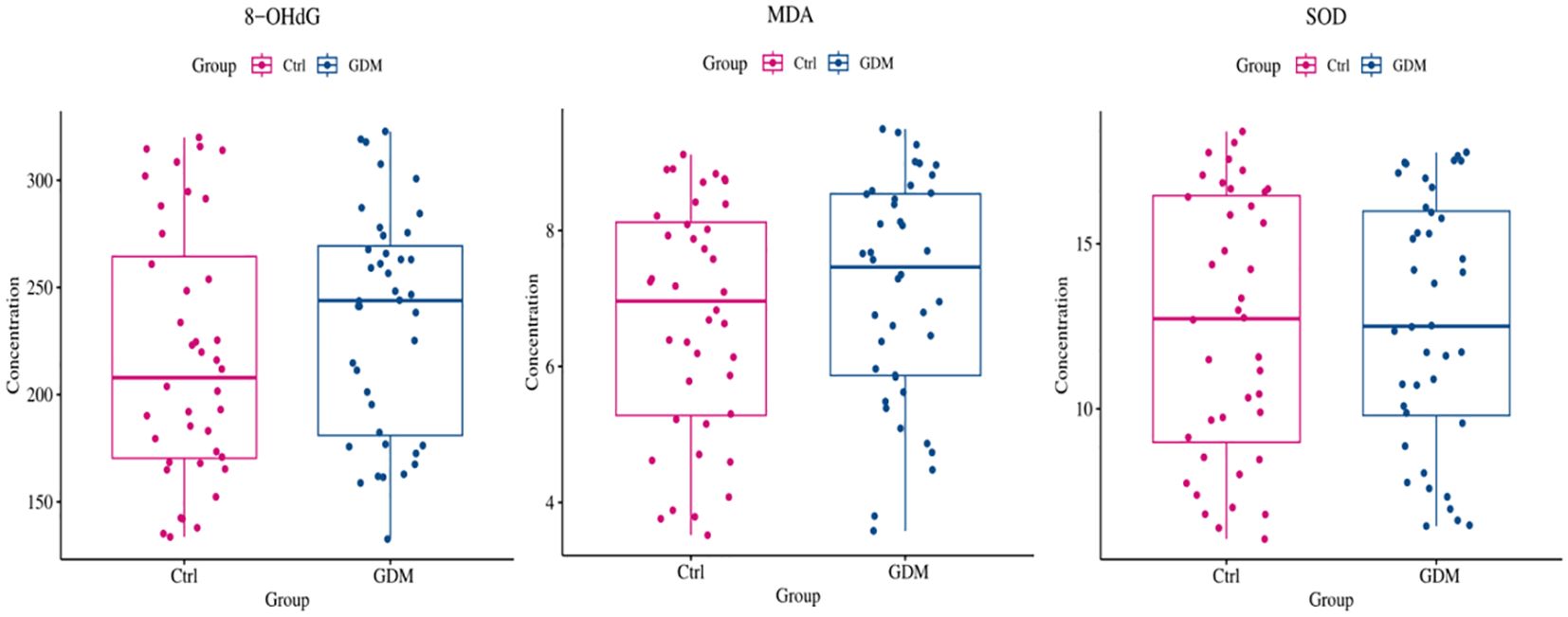
Figure 6. Comparison of oxidative stress indexes in umbilical cord blood between GDM patients and healthy control patients. As shown in figure, although the expression levels of 8-OHdG, MDA, AND SOD in the umbilical cord blood of GDM patients increased, no statistical difference was found (all P>0.05).
