- 1Laboratorio de Alteraciones Reproductivas y Metabólicas, Instituto de Fisiología, Facultad de Ciencias, Universidad de Valparaíso, Valparaíso, Chile
- 2Center for Neurobiochemical Studies in Endocrine Diseases, Laboratory of Neurobiochemistry, Department of Biochemistry and Molecular Biology, Faculty of Chemistry and Pharmaceutical Sciences, Universidad de Chile, Santiago, Chile
- 3Laboratorio de Química Biológica, Instituto de Química, Pontificia Universidad Católica de Valparaíso, Valparaíso, Chile
- 4Univ. Lille, Inserm, CHU Lille, Laboratory of Development and Plasticity of the Neuroendocrine Brain, Lille Neuroscience & Cognition, UMR-S1172, EGID, DISTALZ, Lille, France
- 5Centro de Investigación Biomédica y Aplicada (CIBAP), Escuela de Medicina, Facultad de Ciencias Médicas, Universidad de Santiago de Chile, Santiago, Chile
The link between metabolism and reproduction is well-known. Both undernutrition and obesity affect the reproductive system. Metabolic status influences reproductive physiology by regulating gonadotropin secretion and affecting reproductive organs through hormonal signals. On the other hand, the autonomic nervous system controls follicle development and ovulation in the female reproductive system. This system is regulated by hypothalamic areas associated with metabolism as the Arcuate nuclei (ARC) and paraventricular nuclei (PVN). Metabolic signals, such as nutrients and hormones, acting on the hypothalamus may play a crucial role in modulating sympathetic innervation of the ovary and other reproductive organs. Some of these hormones are leptin, insulin, and GLP-1 that act directly in the hypothalamus to activate the sympathetic nervous system. In this minireview, we propose that leptin could be an important regulator of sympathetic innervation in reproductive tissues. Leptin may affect the density or activity of sympathetic nerves, thereby affecting reproductive function. We also speculate that other hormones such as insulin and GLP-1 may activate sympathetic nerves to the ovary. Additionally, we explore how early-onset obesity can cause lasting changes in the autonomic control of metabolic and reproductive organs, especially in the ovary. This suggests that the hyperactivation of sympathetic nerves in adulthood, due to metabolic programming, could be a possible cause of reproductive and metabolic disorders, such as polycystic ovary syndrome.
1 Introduction: an overview of ovarian physiology
The ovary, the organ housing female gametes, consists of ovarian follicles that serve as functional structures. Each follicle contains an oocyte surrounded by a stratified epithelium called the granulosa layer. Outside this layer lies a connective tissue layer, called the theca layer, enveloping the follicle. Both granulosa and theca cells provide nutrients and trophic support to the oocyte and produce steroid and peptide hormones that regulate various bodily functions. The process by which a follicle grows and matures is termed folliculogenesis or follicular development.
The follicle begins as an oocyte surrounded by a single layer of pregranulosa cells, forming what is known as the primordial follicle. The number of primordial follicles represents a female’s ‘follicular reserve,’ which reflects her potential reproductive capacity. In women, follicle formation occurs during gestation, while in rodents, during early postnatal life (until PND4) (1). These follicles are recruited for growth throughout life until depleted (2). The sequential transition of primordial follicles into primary, secondary, and tertiary (antral) follicles, with some selected to ovulate, is the process that determines reproductive capacity. After ovulation, the selected follicles transform into corpora lutea through the luteinization of granulosa and theca cells. All non-selected follicles are discarded via atresia, a controlled mechanism of cell death (2).
In the ovary, follicles and corpora lutea are embedded in a connective tissue rich in extracellular matrix, known as the stroma. The stroma is currently an active object of study for its diverse properties beyond just supporting the follicles. Blood vessels and nerves enter the ovary through the hilum and interweave within the stroma surrounding the follicles (3). Ovarian function, including follicle development and cyclic hormone production, relies on several internal feedback mechanisms (4), and environmental cues. In this sense, the sympathetic nervous system is a key player since it responds to various stimuli such as stress by cold exposure (5) or even the metabolic status of the body (6). In the following section, we will describe the sympathetic innervation of the ovary and provide an overview of the physiological action of this extrinsic ovarian innervation.
2 Sympathetic innervation of the ovary
It is well established that the ovary is innervated by autonomic nerves. The parasympathetic nerves from the Vagus nerve innervate the blood vessels. However, there is also compelling evidence of a complete and functional intracrine cholinergic system within the organ (7). In rats, sympathetic nerves reach the ovary primarily through the superior ovarian nerve (SON) and the ovarian plexus (OP) (8). The SON emerges from the suprarenal ganglion, but the SON also communicates with the ovaries through the celiac ganglion (CG), superior mesenteric ganglion (SMG), and stellate ganglion (SG), suggesting that both autonomic and sensory information from the ovaries is processed in these three ganglia (8).
Adrenergic receptors are present in the ovarian blood vessels and the follicular structures. While α-1 adrenergic receptor predominates in blood vessels, β-2 adrenergic receptors are mainly found in granulosa or luteinized cells (9). Sympathetic nerves and adrenergic receptors in the rat ovary are present from neonatal age; however, the entire functional development of these nerves occurs near puberty (10). In this sense, before puberty, sympathetic nerve release is basal and calcium-independent, while after puberty, the norepinephrine release is mostly vesicular and calcium-dependent.
Several in vitro, ex vivo and in vivo studies have demonstrated that adrenergic receptor pharmacological activation or blockade can modify sex hormone secretion in the ovary. For example, incubation of ovaries with the selective β-2 adrenergic agonist terbutaline increases cAMP production and induces progesterone secretion. This effect is inhibited by propranolol, a non-selective β-adrenergic antagonist, and butoxamine, a selective β-2 adrenergic antagonist, but not by practolol, a selective β-1 adrenergic antagonist (11). Additionally, isoproterenol, a non-selective β-adrenergic agonist, amplifies the response of theca-interstitial cells to hCG, thereby increasing the release of androstenedione (12).
The sympathetic nervous system (SNS) profoundly influences the regulation of follicular development. Initially, follicle development is independent of gonadotropins. Instead, neurotransmitters such as norepinephrine and vasoactive intestinal peptide (VIP) stimulate the expression of follicle-stimulating hormone receptors (FSHR) in small follicles, priming them for subsequent recruitment by FSH (13). The guanethidine-mediated sympathetic denervation alters follicle dynamics by retarding the progression of follicles, leading to abnormal estrous cycles (14). Also, the surgical denervation of the SON leads to abnormal follicle development and impaired steroid production (15). This underscores the critical role of the sympathetic nervous system in these crucial aspects of ovarian function.
