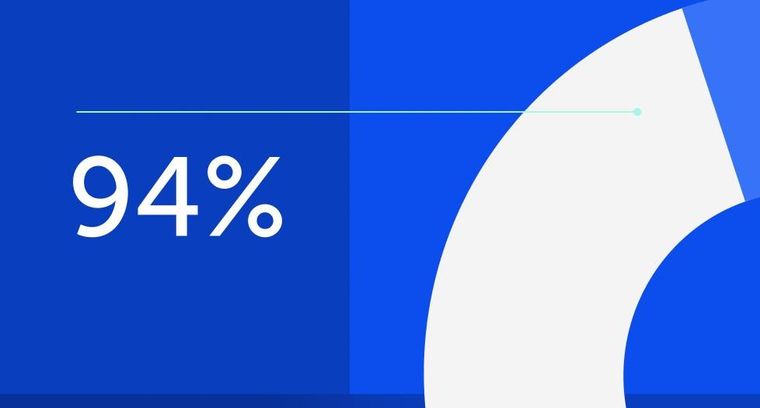
94% of researchers rate our articles as excellent or good
Learn more about the work of our research integrity team to safeguard the quality of each article we publish.
Find out more
REVIEW article
Front. Endocrinol., 22 January 2025
Sec. Reproduction
Volume 15 - 2024 | https://doi.org/10.3389/fendo.2024.1464561
This article is part of the Research TopicInfertility and EndometriosisView all 24 articles
Polycystic ovary syndrome (PCOS) is a prevalent disorder of the endocrine system with significant clinical implications, often leading to health complications related to adipose tissue accumulation, including obesity, insulin resistance (IR), metabolic syndrome, and type 2 diabetes mellitus. While the precise pathogenesis of PCOS remains unclear, it is now recognized that genetic, endocrine, and metabolic dysregulations all contribute significantly to its onset. The immunopathogenesis of PCOS has not been extensively explored, but there is growing speculation that immune system abnormalities may play a pivotal role. This chronic inflammatory state is exacerbated by factors such as obesity and hyperinsulinemia. Therefore, this review aims to elucidate the interplay between IR in PCOS patients, the controlled immune response orchestrated by immune cells and immunomodulatory molecules, and their interactions with adipocytes, hyperandrogenemia, chronic inflammation, and metabolic homeostasis.
Polycystic ovary syndrome (PCOS) is the most prevalent endocrine disorder in women, characterized by anovulatory subfertility. It affects approximately 5.6% of Chinese women of reproductive age and around 10% of women from other ethnic backgrounds (1, 2). Clinical features of PCOS include irregular menstruation, infertility, hyperandrogenemia, ovarian polycystic changes, and metabolic abnormalities such as obesity, insulin resistance (IR), and dyslipidemia (Figure 1). PCOS is recognized as a significant risk factor for T2DM (T2DM), cardiovascular disease, gestational diabetes, and gestational hypertension.
Figure 1. The symptoms of PCOS: PCOS is a chronic endocrine, metabolic and reproductive disorder with multiple signs and symptoms. Its main characteristics are ovulation dysfunction (manifested by irregular menstruation, such as oligomenorrhea and amenorrhea), hyperandrogen (manifested by hypertrichosis and acne), polycystic ovary morphology, metabolic disorders (obesity and insulin resistance) and infertility.
The etiology of PCOS remains elusive, and its pathogenesis is complex. According to the latest diagnostic criteria, PCOS can be diagnosed by meeting any two of the following criteria: clinical/biochemical hyperandrogenemia, ovulation disorders, and ultrasound evidence of polycystic ovarian manifestations/abnormal Anti-Mullerian Hormone (AMH) levels (1, 3). Heterogeneity in the diagnostic modalities of IR is evident, with studies demonstrating that fasting triglycerides can be used selectively in place of fasting glucose for the assessment of IR, and that the HOMA-IR index, glucose, and serum insulin levels do not provide a fully consistent measure of IR severity (4, 5). While IR is not a diagnostic criterion for PCOS, the oral glucose tolerance test (OGTT) has been utilized as a primary screening tool for PCOS patients. In recent years, two distinct subtypes of PCOS with varying biochemical profiles have been internationally defined (6). The reproductive subtype is characterized by elevated serum luteinizing hormone (LH) and sex hormone-binding globulin (SHBG) levels, typically with a normal body mass index (BMI) and insulin levels. On the other hand, the metabolic subtype is associated with high BMI and insulin levels, along with relatively low LH and SHBG levels.