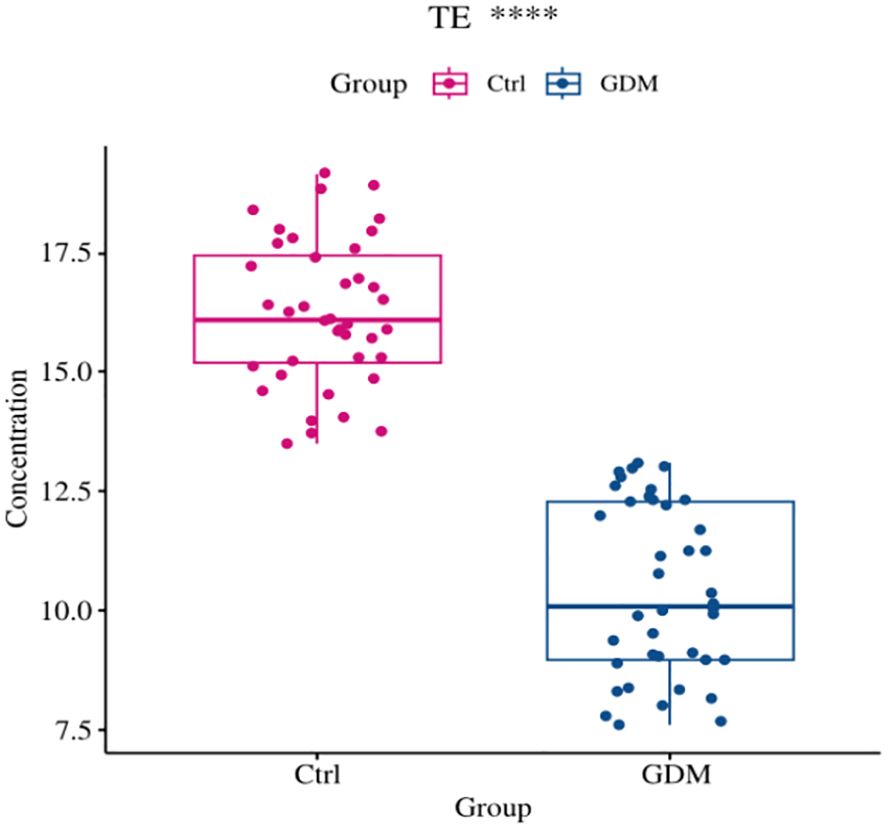
Figure 7. Comparison of cord blood telomerase expression levels between GDM group and Ctrl group. The expression level of TE in cord blood of GDM offspring was significantly lower than that of Ctrl group (10.43±1.80 vs. 16.24±1.53, P<0.001), and the difference was still statistically significant after adjusting for PBMI, PWG, TG (P<0.001).(*** for P<0.001,TE for telomerase, Ctrl for healthy control).
Correlation analysis between TE and TL and various clinical indicators
The results of the analysis are shown in Figure 8, and there was a significant positive correlation between TL and the serum TE expression level (r = 0.699, P < 0.001). However, there was a significant negative correlation between TE and OGTT-FPG (r = -0.487, P < 0.001), OGTT-1h (r = -0.538, P < 0.001), and OGTT-2h levels (r = -0.567, P < 0.001).No correlation was found between TL and oxidative stress indicators (8-OHdG, MDA, SOD) (all P>0.05).
Discussion
In this study, the incidence of GDM in this region was approximately 7.24%, and the relative TL of CBMCs in the offspring of GDM patients was significantly shorter than that in the offspring of healthy pregnant women, and the expression level of TE was reduced. Correlation analysis revealed that there was a positive correlation between TL and TE activity and that there was a negative correlation between the two parameters and blood glucose levels. This finding differs from those of previous studies (41), in which no difference in TL but only a certain change in TE activity were reported. On the one hand, the reason for the difference in results may be that they had a sample size of only a few dozen cases; on the other hand, the reason could be the difference in detection methods, as they used flow cytometry.
Previous studies have suggested that increased foetal oxidative stress in the intrauterine environment is associated with maternal GDM and accelerated telomere attrition (42–44). However, unlike in these studies, in this study, an increase in oxidative stress was not observed in the GDM group, but there was no significant difference compared with that in the Ctrl group, which differs from the findings of previous studies. Therefore, this study suggests that abnormal oxidative stress indicators may be a potential driver of telomere shortening but not a fundamental factor. In addition to cell division, TL may also be regulated by a variety of factors, such as genetic factors and infection, in addition to cell division (45, 46). Telomeric DNA has been shown to be highly sensitive to reactive ROS, especially double-stranded telomeric DNA, which is relatively susceptible to ROS cleavage and has a cleavage preference at the 5’ end of the 5’-GGG-’ sequence (47). Through this study, we speculate that the lack of differences in umbilical cord blood oxidation indices in the offspring of GDM patients may be due to the short duration of GDM and the inherent oxidation and antioxidant balance mechanism in the human body, even in the foetal stage. Thus, the mechanism of telomere shortening may not be solely attributable to the increase in oxidative stress.