In an early study conducted by Gerendai et al., using retrograde viral tracers, the authors mapped a complex neural pathway that originates in the hypothalamus, descends through the brainstem and spinal cord, and ultimately innervates the ovarian tissue via postganglionic sympathetic nerves. The study involved the injection of the pseudorabies virus into the ovary, followed by an examination of the spinal cord and brain for infected neurons. Virus-labeled nerve cells were identified using immunocytochemical techniques, revealing a polysynaptic neural route that connects the ovary to the central nervous system (CNS) and providing insights into the CNS cell groups responsible for regulating the activity of ovarian innervation (16). Interestingly, the hypothalamus, specifically the paraventricular nucleus (PVN), is one of the regions of the brain that were extensively immunolabelled, showing that PVN neurons regulate the sympathetic innervation to the ovary. The PVN is a highly integrative nucleus weighing the magnitude of different external stimuli to integrate a physiological response through the sympathetic nervous system.
3 Hypothalamic control of the ovarian sympathetic innervation
The PVN is a crucial region that regulates SNS activity. Often referred to as the “autonomic master controller” (17), the PVN contains two central neuronal regions: magnocellular and parvocellular. Magnocellular neurons project the posterior pituitary gland and secrete hormones such as oxytocin and vasopressin directly into the bloodstream. In contrast, parvocellular neurons can be divided into two distinct populations. One group consists of neurosecretory cells that project to the median eminence, where they release neuropeptides like corticotropin-releasing hormone (CRH) and thyrotropin-releasing hormone (TRH) into the hypothalamic-pituitary portal system. The other is the parvocellular population, which includes pre-autonomic cells that project to the medulla and spinal cord, allowing them to regulate autonomic nervous system functions (18). Some parvocellular neurons in the PVN also synthesize and release oxytocin and vasopressin (19, 20).
The PVN parvocellular neurons project to several pre-autonomic relay stations, such as the motor pressor nucleus of the rostral ventrolateral medulla (RVLM) and the Nucleus of the solitary tract (NTS). These second-order neurons in the RVLM connect with sympathetic preganglionic neurons (SPNs) in the thoracic and lumbar intermediolateral nucleus (IML) and from there to the SNS nerves in the kidneys and cardiovascular system (21, 22). Although most of the pre-autonomic neurons in the paraventricular nucleus (PVN) project to the RVLM and then to the IML (22) some of them connect directly the NTS. Mutual connections exist between the PVN and the NTS, which can control RVLM activity (23). The NTS is also involved in parasympathetic control, and evidence supports the role of PVN in controlling the RVLM by inhibiting parasympathetic effects, resulting in increased sympathetic output (24). Some spinally projecting pre-autonomic neurons (SPANs), project directly to the intermediolateral spinal columns in the IML, a central integration center and origin of motor sympathetic preganglionic neurons (SPNs) that descend ipsilaterally through the brainstem and spinal cord (21, 22, 25). The last group of pre-autonomic parvocellular neurons from the PVN innervates SPNs in the IML and sends collaterals to the RVLM, potentially having a dual role in controlling SNS output (22).
The PVN plays a role in the polysynaptic pathway that controls ovarian sympathetic innervation, which is of particular significance. This function emphasizes the broad influence of PVN on physiological processes. Specifically, thyrotropin-releasing hormone (TRH)-expressing neurons within the PVN are essential for mediating the activation of sympathetic nerves innervating the ovary (26–29). Hypothalamic manipulation of ceramides during early life in the PVN influences ovarian function without affecting GnRH control but instead influences changes in the maturation of the sympathetic nervous system in the ovary (30). This role of the PVN in controlling ovarian sympathetic innervation is a key area of research in the field of neuroendocrinology, and its implications are far-reaching.
The hypothalamic PVN receives diverse inputs from various brain regions, including the prefrontal cortex, amygdala, locus coeruleus, and hippocampus, as well as other hypothalamic nuclei such as the arcuate nucleus (ARC), ventromedial nucleus (VMN), dorsomedial nucleus (DMN), and lateral hypothalamic area (LHA) (17, 20). These inputs relay information about the bodily physiological state to the PVN. The ARC plays a central role in sensing and integrating metabolic signals related to the body’s energy status and nutrient availability (31). Two key neuron populations within the ARC detect and relay this information. POMC/CART neurons, which express pro-opiomelanocortin and cocaine- and amphetamine-regulated transcript, are activated by signals of energy sufficiency, such as elevated levels of nutrients and hormones like leptin and insulin. Activation of POMC/CART leads to the release of the neurohormone alpha-Melanocyte-stimulating hormone (α-MSH) in the PVN, promoting satiety, suppressing appetite, and increasing the activity of sympathetic nerves (32, 33). In contrast, NPY/AgRP neurons, which express neuropeptide Y and agouti-related peptide, are activated by signals of energy deficiency, such as low levels of nutrients and hormones. Activation of NPY/AgRP neurons stimulates appetite, promotes food-seeking behaviors, and decreases cardiovascular sympathetic activity and thermogenesis (34, 35). Taking together, through activating POMC/CART neurons or inhibiting NPY/AgRP neurons, leptin, insulin or other signals could activate TRH-ergic and other neurons in the PVN, which may impact the sympathetic regulation of the ovary.
4 Leptin control of sympathetic innervation
4.1 Leptin mechanism and site of action within the hypothalamus
Leptin is a hormone primarily secreted by the adipocytes of white adipose tissue that plays a crucial role in controlling food intake and energy expenditure. Leptin receptors (LepR), classified as class I cytokine receptors, including six splice variants (ObRa-f) identified to date. These receptors are expressed in various nuclei of the hypothalamus, including the ARC, VMH, LHA, and PVN, as well as extra-hypothalamic regions in the brain (36). However, leptin’s primary signaling mechanism is via the long isoform of its receptor (ObRb). Many animal models of monogenic obesity are linked to mutations in leptin or its receptors. The obese phenotype of ob/ob mice results from single mutations in leptin gene. Likewise, mutations in the leptin receptor gene explain the obese phenotypes of db/db mice, fa/fa Zucker rats, and fak/fak Koletsky rats.
Leptin reaches the brain via specific transport mediated by the LepR. In the mediobasal hypothalamus, leptin crosses the blood-cerebrospinal fluid barrier through an active transport mediated by tanycytes (37). Additionally, in other areas of the brain, leptin crosses endothelial cells lining microvessels and epithelial cells of the choroid plexus to regulate food reward rather than the homeostatic control of feeding (38).
The functions of leptin in the brain are diverse and critical. One of its key roles in maintaining energy homeostasis is the inhibition of NPY/AgRP neurons and activation of POMC/CART neurons in the ARC. POMC/CART neurons project their fibers to the PVN, where they release both α-MSH and CART. These neuropeptides not only lead to satiety but also activate the sympathetic nervous system (39, 40). This activation promotes lipolysis in white adipose tissue (WAT) (40) and thermogenesis in brown adipose tissue (BAT) (41), demonstrating the profound impact of leptin on bodily physiological processes.