There is a growing body of evidence suggesting that immunity plays a significant role in the manifestation of symptoms in PCOS, particularly concerning insulin resistance, adipocyte dysfunction, glucose metabolism, and chronic inflammation. The chronic low-grade inflammation observed in PCOS patients is primarily linked to the accumulation of visceral adipose tissue, where adipocytes undergo necrosis due to hypoxia, leading to the infiltration of inflammatory cells that secrete various inflammatory cytokines. Immune cells have the capacity to either trigger or suppress inflammation by releasing pro-inflammatory or anti-inflammatory cytokines. Immune molecules such as antibodies complement proteins, and lymphokines are generated by immune cells in response to antigenic stimulation. Dysregulated immune cell function or imbalances in immune-related factors can result in immune dysfunction (7, 8). Due to impaired ovulation in patients with PCOS, IR causes androgen overproduction in patients with low progesterone levels, and the two inflammatory phenotypes reinforce each other. the IR-induced abnormalities persist and are exacerbated by androgenic and metabolic disorders, which induce severe immune dysregulation, resulting in the development of systemic symptoms in the patients (9) (Figure 2).
Figure 2. Factors affecting PCOS, phenotypes and mechanisms of interactions between long-term effects: mechanisms of interactions between IR, hyperandrogenemia and chronic inflammation in PCOS, and the relationship with long-term effects. Environmental and genetic factors may contribute to obesity, and the combination of obesity and these two factors predispose to IR and hyperandrogenemia.The interaction between IR and hyperandrogenemia may create a favorable environment for chronic inflammation, and the whole process is involved by the immune system. Ultimately, the interplay among chronic inflammation, immune system, IR, and hyperandrogenemia may result in infertility, metabolic disorders, and adverse pregnancy outcomes in patients with PCOS.
Moreover, several immune molecules are directly implicated in the immune response and are closely associated with insulin resistance in PCOS. This review provides a brief overview of current research on various innate immune cells and immune molecules, elucidating their roles and mechanisms in the development of insulin resistance in PCOS.
Macrophages play a crucial role in the immune response of patients with PCOS who develop IR. They serve as primary actors in innate immunity and also function as antigen-presenting cells in specific immunity. While not all patients with an IR phenotype show signs of obesity, it is evident that obesity significantly increases the risk of metabolic syndrome in individuals with PCOS (10). In obese PCOS patients with decreased levels of lipocalin (11), there is a substantial increase in both the size and quantity of adipocytes within a short timeframe. This rapid growth leads to local tissue ischemia and hypoxia, triggering the accumulation of macrophages, which can expand to more than five times their original size (12–14). Activated and differentiated M1 macrophages are predominantly located in a ring-like structure surrounding dead adipocytes, known as the crown-like structure (CLS), where they phagocytose these cells and sustain the release of pro-inflammatory cytokines (15).
Clinical studies have shown increased expression and phosphorylation levels of Forkhead box O1 (FOXO1) in peripheral blood macrophages from patients with PCOS (16). Insulin signaling in macrophages leads to the inactivation and nuclear exclusion of FOXO1 during its phosphorylation, a process that can be reversed under conditions of IR or inflammation (17). Silencing FOXO1 in macrophages results in monocytes exhibiting a greater polarization towards the M2-type, which helps to reduce the inflammatory state and IR (18, 19). On the other hand, upregulating FOXO1 promotes the release of Toll-like receptor 4 (TLR4)-mediated pro-inflammatory factors such as IL-1β, IL-6, and TNF-α (16).
TNF-α is recognized as the initial chronic inflammatory cytokine discovered in adipose tissue linked to obesity. TNF-α has the ability to diminish insulin expression by reducing the levels of insulin receptor substrate 1 (IRS-1) and glucose transporter 4 (GLUT4) proteins. Additionally, TNF-α can trigger inflammatory pathways like nuclear factor kappa-B (NF-κB) and c-Jun N-terminal kinase (JNK) in adipocytes. This activation hampers insulin sensitivity further under the influence of inflammatory factors, thereby worsening IR (20–22).
Obesity, inflammation, and IR show a mutually reinforcing relationship, i.e., increased accumulation of adipocytes promotes macrophage aggregation and differentiation, thereby inducing inflammation and stimulating IR.
In lean patients with PCOS, abnormalities in adipose tissue persist (11). In the subcutaneous adipose tissue of PCOS patients, there were higher levels of CD11c-positive adipose tissue macrophages, as well as elevated levels of TNF-α and leptin compared to body mass index (BMI)-matched women without PCOS. Additionally, in visceral adipose tissue, catecholamines exhibited significantly increased lipolytic effects, leading to the release of fatty acids from visceral adipose tissue, which were closely linked to insulin resistance. On the other hand, some studies have indicated that there is no significant difference in the transport and mechanism of action of fatty acids and monoacylglycerol in PCOS patients, regardless of whether adipocytes are normal or abnormal in size (23, 24).