Telomeres, as protective structures at the ends of chromosomes, play a key role in maintaining the integrity of DNA information, and their length decreases with age (48–50). In addition, effectively controlling for the confounding factor of TL change may be caused by maternal age (P>0.05). In addition, we also found that the levels of PBMI, PGW, and TG in the GDM group were greater than those in the Ctrl group, and the elevated levels of these indicators were consistent with the typical metabolic abnormalities in GDM patients. Previous studies have shown that PBMI (28, 51–55), PGW (56, 57), and TG (58) levels are factors contributing to telomere shortening in offspring. Therefore, we adjusted for these factors, and the results of the analysis revealed that the TL of umbilical cord blood cells in the offspring in the GDM group was still shorter than that of the offspring in the Ctrl group, and there was a negative correlation with blood glucose levels. In a 2014 study by Xu et al., which showed that the offspring of pregnant women with GDM had relatively short telomeres in cord blood (59), although the factors that may affect TL, such as maternal age and neonatal weight, were adjusted for, factors that may differ in the disease characteristics of GDM patients were not adjusted for, so the results obtained are debatable.
In addition, we analysed the sex stratification of neonates and reported a more pronounced trend towards TL shortening in male neonates, which is consistent with the findings of previous studies (60, 61). Similar studies have also shown that elevated maternal blood glucose levels are associated with an increased risk of impaired glucose tolerance in male offspring (62), and there is evidence that TL in boys is closely related to their glucose load response (63), suggesting that sex differences may affect telomere sensitivity to blood glucose and, in turn, health throughout life (63). However, in 2017, Hjort L et al. evaluated the offspring of pregnant women with GDM from 9 to 16 years of age via quantitative PCR and reported that there was no significant difference in the total number of children with GDM, with only the female offspring showing telomere shortening (64). Because telomere changes are affected by many factors, the telomere state from childhood to adolescence may not directly reflect the intrauterine condition, considering the effects of time and the external environment. Although the results of these studies differ, they all point to the hypothesis that GDM creates an unfavourable intrauterine environment or induces changes in the telomere system, which may be a potential basis for future studies of the pathogenesis of metabolic diseases caused by intrauterine GDM exposure. The association between foetal TL and metabolic phenotype may be regulated by the intrauterine environment, sex-specific physiological mechanisms, and sex-specific genetic and epigenetic alterations, and further research is urgently needed to improve the understanding of this phenomenon (65). In addition, we also observed a decrease in umbilical cord blood TE activity in the offspring of pregnant women with GDM compared with Ctrls, and TL was positively correlated with TE activity. However, both were negatively correlated with glycaemic indicators, which reinforces our focus on the role of the hyperglycaemic environment in regulating the telomere/telomerase system given that gestational diabetes is characterized by hyperglycaemia.
There are shortcomings in this study, and we had no mechanisms for further study of telomere shortening. We did not measure TL in the mothers. In addition, there are a variety of methods for the detection of telomere length and telomerase, and these methods have different advantages and disadvantages. For example, FISH technology still has irreplaceable advantages in some specific application scenarios (such as detailed analysis of telomere length of specific chromosomes in a single cell), but qPCR technology has become the preferred method for detecting telomere length in most research and clinical applications due to its advantages of high throughput, low cost, easy operation, high sensitivity and specificity (66–68). In addition, TRAP technology does have more advantages in the in-depth study of the mechanism of telomerase activity, but the ELISA detection technology of telomerase in serum has also been adopted in many articles (69–71). Therefore, the in-depth study of the mechanism of telomerase activity is the focus of our research group, and we will also use multiple detection methods for verification in the follow-up detection to reduce the bias of the results and achieve more accurate research results.However, our research also has advantages; for one of the important factors affecting TL by maternal age, we reduced the age gap as much as possible to homogenize the samples in the initial study object inclusion stage.
Conclusions
Overall, this study revealed that the shortening of TL in neonates born to GDM patients was more pronounced in the male infant population, suggesting that intrauterine exposure to GDM in a poor intrauterine environment increased telomere attrition, which may be one of the key mechanisms linking maternal GDM to the increased risk of metabolic disease in offspring later in life, and further longitudinal association studies are needed.
Data availability statement
The datasets presented in this study can be found in online repositories. The names of the repository/repositories and accession number(s) can be found in the article/supplementary material.
Ethics statement
The studies involving humans were approved by the Ethics Review Committee of Hefei Maternal and Child Health Hospital (YYLL2023-10-08-02). The studies were conducted in accordance with the local legislation and institutional requirements. The participants provided their written informed consent to participate in this study.