4.2 Leptin action on the sympathetic nervous system
The catabolic function of leptin through activating the SNS is relatively predictable from a homeostatic viewpoint, as an increase in leptin due to fat accumulation leads the organism to be more prone to thermogenesis and fat catabolism (41–43). However, intracerebroventricular (ICV) leptin injection also increases sympathetic nerve activity in other organs such as kidneys, thus increasing blood pressure (44). In addition, ICV leptin injection increases norepinephrine levels in the liver and the ovary (45). In this sense, leptin control of sympathetic activity goes beyond its role in energy homeostasis, acting as a periphery-brain signal for other physiological functions.
Different nuclei of the hypothalamus have different influences on sympathetic innervation and several studies have shown this difference depending on the nuclei in which leptin was microinjected. Leptin microinjection into the ARC, but not into VMH, increased hepatic sympathetic activity conveying specifically in phosphatidylinositol 3-kinase (PI3K) but not on AMP-activated protein kinase (AMPK), STAT3, or ERK1/2 pathways (46). Interestingly, the blockade of PI3K prevents leptin-induced sympathetic activation to the kidney but not in BAT, lumbar, or adrenal glands (47). Furthermore, ICV administration of leptin activates hepatic AMPK through sympathetic nerves, but hepatic vagotomy does not affect this activation (48). In contrast, chemical sympathectomy inhibited α1-adrenergic receptors-induced AMPK activation in the liver induced by leptin (48), differing on the lipolytic effects of leptin in WAT, where β-adrenergic receptors are required (42). On the other side, the blockade of ERK1/2 eliminates leptin-induced increases in sympathetic nerve activity in the BAT. However, it does not alter the stimulatory effects of leptin on sympathetic nerve activity in the kidney, lumbar, or adrenal gland (47). Another study showed that microinjection of leptin into the commissural and medial subnuclei of the caudal NTS increased renal but not BAT sympathetic nerve activity (49).
We have recently shown that sub-chronic ICV leptin injection increases norepinephrine levels in the ovary (45). Since TRH release from the magnocellular PVN induces the activation of the SNS nerves arriving into the ovary (29), our results suggest that leptin-mediated activation of TRH neurons in the PVN could be the source of the leptin-induced release of norepinephrine in the ovary. However, it remains unclear whether leptin directly activates the TRH-ergic neurons, or if it does it indirectly by activating POMC neurons in the ARH, or possibly both. The latter possibility is plausible, as both hypophysiotropic and pre-autonomic TRH-ergic neurons of the PVN are innervated by POMC/CART and NPY/AgRP neurons of the ARC (50). Interestingly, the knockdown of LepR in this area does not decrease the tonic regulation of cardiovascular function via the sympathetic nervous system, but it does stimulate food intake (51). Finally, obesity may alter the PVN-SNS-ovary pathway through increasing leptin. Indeed, we observed that early-onset obesity in rats increases NE levels in the ovary when they are adults, which occurs alongside high leptin levels (45). This same pathway may be involved in the precocious puberty induced by obesity (30).
Collectively, these studies underscore the intricate and multifaceted nature of brain leptin action and its diverse physiological effects. Leptin triggers different signaling pathways at the hypothalamus and the leptin-dependent SNS activation in different tissues depends on different hypothalamic nuclei. Figure 1 illustrates a proposed model of how Leptin, through its action on the brain, controls ovarian function.
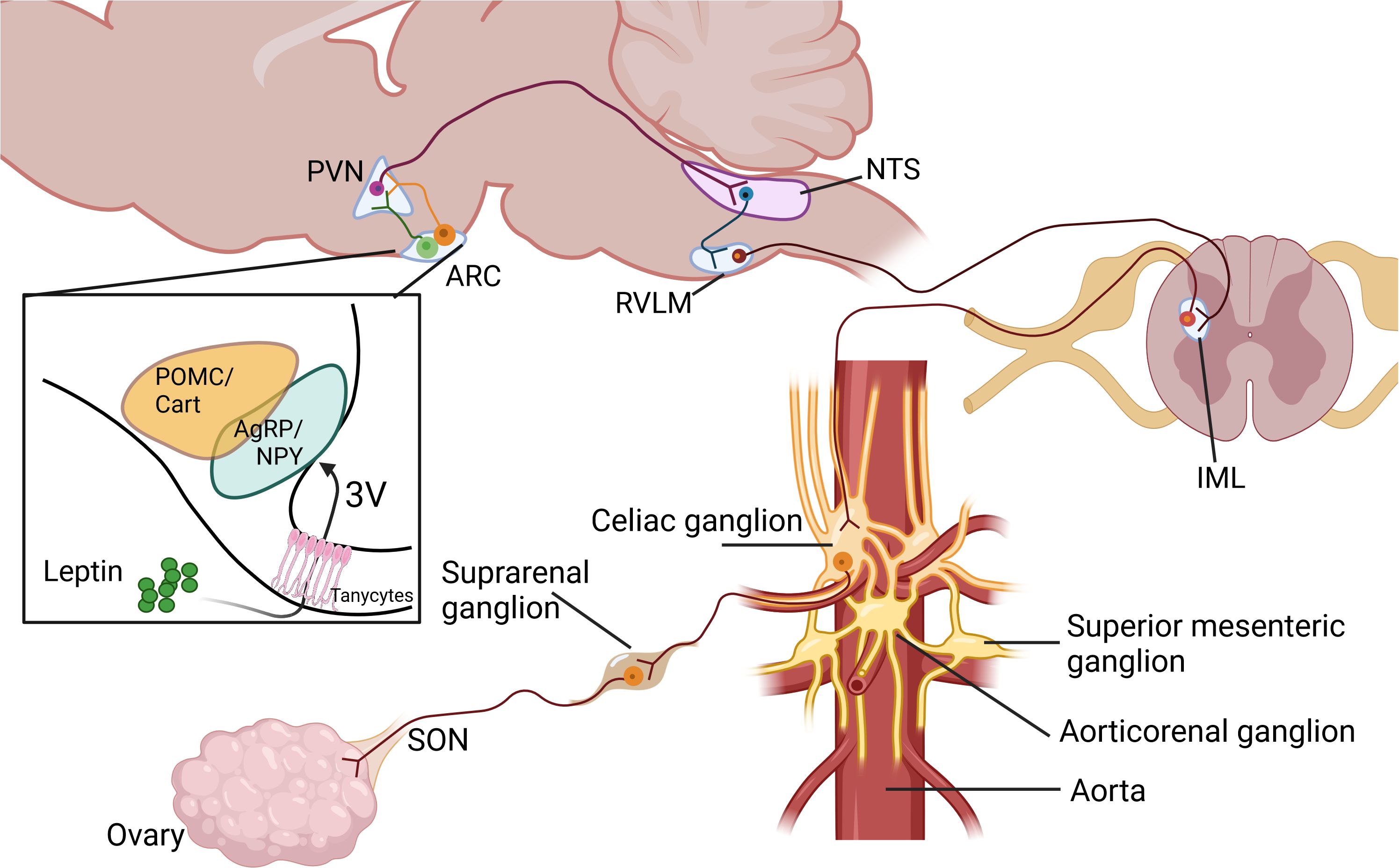
Figure 1. Proposed pathways in the control of ovarian function by leptin through the sympathetic nervous system. Paraventricular nucleus of the hypothalamus (PVN), Arcuate nucleus of the hypothalamus (ARC), Nucleus of the solitary tract (NTS), Rostral ventrolateral medulla (RVLM), Intermediolateral cell column at spinal cord (IML), Superior Ovarian Nerve (SON). Created in BioRender. Fernandois, D. (2025) https://BioRender.com/x73b564.