Presently, the majority of studies concentrate on investigating the mechanism of IR in obese patients with PCOS. However, the cause of IR in lean PCOS patients might be associated with the pro-inflammatory differentiation of macrophages triggered by abnormal fatty acid lipolysis in visceral fat. It is noteworthy that the locations of macrophage aggregation in the two categories of PCOS patients are not identical (25). In patients with polycystic ovary syndrome (PCOS), there are elevated levels of androgens and serum homocysteine. In mice with PCOS induced by dehydroepiandrosterone (DHEA), homocysteine leads to an imbalance of M1/M2-type macrophages in adipose tissue, causing a shift from M2-type to M1-type macrophages. This transition exacerbates DHEA-induced IR. Furthermore, the abnormally elevated insulin levels at this stage can stimulate androgen secretion from ovarian membranous cells, reduce insulin receptor autophosphorylation in ovarian granulosa cells, and further worsen the chronic inflammatory response and IR in the ovaries (26, 27).
T cells are a type of immune cell that matures in the thymus and plays a crucial role in specific immunity within an organism. They exhibit diverse subtypes and functions. CD4+ T cells contribute to maintaining the body’s immune response by recognizing major histocompatibility complex (MHC)-II-like molecules on the surface of antigen-presenting cells, subsequently activating and guiding other immune cells to the infection site. In the follicular fluid of patients with PCOS, the proportion of CD8+ T cells was notably lower compared to CD4+ T cells (28). CD4+ T cells play a more significant role in various clinical manifestations in PCOS patients, including IR. Based on their complex metabolic programming and cytokine production, CD4+ T cells can differentiate into distinct functional subpopulations. Among these, Th1 [producing interferon-gamma (IFN-γ)], Th17 (producing IL-17, IL-21, and IL-22), and Th2 (producing IL-4, IL-5, and IL-13) effector cells primarily rely on aerobic glycolysis for energy generation. In contrast, regulatory T cells (Treg) [producing IL-10, transforming growth factor β (TGF-β)] depend on oxidative phosphorylation driven by fatty acid oxidation (29–31).
In peripheral blood and adipose tissue of PCOS patients with metabolic abnormalities, T cells are the second largest immune cell population after macrophages, with significantly increased proportions of Th1, Th17, and CD8+ T cells and decreased proportions of Th2 and Treg cells (32–34). Metabolites of adipocytes can influence T-cell differentiation mediated by the T cell antigen receptor (TCR), and of the many soluble differentiation stimulators, fatty acids have been shown to be the strongest stimulators of Th1 differentiation (35, 36). T cells differentiated into pro-inflammatory Th1 cells produce IFN-γ, which induces macrophage differentiation towards the M1 phenotype. The aggregation of Th1 cells and infiltration of IFN-γ will recruit other immune cells, including macrophages, and increase the body’s inflammatory immune response (37). The above responses will be more pronounced in obese individuals. It was found that, in addition to its effects on immune cells, IFN-γ can directly induce IR in mature human adipocytes by taking charge of the activation of the janus kinase (JAK)/signal transducer and activator of transcription 1 (STAT1) pathway and inhibiting the expression of insulin signaling genes (GLUT4 and IRS1), lipid-forming genes (Perilipin, Lipoprotein Lipase, and Fatty Acid Synthesis Enzymes), and genes related to lipid storage (Recombinant Peroxisome Proliferator Activated Receptor(PPAG) and Lipocalin) (38). Insulin sensitivity was improved when the interferon gene was knocked out (39).
In the immunoregulation of T cells, the co-inhibitory receptors cytotoxic T-lymphocyte-associated protein 4(CTLA-4) and programmed cell death protein 1(PD-1) are co-expressed on effector T cells and participate in the dynamic balance of the immune response. The expression of PD-1 is maintained in T cells, which participates in the routine immune response of the body. In the acute phase, the expression of PD-1 decreases rapidly, and the body produces a rapid immune response. The proportion of T cells with elevated PD-1 expression in serum and follicular fluid was significantly higher in patients with PCOS than in normal patients, then metabolic disorders in patients with PCOS are associated with PD-1 expression (40). PD-1-overexpressing T cells are one of the subpopulations of the T-cell depletion phenotype (41, 42), and when in the presence of a PD-L1/PD-1 pathway blocking antibody, dendritic cells trigger the proliferation of syngeneic T cells and modulate T-cell subsets, which have elevated levels of IL-2 (43). This may suggest that in PCOS patients with abnormal T cells, PD-1 always plays a role in the relevant pathway and that high expression of PD-1 may inhibit T cell differentiation to Th2 type cells that secrete anti-inflammatory cytokines. In contrast, insulin has an anti-inflammatory effect on circulating immune cells, which may induce T cells to differentiate into an anti-inflammatory Th2 phenotype, and the dysregulation of the Th1/Th2 ratio and the PD-1-associated pathway may play a key role in resisting the effect of insulin on the immune response associated with T cells (44, 45).