Author contributions
SL: Data curation, Formal analysis, Funding acquisition, Project administration, Writing – original draft, Writing – review & editing. LX: Data curation, Investigation, Writing – review & editing. YC: Data curation, Writing – review & editing. DL: Supervision, Writing – review & editing. BZ: Project administration, Supervision, Writing – review & editing. XC: Investigation, Project administration, Supervision, Writing – review & editing. MZ: Formal analysis, Methodology, Supervision, Validation, Writing – review & editing.
Funding
The author(s) declare financial support was received for the research, authorship, and/or publication of this article. Funded by scientific research projects of Anhui Provincial Department of Education (2022AH050674).
Acknowledgments
We sincerely thank our patient for allowing us to publish her clinical data.
Conflict of interest
The authors declare that the research was conducted in the absence of any commercial or financial relationships that could be construed as a potential conflict of interest.
Publisher’s note
All claims expressed in this article are solely those of the authors and do not necessarily represent those of their affiliated organizations, or those of the publisher, the editors and the reviewers. Any product that may be evaluated in this article, or claim that may be made by its manufacturer, is not guaranteed or endorsed by the publisher.
Abbreviations
GDM, Gestational diabetes mellitus; Ctrl, Healthy control; PBMI, Pre-pregnancy body mass index; PWG, Pregnancy weight gain; GA, Gestational age; OGTT FPG, glucose tolerance test and fasting blood glucose; LDL, Low Density Lipoprotein; HDL, High-density lipoprotein; TG, Triglyceride; TC, Total Cholesterol; SBP, Systolic blood pressure; DBP, Diastolic blood pressure; PPH, Postpartum hemorrhage; NBW, Newborn birth weight.
References
1. Saeedi M, Cao Y, Fadl H, Gustafson H, Simmons D. Increasing prevalence of gestational diabetes mellitus when implementing the IADPSG criteria: A systematic review and meta-analysis. Diabetes Res Clin Pract. (2021) 172:108642. doi: 10.1016/j.diabres.2020.108642
2. Wang H, Li N, Chivese T, Werfalli M, Sun H, Yuen L, et al. IDF diabetes atlas: estimation of global and regional gestational diabetes mellitus prevalence for 2021 by international association of diabetes in pregnancy study group’s criteria. Diabetes Res Clin Pract. (2022) 183:109050. doi: 10.1016/j.diabres.2021.109050
3. Nguyen CL, Pham NM, Binns CW, Duong DV, Lee AH. Prevalence of gestational diabetes mellitus in eastern and southeastern asia: A systematic review and meta-analysis. J Diabetes Res. (2018) 2018:6536974. doi: 10.1155/2018/6536974
4. Diagnostic criteria and classification of hyperglycaemia first detected in pregnancy. Geneva: World Health Organization (2013).
5. American Diabetes Association. Diagnosis and classification of diabetes mellitus. Diabetes Care. (2011) 34 Suppl 1:S62–9. doi: 10.2337/dc11-S062
6. Hod M, Kapur A, Sacks DA, Hadar E, Agarwal M, Di Renzo GC. The International Federation of Gynecology and Obstetrics (FIGO) Initiative on gestational diabetes mellitus: A pragmatic guide for diagnosis, management, and care. Int J Gynaecol Obstet. (2015) 131 Suppl 3:S173–211. doi: 10.1016/S0020-7292(15)30007-2
7. Zhu WW, Yang HX, Wang C, Su RN, Feng H, Kapur A. High prevalence of gestational diabetes mellitus in beijing: effect of maternal birth weight and other risk factors. Chin Med J (Engl). (2017) 130:1019–25. doi: 10.4103/0366-6999.204930
8. Wang C, Jin L, Tong M, Zhang J, Yu J, Meng W, et al. Prevalence of gestational diabetes mellitus and its determinants among pregnant women in Beijing. J Matern Fetal Neonatal Med. (2022) 35:1337–43. doi: 10.1080/14767058.2020.1754395