4.3 Developmental reprogramming of sympathetic innervation by maternal obesity: role of leptin
Maternal obesity during pregnancy and lactation can affect hormone secretion, metabolite concentrations, and the availability of nutrients to the fetus or infant. Consequently, these hormones, metabolites, and nutrients can impact the development of the offspring’s organs. We and other groups have shown that the offspring of obese mothers have elevated plasmatic levels of leptin during infancy (52, 53). Interestingly, leptin is not effective in reducing milk consumption during infancy in rats (54), but it does impact metabolic rate. Notably, leptin functions as a neurotrophic factor during brain neurodevelopment, rather than solely regulating energy balance (55, 56). Since hypothalamic circuits controlling energy balance mature during infancy (57), increased levels of neurotrophic factors such as estradiol or leptin during this critical period can negatively impact the development of hypothalamic circuits controlling energy balance and the hypothalamic connections to the SNS. In this context, previous research has shown that the offspring of rats fed a high-fat diet during pregnancy and lactation, which models maternal obesity, exhibit increased estradiol (58) and leptin levels (52, 53) during early development. This model also exhibits increased norepinephrine levels in the ovary (52) and kidneys (59) in the offspring. Therefore, leptin levels during postnatal development may be critical for correctly establishing hypothalamic regulation of the sympathetic outflow to the organs. In other words, the offspring of obese mothers may have an elevated set-point regulation for the sympathetic tone and increased innervation of these organs due to increased leptin levels during infancy. That leads to a higher susceptibility to developing chronic diseases when exposed to environmental challenges (i.e., stress, overfeeding) during adulthood. Indeed, offspring of obese mothers have increased risk factors for developing hypertension (59), polycystic ovaries (52, 58), and non-alcoholic fatty liver disease (60), all pathologies that are associated with hyperactivation of the SNS.
5 A potential role of hormones beyond leptin in hypothalamic modulation of sympathetic pathways to the ovary
5.1 Insulin
Insulin is released from the pancreas in response to elevated blood glucose levels and primarily acts on the liver, muscles, and adipose tissue to regulate blood glucose levels in a homeostatic manner. Insulin also reaches the hypothalamus, where it induces satiety and influences specific nuclei involved in the sympathetic pathways to the ovary. Importantly, insulin activates at least two excitatory inputs into the paraventricular nucleus (PVN). The first is α-melanocyte-stimulating hormone (α-MSH), which binds to melanocortin type 3 and 4 receptors (MC3/4R) (61). The second is glutamate, which binds to NMDA receptors (62). Additionally, insulin inhibits the primary inhibitory input to the PVN, which comes from neuropeptide Y (NPY) projections originating in the arcuate nucleus (ARC) (32). Both the action of leptin and insulin are even potentiated by another hormone, Angiotensin II, to increase the excitation of sympathetic fibers (63). Interestingly, despite insulin resistance and decreased transport of insulin across the blood-brain barrier, the brain becomes more sensitive to the sympathoexcitatory effects and pressure action of insulin in obesity, through an unknown mechanism (64). It is still unclear whether the insulin action on ARC-PVN-SNS pathway controls ovarian physiology. Future research should clarify whether brain insulin action influences ovarian physiology and contributes to ovarian pathology through the sympathetic nervous system.
5.2 Glucagon like peptide 1
Glucagon-like peptide 1 (GLP-1) is an incretin secreted from the gut after a meal. It increases insulin secretion from the pancreas and has wide effects on the body. Additionally, neurons that release GLP-1 are found in the nucleus of the solitary tract (NTS), ventrolateral medulla, and olfactory bulb (65, 66). These neurons project to the hypothalamus and other brain regions (67, 68). GLP-1 receptors are highly expressed in the PVN and arcuate nucleus (69, 70), both nuclei involved in controlling pre-autonomic neurons in the brain. Indeed, GLP-1agonists injections in the PVN lead to increase renal sympathetic activation and mean arterial pressure demonstrating an activation on sympathetic nerves (70). Alternatively, the action of GLP-1 on the olfactory bulb inhibits PVN neurons lead to decrease sympathetic tone in the pancreas (71). Regarding the ovary, several reports have proposed GLP-1 agonists to treat PCOS, which is still on research. Certainly, GLP-1 has demonstrated direct and indirect effects on the ovary. In example, GLP-1 administration to rats increases follicular atresia and alters the redox balance of the ovary (72). Also, GLP-1 or exendin-4, a GLP-1R agonist, modulates hypothalamus-pituitary gonad axis, modifying follicle development (73). On the other hand, GLP-1-based multi-agonists have demonstrated notable beneficial effects. Specifically, the GLP-1/Estrogen combination has shown superior efficacy compared to metformin and other multi-agonists in managing the metabolic complications of PCOS, while also enhancing ovarian cyclicity in an anovulatory PCOS model (74). Although hypothalamic GLP-1 regulates sympathetic innervation to various organs, the effect of GLP-1 on regulating sympathetic innervation of the ovary remains speculative and needs to be demonstrated.
6 Role of the PVN- SNS-ovary pathway in ovarian pathophysiology and treatment
It is well known that polycystic ovary syndrome (PCOS) in humans is associated with increased sympathetic nervous system activity and a heightened cardiovascular risk. In animal models of PCOS, which can be induced by early administration of androgens or estrogens, an increase in the activity of ovarian sympathetic nerves has been observed (75, 76). Notably, denervation of the sympathetic nerves to the ovaries can partially restore ovulation, improve estrous cyclicity, and enhance follicle development (77). Additionally, insulin resistance and leptin resistance, along with hyperleptinemia and hyperinsulinemia, are also present in animal models of PCOS (74, 78, 79). Consequently, elevated levels of leptin and compensatory hyperinsulinemia may lead to increased activity in the sympathetic nerves that innervate the ovaries. Moreover, interventions such as weight loss, regular physical exercise, metformin, and GLP-1 agonists have demonstrated favorable outcomes in enhancing follicle development, promoting ovulation, and alleviating the symptoms associated with PCOS (80–84). All of these treatments improve insulin sensitivity, leading to decreased insulin and leptin levels due to a reduction in body fat. However, it remains uncertain whether these improvements also reduce sympathetic activity in the ovaries or other organs. In summary, these insights emphasize the need for further research to determine whether the modulation of sympathetic nerve activity through lowered leptin or insulin levels plays a central role in the effects of these treatments.