9. Gao C, Sun X, Lu L, Liu F, Yuan J. Prevalence of gestational diabetes mellitus in mainland China: A systematic review and meta-analysis. J Diabetes Investig. (2019) 10:154–62. doi: 10.1111/jdi.2019.10.issue-1
10. Barker DJ, Osmond C, Law CM. The intrauterine and early postnatal origins of cardiovascular disease and chronic bronchitis. J Epidemiol Community Health. (1989) 43:237–40. doi: 10.1136/jech.43.3.237
11. ACOG practice bulletin no. 190: gestational diabetes mellitus. Obstet Gynecol. (2018) 131:e49–64. doi: 10.1097/AOG.0000000000002501
12. Ye W, Luo C, Huang J, Li C, Liu Z, Liu F. Gestational diabetes mellitus and adverse pregnancy outcomes: systematic review and meta-analysis. BMJ. (2022) 377:e067946. doi: 10.1136/bmj-2021-067946
13. Karkia R, Giacchino T, Shah S, Gough A, Ramadan G, Akolekar R. Gestational diabetes mellitus: association with maternal and neonatal complications. Medicina (Kaunas). (2023) 59:2096. doi: 10.3390/medicina59122096
14. Johns EC, Denison FC, Norman JE, Reynolds RM. Gestational diabetes mellitus: mechanisms, treatment, and complications. Trends Endocrinol Metab. (2018) 29:743–54. doi: 10.1016/j.tem.2018.09.004
15. Moon JH, Jang HC. Gestational diabetes mellitus: diagnostic approaches and maternal-offspring complications. Diabetes Metab J. (2022) 46:3–14. doi: 10.4093/dmj.2021.0335
16. Thakur A, Agrawal S, Chakole S, Wandile B. A critical review of diagnostic strategies and maternal offspring complications in gestational diabetes mellitus. Cureus. (2023) 15:e51016. doi: 10.7759/cureus.51016
17. Nakshine VS, Jogdand SD. A comprehensive review of gestational diabetes mellitus: impacts on maternal health, fetal development, childhood outcomes, and long-term treatment strategies. Cureus. (2023) 15:e47500. doi: 10.7759/cureus.47500
18. Geelhoed JJ, Fraser A, Tilling K, Benfield L, Davey Smith G, Sattar N, et al. Preeclampsia and gestational hypertension are associated with childhood blood pressure independently of family adiposity measures: the Avon Longitudinal Study of Parents and Children. Circulation. (2010) 122:1192–9. doi: 10.1161/CIRCULATIONAHA.110.936674
19. Lawlor DA, Fraser A, Lindsay RS, Ness A, Dabelea D, Catalano P, et al. Association of existing diabetes, gestational diabetes and glycosuria in pregnancy with macrosomia and offspring body mass index, waist and fat mass in later childhood: findings from a prospective pregnancy cohort. Diabetologia. (2010) 53:89–97. doi: 10.1007/s00125-009-1560-z
20. Clausen TD, Mathiesen ER, Hansen T, Pedersen O, Jensen DM, Lauenborg J, et al. High prevalence of type 2 diabetes and pre-diabetes in adult offspring of women with gestational diabetes mellitus or type 1 diabetes: the role of intrauterine hyperglycemia. Diabetes Care. (2008) 31:340–6. doi: 10.2337/dc07-1596
21. Dabelea D, Hanson RL, Lindsay RS, Pettitt DJ, Imperatore G, Gabir MM, et al. Intrauterine exposure to diabetes conveys risks for type 2 diabetes and obesity: a study of discordant sibships. Diabetes. (2000) 49:2208–11. doi: 10.2337/diabetes.49.12.2208
22. Sobngwi E, Boudou P, Mauvais-Jarvis F, Leblanc H, Velho G, Vexiau P, et al. Effect of a diabetic environment in utero on predisposition to type 2 diabetes. Lancet. (2003) 361:1861–5. doi: 10.1016/S0140-6736(03)13505-2
23. Boerschmann H, Pflüger M, Henneberger L, Ziegler AG, Hummel S. Prevalence and predictors of overweight and insulin resistance in offspring of mothers with gestational diabetes mellitus. Diabetes Care. (2010) 33:1845–9. doi: 10.2337/dc10-0139
24. Patel S, Fraser A, Davey Smith G, Lindsay RS, Sattar N, Nelson SM, et al. Associations of gestational diabetes, existing diabetes, and glycosuria with offspring obesity and cardiometabolic outcomes. Diabetes Care. (2012) 35:63–71. doi: 10.2337/dc11-1633
25. Temple RC, Aldridge VJ, Murphy HR. Prepregnancy care and pregnancy outcomes in women with type 1 diabetes. Diabetes Care. (2006) 29:1744–9. doi: 10.2337/dc05-2265
26. Petry CJ, Hales CN. Long-term effects on offspring of intrauterine exposure to deficits in nutrition. Hum Reprod Update. (2000) 6:578–86. doi: 10.1093/humupd/6.6.578
27. Davis EF, Newton L, Lewandowski AJ, Lazdam M, Kelly BA, Kyriakou T, et al. Pre-eclampsia and offspring cardiovascular health: mechanistic insights from experimental studies. Clin Sci (Lond). (2012) 123:53–72. doi: 10.1042/CS20110627
28. Xu J, Ye J, Wu Y, Zhang H, Luo Q, Han C, et al. Reduced fetal telomere length in gestational diabetes. PloS One. (2014) 9:e86161. doi: 10.1371/journal.pone.0086161
30. Blackburn EH. Structure and function of telomeres. Nature. (1991) 350:569–73. doi: 10.1038/350569a0
31. Frenck RW Jr, Blackburn EH, Shannon KM. The rate of telomere sequence loss in human leukocytes varies with age. Proc Natl Acad Sci U.S.A. (1998) 95:5607–10. doi: 10.1073/pnas.95.10.5607
32. Olovnikov AM. A theory of marginotomy: the incomplete copying of template margin inenzymic synthesis of polynucleotides and biological significance of the phenomenon. J Theor Biol. (1973) 41:181–90. doi: 10.1016/0022-5193(73)90198-7
33. Bojesen SE. Telomeres and human health. J Intern Med. (2013) 274:399–413. doi: 10.1111/joim.2013.274.issue-5
34. Aviv A, Chen W, Gardner JP, Kimura M, Brimacombe M, Cao X, et al. Leukocyte telomere dynamics: longitudinal findings among young adults in the Bogalusa Heart Study. Am J Epidemiol. (2009) 169:323–9. doi: 10.1093/aje/kwn338
35. Matsubara Y, Murata M, Yoshida T, Watanabe K, Saito I, Miyaki K, et al. Telomere length of normal leukocytes is affected by a functional polymorphism of hTERT. Biochem Biophys Res Commun. (2006) 341:128–31. doi: 10.1016/j.bbrc.2005.12.163
36. Iizuka T, Sawabe M, Takubo K, Liu M, Homma Y, Suzuki M, et al. hTERT promoter polymorphism, -1327C>T, is associated with the risk of epithelial cancer. Springerplus. (2013) 2:249. doi: 10.1186/2193-1801-2-249
37. Greider CW, Blackburn EH. Identification of a specific telomere terminal transferase activity in Tetrahymena extracts. Cell. (1985) 43:405–13. doi: 10.1016/0092-8674(85)90170-9
38. Harrington L, McPhail T, Mar V, Zhou W, Oulton R, Bass MB, et al. A mammalian telomerase-associated protein. Science. (1997) 275:973–77. doi: 10.1126/science.275.5302.973
39. Cawthon RM. Telomere measurement by quantitative PCR. Nucleic Acids Res. (2002) 30:e47. doi: 10.1093/nar/30.10.e47
40. Gil ME, Coetzer TL. Real-time quantitative PCR of telomere length. Mol Biotechnol. (2004) 27:169–72. doi: 10.1385/MB:27:2:169
41. Cross JA, Temple RC, Hughes JC, Dozio NC, Brennan C, Stanley K, et al. Cord blood telomere length, telomerase activity and inflammatory markers in pregnancies in women with diabetes or gestational diabetes. Diabetes Med. (2010) 27:1264–70. doi: 10.1111/j.1464-5491.2010.03099.x
42. Kamath U, Rao G, Raghothama C, Rai L, Rao P. Erythrocyte indicators of oxidative stress in gestational diabetes. Acta Paediatr. (1998) 87:676–9. doi: 10.1111/j.1651-2227.1998.tb01529.x
43. Kinalski M, Sledziewski A, Telejko B, Kowalska I, Kretowski A, Zarzycki W, et al. Lipid peroxidation, antioxidant defence and acid-base status in cord blood at birth: the influence of diabetes. Horm Metab Res. (2001) 33:227–31. doi: 10.1055/s-2001-14953
44. Sobki SH, Al-Senaidy AM, Al-Shammari TA, Inam SS, Al-Gwiser AA, Bukhari SA. Impact of gestational diabetes on lipid profiling and indices of oxidative stress in maternal and cord plasma. Saudi Med J. (2004) 25:876–80.