7 Concluding remarks
In the complex relationship between metabolism and reproduction, leptin plays a crucial role in regulating how metabolism affects reproduction by controlling the endocrine HPG axis. Our proposal suggests that leptin also influences the sympathetic innervation of the ovary, leading to increased neural activity. This implies that in cases of obesity, leptin’s altered control of the SNS could significantly impact ovarian function and contribute to conditions like PCOS. Additionally, early exposure to obesity or overfeeding may raise leptin levels during early development, potentially changing the relationship between the hypothalamus and SNS. This could result in an increased susceptibility to chronic diseases in offspring due to overactivation of the SNS.
Author contributions
CA: Writing – original draft, Writing – review & editing. AP: Writing – review & editing. JE: Writing – review & editing, Funding acquisition. DF: Writing – review & editing. RB: Funding acquisition, Writing – original draft, Writing – review & editing. GC: Funding acquisition, Supervision, Writing – original draft, Writing – review & editing.
Funding
The author(s) declare financial support was received for the research, authorship, and/or publication of this article. Fondecyt 1201816 (GC), DICYT N° 022401BP (RB).
Acknowledgments
Writing and grammar suggestions were generated using Grammarly (Free Version, 2024), an AI-powered writing assistant developed by Grammarly Inc.
Conflict of interest
The authors declare that the research was conducted in the absence of any commercial or financial relationships that could be construed as a potential conflict of interest.
Publisher’s note
All claims expressed in this article are solely those of the authors and do not necessarily represent those of their affiliated organizations, or those of the publisher, the editors and the reviewers. Any product that may be evaluated in this article, or claim that may be made by its manufacturer, is not guaranteed or endorsed by the publisher.
References
1. Kezele P, Skinner MK. Regulation of ovarian primordial follicle assembly and development by estrogen and progesterone: endocrine model of follicle assembly. Endocrinology. (2003) 144:3329–37. doi: 10.1210/en.2002-0131
2. McGee EA, Hsueh AJ. Initial and cyclic recruitment of ovarian follicles. Endocr Rev. (2000) 21:200–14. doi: 10.1210/edrv.21.2.0394
3. Kinnear HM, Tomaszewski CE, Chang AL, Moravek MB, Xu M, Padmanabhan V, et al. The ovarian stroma as a new frontier. Reproduction. (2020) 160:R25–39. doi: 10.1530/REP-19-0501
4. Li L, Shi X, Shi Y, Wang Z. The signaling pathways involved in ovarian follicle development. Front Physiol. (2021) 12:730196. doi: 10.3389/fphys.2021.730196
5. Dorfman M, Arancibia S, Fiedler JL, Lara HE. Chronic intermittent cold stress activates ovarian sympathetic nerves and modifies ovarian follicular development in the rat. Biol Reprod. (2003) 68:2038–43. doi: 10.1095/biolreprod.102.008318
6. Thorp AA, Schlaich MP. Relevance of sympathetic nervous system activation in obesity and metabolic syndrome. J Diabetes Res. (2015) 2015:341583. doi: 10.1155/2015/341583
7. Mayerhofer A, Kunz LA. non-neuronal cholinergic system of the ovarian follicle. Ann Anat. (2005) 187:521–8. doi: 10.1016/j.aanat.2005.06.005
8. Pastelin CF, Rivera-Castro ME, Mirto-Aguilar N, Moran C. Structural organization of the neuronal pathways of the superior ovarian nerve in the rat. J Ovarian Res. (2023) 16:25. doi: 10.1186/s13048-023-01109-1
9. Norjavaara E, Rosberg S, Gafvels M, Boberg BM, Selstam G. Beta-adrenergic receptor concentration and subtype in the corpus luteum of the adult pseudopregnant rat. J Reprod Fertil. (1989) 86:567–75. doi: 10.1530/jrf.0.0860567
10. Ricu M, Paredes A, Greiner M, Ojeda SR, Lara HE. Functional development of the ovarian noradrenergic innervation. Endocrinology. (2008) 149:50–6. doi: 10.1210/en.2007-1204
11. Ratner A, Weiss GK, Sanborn CR. Stimulation by beta 2-adrenergic receptors of the production of cyclic AMP and progesterone in rat ovarian tissue. J Endocrinol. (1980) 87:123–9. doi: 10.1677/joe.0.0870123
12. Dyer CA, Erickson GF. Norepinephrine amplifies human chorionic gonadotropin-stimulated androgen biosynthesis by ovarian theca-interstitial cells. Endocrinology. (1985) 116:1645–52. doi: 10.1210/endo-116-4-1645
13. Mayerhofer A, Dissen GA, Costa ME, Ojeda SR. A role for neurotransmitters in early follicular development: induction of functional follicle-stimulating hormone receptors in newly formed follicles of the rat ovary. Endocrinology. (1997) 138:3320–9. doi: 10.1210/endo.138.8.5335
14. Lara HE, McDonald JK, Ahmed CE, Ojeda SR. Guanethidine-mediated destruction of ovarian sympathetic nerves disrupts ovarian development and function in rats. Endocrinology. (1990) 127:2199–209. doi: 10.1210/endo-127-5-2199
15. Forneris ML, Aguado LI. Neonatal superior ovarian nerve transection disturbs the cyclic activity of the female rats. J Steroid Biochem Mol Biol. (2002) 82:75–82. doi: 10.1016/s0960-0760(02)00149-8
16. Gerendai I, Toth IE, Boldogkoi Z, Medveczky I, Halasz B. Neuronal labeling in the rat brain and spinal cord from the ovary using viral transneuronal tracing technique. Neuroendocrinology. (1998) 68:244–56. doi: 10.1159/000054372
17. Grzeda E, Ziarniak K, Sliwowska JH. The paraventricular nucleus of the hypothalamus - the concertmaster of autonomic control. Focus on blood pressure regulation. Acta Neurobiol Exp (Wars). (2023) 83:34–44. doi: 10.55782/ane-2023-004