45. Codd V, Nelson CP, Albrecht E, Mangino M, Deelen J, Buxton JL, et al. Identification of seven loci affecting mean telomere length and their association with disease. Nat Genet. (2013) 45:422–427e4272. doi: 10.1038/ng.2528
46. Campisi J. Cellular senescence: putting the paradoxes in perspective. Curr Opin Genet Dev. (2011) 21:107–12. doi: 10.1016/j.gde.2010.10.005
47. Oikawa S, Kawanishi S. Site-specific DNA damage at GGG sequence by oxidative stress may accelerate telomere shortening. FEBS Lett. (1999) 453:365–8. doi: 10.1016/S0014-5793(99)00748-6
48. Aubert G, Lansdorp PM. Telomeres and aging. Physiol Rev. (2008) 88:557–79. doi: 10.1152/physrev.00026.2007
49. Turner KJ, Vasu V, Griffin DK. Telomere biology and human phenotype. Cells. (2019) 8:73. doi: 10.3390/cells8010073
50. Arsenis NC, You T, Ogawa EF, Tinsley GM, Zuo L. Physical activity and telomere length: Impact of aging and potential mechanisms of action. Oncotarget. (2017) 8:45008–19. doi: 10.18632/oncotarget.16726
51. Sharma R, Diwan B. Lipids and the hallmarks of ageing: From pathology to interventions. Mech Ageing Dev. (2023) 215:111858. doi: 10.1016/j.mad.2023.111858
52. Chen Z, Chen Z, Jin X. Mendelian randomization supports causality between overweight status and accelerated aging. Aging Cell. (2023) 22:e13899. doi: 10.1111/acel.13899
53. Martens DS, Plusquin M, Gyselaers W, De Vivo I, Nawrot TS. Maternal pre-pregnancy body mass index and newborn telomere length. BMC Med. (2016) 14:148. doi: 10.1186/s12916-016-0689-0
54. Müezzinler A, Zaineddin AK, Brenner H. Body mass index and leukocyte telomere length in adults: a systematic review and meta-analysis. Obes Rev. (2014) 15:192–201. doi: 10.1111/obr.12126
55. Gao X, Kong Y, Li S, Dong S, Huang X, Qi D, et al. Intermediate effects of body mass index and C-reactive protein on the serum cotinine-leukocyte telomere length association. Front Aging Neurosci. (2022) 13:827465. doi: 10.3389/fnagi.2021.827465
56. Gielen M, Hageman GJ, Antoniou EE, Nordfjall K, Mangino M, Balasubramanyam M, et al. Body mass index is negatively associated with telomere length: a collaborative cross-sectional meta-analysis of 87 observational studies. Am J Clin Nutr. (2018) 108:453–75. doi: 10.1093/ajcn/nqy107
57. Rode L, Nordestgaard BG, Weischer M, Bojesen SE. Increased body mass index, elevated C-reactive protein, and short telomere length. J Clin Endocrinol Metab. (2014) 99:E1671–5. doi: 10.1210/jc.2014-1161
58. Guzzardi MA, Iozzo P, Salonen MK, Kajantie E, Eriksson JG. Maternal adiposity and infancy growth predict later telomere length: a longitudinal cohort study. Int J Obes (Lond). (2016) 40:1063–9. doi: 10.1038/ijo.2016.58
59. Maugeri A, Magnano San Lio R, La Rosa MC, Giunta G, Panella M, Cianci A, et al. The relationship between telomere length and gestational weight gain: findings from the mamma & Bambino cohort. Biomedicines. (2021) 10:67. doi: 10.3390/biomedicines10010067
60. Gardner M, Bann D, Wiley L, Cooper R, Hardy R, Nitsch D, et al. Gender and telomere length: systematic review and meta-analysis. Exp Gerontol. (2014) 51:15–27. doi: 10.1016/j.exger.2013.12.004
61. Ly K, Walker C, Berry S, Snell R, Marks E, Thayer Z, et al. Telomere length in early childhood is associated with sex and ethnicity. Sci Rep. (2019) 9:10359. doi: 10.1038/s41598-019-46338-x
62. Tam WH, Ma RCW, Ozaki R, Li AM, Chan MHM, Yuen LY, et al. In utero exposure to maternal hyperglycemia increases childhood cardiometabolic risk in offspring. Diabetes Care. (2017) 40:679–86. doi: 10.2337/dc16-2397
63. Wong KK, Cheng F, Lim CKP, Tam CHT, Tutino G, Yuen LY, et al. Early emergence of sexual dimorphism in offspring leukocyte telomere length was associated with maternal and children’s glucose metabolism-a longitudinal study. BMC Med. (2022) 20:490. doi: 10.1186/s12916-022-02687-5
64. Hjort L, Vryer R, Grunnet LG, Burgner D, Olsen SF, Saffery R, et al. Telomere length is reduced in 9- to 16-year-old girls exposed to gestational diabetes in utero. Diabetologia. (2018) 61:870–80. doi: 10.1007/s00125-018-4549-7
65. Sandovici I, Fernandez-Twinn DS, Hufnagel A, Constância M, Ozanne SE. Sex differences in the intergenerational inheritance of metabolic traits. Nat Metab. (2022) 4:507–23. doi: 10.1038/s42255-022-00570-4
66. Chen X, Zeng C, Gong C, Zhang L, Wan Y, Tao F. Associations between early life parent-child separation and shortened telomere length and psychopathological outcomes during adolescence. Psychoneuroendocrinology. (2019) 103:195–202. doi: 10.1016/j.psyneuen.2019.01.021
67. Sun Y, Fang J, Wan Y, Su P, Tao F. Association of early-life adversity with measures of accelerated biological aging among children in China. JAMA Netw Open. (2020) 3:e2013588. doi: 10.1001/jamanetworkopen.2020.13588
68. Ojeda-Rodriguez A, Rangel-Zuñiga OA, Arenas-de Larriva AP, Gutierrez-Mariscal FM, Torres-Peña JD, Romero-Cabrera JL. Telomere length as biomarker of nutritional therapy for prevention of type 2 diabetes mellitus development in patients with coronary heart disease: CORDIOPREV randomised controlled trial. Cardiovasc Diabetol. (2024) 23:98. doi: 10.1186/s12933-024-02175-5
69. Lv BB, Yang CL, Tan ZX, Zheng L, Li MD, Jiang YL. Association between cadmium exposure and pulmonary function reduction: Potential mediating role of telomere attrition in chronic obstructive pulmonary disease patients. Ecotoxicol Environ Saf. (2023) 251:114548. doi: 10.1016/j.ecoenv.2023.114548
70. Zhao Y, Bai X, Jia X, Lu Y, Cheng W, Shu M, et al. Age-related changes of human serum Sirtuin6 in adults. BMC Geriatr. (2021) 21:452. doi: 10.1186/s12877-021-02399-0
Keywords: TL, telomerase, TE, telomere length, gestational diabetes mellitus, GDM
Citation: Liu S, Xu L, Cheng Y, Liu D, Zhang B, Chen X and Zheng M (2024) Decreased telomerase activity and shortened telomere length in infants whose mothers have gestational diabetes mellitus and increased severity of telomere shortening in male infants. Front. Endocrinol. 15:1490336. doi: 10.3389/fendo.2024.1490336
Received: 03 September 2024; Accepted: 02 December 2024;
Published: 16 December 2024.
Edited by:
Charles W. Putnam, University of Arizona, United StatesReviewed by:
Deborah Ramini, Italian National Research Center on Aging (INRCA-IRCCS) (Ancona), ItalyRanjodh Singh Sandhu, National Institutes of Health (NIH), United States
Copyright © 2024 Liu, Xu, Cheng, Liu, Zhang, Chen and Zheng. This is an open-access article distributed under the terms of the Creative Commons Attribution License (CC BY). The use, distribution or reproduction in other forums is permitted, provided the original author(s) and the copyright owner(s) are credited and that the original publication in this journal is cited, in accordance with accepted academic practice. No use, distribution or reproduction is permitted which does not comply with these terms.
*Correspondence: Shuhua Liu, bGl1c2h1aHVhMDIyNUAxNjMuY29t; Bin Zhang, emhhbmdiaW5zaGFubXVAMTI2LmNvbQ==; Xianxia Chen, Q2hlbnh4MzkxQHNvaHUuY29t; Mingming Zheng, bV9tX3poZW5nQDE2My5jb20=
†These authors have contributed equally to this work