18. Ferguson AV, Latchford KJ, Samson WK. The paraventricular nucleus of the hypothalamus - a potential target for integrative treatment of autonomic dysfunction. Expert Opin Ther Targets. (2008) 12:717–27. doi: 10.1517/14728222.12.6.717
19. Ceccatelli S, Cintra A, Hokfelt T, Fuxe K, Wikstrom AC, Gustafsson JA. Coexistence of glucocorticoid receptor-like immunoreactivity with neuropeptides in the hypothalamic paraventricular nucleus. Exp Brain Res. (1989) 78:33–42. doi: 10.1007/BF00230684
20. Grassi D, Marraudino M, Garcia-Segura LM, Panzica GC. The hypothalamic paraventricular nucleus as a central hub for the estrogenic modulation of neuroendocrine function and behavior. Front Neuroendocrinol. (2022) 65:100974. doi: 10.1016/j.yfrne.2021.100974
21. Dampney RA. Functional organization of central pathways regulating the cardiovascular system. Physiol Rev. (1994) 74:323–64. doi: 10.1152/physrev.1994.74.2.323
22. Badoer E. Hypothalamic paraventricular nucleus and cardiovascular regulation. Clin Exp Pharmacol Physiol. (2001) 28:95–9. doi: 10.1046/j.1440-1681.2001.03413.x
23. Kawabe T, Chitravanshi VC, Kawabe K, Sapru HN. Cardiovascular function of a glutamatergic projection from the hypothalamic paraventricular nucleus to the nucleus tractus solitarius in the rat. Neuroscience. (2008) 153:605–17. doi: 10.1016/j.neuroscience.2008.02.076
24. Kawabe T, Chitravanshi VC, Nakamura T, Kawabe K, Sapru HN. Mechanism of heart rate responses elicited by chemical stimulation of the hypothalamic paraventricular nucleus in the rat. Brain Res. (2009) 1248:115–26. doi: 10.1016/j.brainres.2008.10.059
25. Nunn N, Womack M, Dart C, Barrett-Jolley R. Function and pharmacology of spinally-projecting sympathetic pre-autonomic neurones in the paraventricular nucleus of the hypothalamus. Curr Neuropharmacol. (2011) 9:262–77. doi: 10.2174/157015911795596531
26. Jara P, Rage F, Dorfman M, Grouselle D, Barra R, Arancibia S, et al. Cold-induced glutamate release in vivo from the magnocellular region of the paraventricular nucleus is involved in ovarian sympathetic activation. J Neuroendocrinol. (2010) 22:979–86. doi: 10.1111/j.1365-2826.2010.02040.x
27. Toth IE, Banczerowski P, Boldogkoi Z, Toth JS, Szabo A, Halasz B, et al. Cerebral neurons involved in the innervation of both the adrenal gland and the ovary: a double viral tracing study. Brain Res Bull. (2008) 77:306–11. doi: 10.1016/j.brainresbull.2008.08.022
28. Gerendai I, Kocsis K, Halasz B. Supraspinal connections of the ovary: structural and functional aspects. Microsc Res Tech. (2002) 59:474–83. doi: 10.1002/jemt.10225
29. Fiedler J, Jara P, Luza S, Dorfman M, Grouselle D, Rage F, et al. Cold stress induces metabolic activation of thyrotrophin-releasing hormone-synthesising neurones in the magnocellular division of the hypothalamic paraventricular nucleus and concomitantly changes ovarian sympathetic activity parameters. J Neuroendocrinol. (2006) 18:367–76. doi: 10.1111/j.1365-2826.2006.01427.x
30. Heras V, Castellano JM, Fernandois D, Velasco I, Rodriguez-Vazquez E, Roa J, et al. Central ceramide signaling mediates obesity-induced precocious puberty. Cell Metab. (2020) 32:951–966.e958. doi: 10.1016/j.cmet.2020.10.001
31. Morton GJ, Cummings DE, Baskin DG, Barsh GS, Schwartz MW. Central nervous system control of food intake and body weight. Nature. (2006) 443:289–95. doi: 10.1038/nature05026
32. Cassaglia PA, Shi Z, Brooks VL. Insulin increases sympathetic nerve activity in part by suppression of tonic inhibitory neuropeptide Y inputs into the paraventricular nucleus in female rats. Am J Physiol Regul Integr Comp Physiol. (2016) 311:R97–R103. doi: 10.1152/ajpregu.00054.2016
33. Balthasar N, Dalgaard LT, Lee CE, Yu J, Funahashi H, Williams T, et al. Divergence of melanocortin pathways in the control of food intake and energy expenditure. Cell. (2005) 123:493–505. doi: 10.1016/j.cell.2005.08.035
34. Shi Z, Bonillas AC, Wong J, Padilla SL, Brooks VL. Neuropeptide Y suppresses thermogenic and cardiovascular sympathetic nerve activity via Y1 receptors in the paraventricular nucleus and dorsomedial hypothalamus. J Neuroendocrinol. (2021) 33:e13006. doi: 10.1111/jne.13006
35. Shi Z, Madden CJ, Brooks VL. Arcuate neuropeptide Y inhibits sympathetic nerve activity via multiple neuropathways. J Clin Invest. (2017) 127:2868–80. doi: 10.1172/JCI92008
36. Wada N, Hirako S, Takenoya F, Kageyama H, Okabe M, Shioda S. Leptin and its receptors. J Chem Neuroanat. (2014) 61-62:191–9. doi: 10.1016/j.jchemneu.2014.09.002
37. Balland E, Dam J, Langlet F, Caron E, Steculorum S, Messina A, et al. Hypothalamic tanycytes are an ERK-gated conduit for leptin into the brain. Cell Metab. (2014) 19:293–301. doi: 10.1016/j.cmet.2013.12.015
38. Di Spiezio A, Sandin ES, Dore R, Muller-Fielitz H, Storck SE, Bernau M, et al. The LepR-mediated leptin transport across brain barriers controls food reward. Mol Metab. (2018) 8:13–22. doi: 10.1016/j.molmet.2017.12.001
39. Bray GA. Reciprocal relation of food intake and sympathetic activity: experimental observations and clinical implications. Int J Obes Relat Metab Disord. (2000) 24 Suppl 2:S8–17. doi: 10.1038/sj.ijo.0801269
40. Matsumura K, Tsuchihashi T, Fujii K, Iida M. Neural regulation of blood pressure by leptin and the related peptides. Regul Pept. (2003) 114:79–86. doi: 10.1016/s0167-0115(03)00116-2
41. Rezai-Zadeh K, Munzberg H. Integration of sensory information via central thermoregulatory leptin targets. Physiol Behav. (2013) 121:49–55. doi: 10.1016/j.physbeh.2013.02.014
42. Zeng W, Pirzgalska RM, Pereira MM, Kubasova N, Barateiro A, Seixas E, et al. Sympathetic neuro-adipose connections mediate leptin-driven lipolysis. Cell. (2015) 163:84–94. doi: 10.1016/j.cell.2015.08.055
43. Scarpace PJ, Matheny M. Leptin induction of UCP1 gene expression is dependent on sympathetic innervation. Am J Physiol. (1998) 275:E259–264. doi: 10.1152/ajpendo.1998.275.2.E259
44. Dunbar JC, Hu Y, Lu H. Intracerebroventricular leptin increases lumbar and renal sympathetic nerve activity and blood pressure in normal rats. Diabetes. (1997) 46:2040–3. doi: 10.2337/diab.46.12.2040
45. Fernandois D, Vazquez MJ, Barroso A, Paredes AH, Tena-Sempere M, Cruz G. Multi-organ increase in norepinephrine levels after central leptin administration and diet-induced obesity. Int J Mol Sci. (2023) 24:16909. doi: 10.3390/ijms242316909
46. Tanida M, Yamamoto N, Morgan DA, Kurata Y, Shibamoto T, Rahmouni K. Leptin receptor signaling in the hypothalamus regulates hepatic autonomic nerve activity via phosphatidylinositol 3-kinase and AMP-activated protein kinase. J Neurosci. (2015) 35:474–84. doi: 10.1523/JNEUROSCI.1828-14.2015
47. Rahmouni K, Sigmund CD, Haynes WG, Mark AL. Hypothalamic ERK mediates the anorectic and thermogenic sympathetic effects of leptin. Diabetes. (2009) 58:536–42. doi: 10.2337/db08-0822
48. Miyamoto L, Ebihara K, Kusakabe T, Aotani D, Yamamoto-Kataoka S, Sakai T, et al. Leptin activates hepatic 5’-AMP-activated protein kinase through sympathetic nervous system and alpha1-adrenergic receptor: a potential mechanism for improvement of fatty liver in lipodystrophy by leptin. J Biol Chem. (2012) 287:40441–7. doi: 10.1074/jbc.M112.384545
49. Mark AL, Agassandian K, Morgan DA, Liu X, Cassell MD, Rahmouni K. Leptin signaling in the nucleus tractus solitarii increases sympathetic nerve activity to the kidney. Hypertension. (2009) 53:375–80. doi: 10.1161/HYPERTENSIONAHA.108.124255
50. Lechan RM, Fekete C. The TRH neuron: a hypothalamic integrator of energy metabolism. Prog Brain Res. (2006) 153:209–35. doi: 10.1016/S0079-6123(06)53012-2
51. Shih CD, Au LC, Chan JY. Differential role of leptin receptors at the hypothalamic paraventricular nucleus in tonic regulation of food intake and cardiovascular functions. J BioMed Sci. (2003) 10:367–78. doi: 10.1159/000071156
52. Alvarez D, Ceballo K, Olguin S, Martinez-Pinto J, Maliqueo M, Fernandois D, et al. Prenatal metformin treatment improves ovarian function in offspring of obese rats. J Endocrinol. (2018) 239:325–38. doi: 10.1530/JOE-18-0352
53. Tamashiro KL, Terrillion CE, Hyun J, Koenig JI, Moran TH. Prenatal stress or high-fat diet increases susceptibility to diet-induced obesity in rat offspring. Diabetes. (2009) 58:1116–25. doi: 10.2337/db08-1129
54. Mistry AM, Swick A, Romsos DR. Leptin alters metabolic rates before acquisition of its anorectic effect in developing neonatal mice. Am J Physiol. (1999) 277:R742–747. doi: 10.1152/ajpregu.1999.277.3.R742
55. Bouret SG. Leptin, nutrition, and the programming of hypothalamic feeding circuits. Nestle Nutr Workshop Ser Pediatr Program. (2010) 65:25–35. doi: 10.1159/000281143
56. Bouret SG, Draper SJ, Simerly RB. Trophic action of leptin on hypothalamic neurons that regulate feeding. Science. (2004) 304:108–10. doi: 10.1126/science.1095004
57. Picut CA, Dixon D, Simons ML, Stump DG, Parker GA, Remick AK. Postnatal ovary development in the rat: morphologic study and correlation of morphology to neuroendocrine parameters. Toxicol Pathol. (2015) 43:343–53. doi: 10.1177/0192623314544380
58. Ambrosetti V, Guerra M, Ramirez LA, Reyes A, Alvarez D, Olguin S, et al. Increase in endogenous estradiol in the progeny of obese rats is associated with precocious puberty and altered follicular development in adulthood. Endocrine. (2016) 53:258–70. doi: 10.1007/s12020-016-0858-0
59. Samuelsson AM, Morris A, Igosheva N, Kirk SL, Pombo JM, Coen CW, et al. Evidence for sympathetic origins of hypertension in juvenile offspring of obese rats. Hypertension. (2010) 55:76–82. doi: 10.1161/HYPERTENSIONAHA.109.139402
60. Oben JA, Mouralidarane A, Samuelsson AM, Matthews PJ, Morgan ML, McKee C, et al. Maternal obesity during pregnancy and lactation programs the development of offspring non-alcoholic fatty liver disease in mice. J Hepatol. (2010) 52:913–20. doi: 10.1016/j.jhep.2009.12.042
61. Ward KR, Bardgett JF, Wolfgang L, Stocker SD. Sympathetic response to insulin is mediated by melanocortin 3/4 receptors in the hypothalamic paraventricular nucleus. Hypertension. (2011) 57:435–41. doi: 10.1161/HYPERTENSIONAHA.110.160671
62. Stocker SD, Gordon KW. Glutamate receptors in the hypothalamic paraventricular nucleus contribute to insulin-induced sympathoexcitation. J Neurophysiol. (2015) 113:1302–9. doi: 10.1152/jn.00764.2014
63. Shi Z, Stornetta RL, Stornetta DS, Abbott SBG, Brooks VL. The arcuate nucleus: A site of synergism between Angiotensin II and leptin to increase sympathetic nerve activity and blood pressure in rats. Neurosci Lett. (2022) 785:136773. doi: 10.1016/j.neulet.2022.136773
64. Shi Z, Zhao D, Cassaglia PA, Brooks VL. Sites and sources of sympathoexcitation in obese male rats: role of brain insulin. Am J Physiol Regul Integr Comp Physiol. (2020) 318:R634–48. doi: 10.1152/ajpregu.00317.2019
65. Han VK, Hynes MA, Jin C, Towle AC, Lauder JM, Lund PK. Cellular localization of proglucagon/glucagon-like peptide I messenger RNAs in rat brain. J Neurosci Res. (1986) 16:97–107. doi: 10.1002/jnr.490160110
66. Jin SL, Han VK, Simmons JG, Towle AC, Lauder JM, Lund PK. Distribution of glucagonlike peptide I (GLP-I), glucagon, and glicentin in the rat brain: an immunocytochemical study. J Comp Neurol. (1988) 271:519–32. doi: 10.1002/cne.902710405
67. Tauchi M, Zhang R, D’Alessio DA, Stern JE, Herman JP. Distribution of glucagon-like peptide-1 immunoreactivity in the hypothalamic paraventricular and supraoptic nuclei. J Chem Neuroanat. (2008) 36:144–9. doi: 10.1016/j.jchemneu.2008.07.009
68. Larsen PJ, Tang-Christensen M, Holst JJ, Orskov C. Distribution of glucagon-like peptide-1 and other preproglucagon-derived peptides in the rat hypothalamus and brainstem. Neuroscience. (1997) 77:257–70. doi: 10.1016/s0306-4522(96)00434-4
69. Merchenthaler I, Lane M, Shughrue P. Distribution of pre-pro-glucagon and glucagon-like peptide-1 receptor messenger RNAs in the rat central nervous system. J Comp Neurol. (1999) 403:261–80. doi: 10.1002/(sici)1096-9861(19990111)403:2<261::aid-cne8>3.0.co;2-5
70. Xu XY, Wang JX, Chen JL, Dai M, Wang YM, Chen Q, et al. GLP-1 in the hypothalamic paraventricular nucleus promotes sympathetic activation and hypertension. J Neurosci. (2024) 44. doi: 10.1523/JNEUROSCI.2032-23.2024
71. Montaner M, Denom J, Simon V, Jiang W, Holt MK, Brierley DI, et al. A neuronal circuit driven by GLP-1 in the olfactory bulb regulates insulin secretion. Nat Commun. (2024) 15:6941. doi: 10.1038/s41467-024-51076-4
72. Saber SM, Abd El-Rahman HA. Liraglutide treatment effects on rat ovarian and uterine tissues. Reprod Biol. (2019) 19:237–44. doi: 10.1016/j.repbio.2019.07.003
73. Outeirino-Iglesias V, Romani-Perez M, Gonzalez-Matias LC, Vigo E, Mallo F. GLP-1 increases preovulatory LH source and the number of mature follicles, as well as synchronizing the onset of puberty in female rats. Endocrinology. (2015) 156:4226–37. doi: 10.1210/en.2014-1978
74. Sanchez-Garrido MA, Serrano-Lopez V, Ruiz-Pino F, Vazquez MJ, Rodriguez-Martin A, Torres E, et al. Superior metabolic improvement of polycystic ovary syndrome traits after GLP1-based multi-agonist therapy. Nat Commun. (2024) 15:8498. doi: 10.1038/s41467-024-52898-y
75. Lara HE, Ferruz JL, Luza S, Bustamante DA, Borges Y, Ojeda SR. Activation of ovarian sympathetic nerves in polycystic ovary syndrome. Endocrinology. (1993) 133:2690–5. doi: 10.1210/endo.133.6.7902268
76. Anesetti G, Chavez-Genaro R. Neonatal testosterone exposure induces early development of follicular cysts followed by sympathetic ovarian hyperinnervation. Reprod Fertil Dev. (2015) 28:1753–61. doi: 10.1071/RD14460
77. Del Campo M, Piquer B, Witherington J, Sridhar A, Lara HE. Effect of superior ovarian nerve and plexus nerve sympathetic denervation on ovarian-derived infertility provoked by estradiol exposure to rats. Front Physiol. (2019) 10:349. doi: 10.3389/fphys.2019.00349
78. Manneras L, Cajander S, Holmang A, Seleskovic Z, Lystig T, Lonn M, et al. A new rat model exhibiting both ovarian and metabolic characteristics of polycystic ovary syndrome. Endocrinology. (2007) 148:3781–91. doi: 10.1210/en.2007-0168
79. Fang YQ, Ding H, Li T, Zhao XJ, Luo D, Liu Y, et al. N-acetylcysteine supplementation improves endocrine-metabolism profiles and ovulation induction efficacy in polycystic ovary syndrome. J Ovarian Res. (2024) 17:205. doi: 10.1186/s13048-024-01528-8
80. Scragg J, Hobson A, Willis L, Taylor KS, Dixon S, Jebb SA. Effect of weight loss interventions on the symptomatic burden and biomarkers of polycystic ovary syndrome: A systematic review of randomized controlled trials. Ann Intern Med. (2024) 177:1664–74. doi: 10.7326/M23-3179
81. Jensterle M, Kravos NA, Ferjan S, Goricar K, Dolzan V, Janez A. Long-term efficacy of metformin in overweight-obese PCOS: longitudinal follow-up of retrospective cohort. Endocr Connect. (2020) 9:44–54. doi: 10.1530/EC-19-0449
82. Bernal JVM, da Veiga AC, Philbois SV, Ribeiro VB, Aguilar BA, Paixao TEV, et al. Women with polycystic ovary syndrome and excess body fat exhibit atypical sympathetic autonomic modulation that is partially reversed by aerobic physical training. Clin Endocrinol (Oxf). (2024). doi: 10.1111/cen.v102.2
83. Furat Rencber S, Kurnaz Ozbek S, Eraldemir C, Sezer Z, Kum T, Ceylan S, et al. Effect of resveratrol and metformin on ovarian reserve and ultrastructure in PCOS: an experimental study. J Ovarian Res. (2018) 11:55. doi: 10.1186/s13048-018-0427-7
84. Austregesilo de Athayde De Hollanda Morais B, Martins Prizao V, de Moura de Souza M, Ximenes Mendes B, Rodrigues Defante ML, Cosendey Martins O, et al. The efficacy and safety of GLP-1 agonists in PCOS women living with obesity in promoting weight loss and hormonal regulation: A meta-analysis of randomized controlled trials. J Diabetes Complications. (2024) 38:108834. doi: 10.1016/j.jdiacomp.2024.108834
Keywords: sympathetic, ovary, leptin, hypothalamus, metabolic
Citation: Astudillo-Guerrero C, Paredes AH, Escobar J, Fernandois D, Barra R and Cruz G (2025) Metabolic control of ovarian function through the sympathetic nervous system: role of leptin. Front. Endocrinol. 15:1484939. doi: 10.3389/fendo.2024.1484939
Received: 22 August 2024; Accepted: 26 December 2024;
Published: 03 February 2025.
Edited by:
Sheba MohanKumar, University of Georgia, United StatesReviewed by:
David Garcia-Galiano, University of Cordoba, SpainCopyright © 2025 Astudillo-Guerrero, Paredes, Escobar, Fernandois, Barra and Cruz. This is an open-access article distributed under the terms of the Creative Commons Attribution License (CC BY). The use, distribution or reproduction in other forums is permitted, provided the original author(s) and the copyright owner(s) are credited and that the original publication in this journal is cited, in accordance with accepted academic practice. No use, distribution or reproduction is permitted which does not comply with these terms.
*Correspondence: Gonzalo Cruz, Z29uemFsby5jcnV6QHV2LmNs; Rafael Barra, cmFmYWVsLmJhcnJhQHVzYWNoLmNs