CD4+ CD25+ Foxp3+ Treg cells were significantly lower in the peripheral blood of PCOS patients than in controls (34). FOXP3+ Treg cells can stimulate the production of IL-10 by macrophages through the secretion of IL-13, mediated by transforming growth factor-β. TGF-β and IL-10 act as the main anti-inflammatory cytokines in metabolism (46, 47). Smad4 and Recombinant Runt Related Transcription Factor 2 (RUNX2) genes are part of the TGF-β signaling pathway. The expression of these genes and related mRNAs increases during the development of hyperinsulinemia and IR in organisms (48). PCOS patients also differ from some obese patients in terms of hormone secretion levels. Estrogen helps limit inflammation, and in PCOS patients with hyperandrogenemia, the expression of the transcription factor B lymphocyte-induced maturation protein 1 (BLIMP1), which is androgen-dependent, is elevated. This leads to an immune response that brings about a new balance in the interaction between increased adipocyte inflammation and male-specific IL-33-producing stromal cells that actively recruit and locally expand Treg cell populations in a BLIMP1-dependent manner under the influence of sex hormones (49). In PCOS patients, there was a notable increase in Th17 cells, which predominantly secrete IL-17 to trigger neutrophilic inflammation, despite an overall decrease in Treg cells in the serum (34, 50). This indicates that the imbalance between Th17 and Treg cells in the context of abnormal insulin metabolism in PCOS patients is primarily due to the reduced levels of Treg cells.
Similar to T-cells, B-cells are classified into different subpopulations based on their distinct phenotypes, functions, and the primary cytokines they secrete. Presently, there are two primary categories: B-1 and B-2 cells. B-1 cells are predominantly found in organ cavities, mucosal tissues, and adipose tissues. They can be further categorized into CD5+B-1a and CD5-B-1b based on their CD5 expression (51, 52). B-1a cells, as innate B-like cells, are the primary producers of natural IgM antibodies in the body (53), and mainly function in the absence of antigens. In contrast, B-1b cells participate in T-cell-mediated immune responses to external antigens. B-2 cells are B cells traditionally derived from the bone marrow and migrate to lymphoid organs (54). They generate and concentrate specific antibodies, playing a crucial role in humoral immunity, and have the ability to differentiate into memory B cells and plasma cells. When B cells secrete anti-inflammatory factors like IL-10, and IL-35, they are collectively known as regulatory B cells (Bregs). These cells are not distinct subtypes from B-1 and B-2 cells but rather functional subtypes within these two classes of B cells.
In the context of IR driven by inflammatory adipose tissue, B-cells are the initial immune cells to accumulate, followed closely by T-cells, and eventually by macrophages (55). In individuals with PCOS experiencing low-grade chronic inflammation, the levels of anti-inflammatory IL-10 produced by Bregs were significantly lower compared to controls (56–58). Conversely, enhancing IL-10 expression or administering it for short durations in IR mice led to a notable increase in systemic insulin sensitivity. This effect was lost in the absence of IL-10 (59–61), indicating its role in inhibiting the differentiation of T cells into pro-inflammatory Th1 cells.
In patients with PCOS, the levels of IL-10 produced by B-1 cells decrease, leading to an increase in T-cell differentiation towards a pro-inflammatory phenotype, consequently promoting the development of insulin resistance originating from visceral adiposity (62–64). Additionally, B-2 cells have been demonstrated to have a pro-inflammatory function in the expansion of adipose tissue in rats consuming a high-fat diet (65). Moreover, in PCOS patients, this activation of B cells is closely linked to hyperandrogenism and the androgen receptor (66).
In line with mouse data, hyperandrogenemia also increases levels of IL-1β, IL-8, and IL-18 in PCOS patients (67). IL-8 functions as a pro-inflammatory factor that attracts neutrophils to adipose tissue, contributing to inflammation and IR (68). Furthermore, in obese PCOS individuals, there are changes in the levels of the pro-inflammatory cytokine leptin. Leptin triggers the phosphorylation of JAK2, STAT3, p38MAPK, and ERK1/2 in B-cells, decreases the expression of apoptotic factors, enhances B-cell survival, and promotes Th1 cell differentiation (69, 70). Limited research has focused on the pro-inflammatory mechanisms of B cells in insulin-resistant PCOS patients. Additionally, the association between B cells and macrophage polarization mechanisms in dysfunctional adipose tissue requires further exploration.
Dendritic cells (DCs) are antigen-presenting cells that play a role in both innate and acquired immunity. They are categorized based on morphology into conventional DCs (CDCs) and plasma cell-like DCs (PDCs) (71). DCs express surface markers like MHCII, CD11b, CD11c, and C-X3-C motif chemokine receptor 1, which are shared with macrophages. This similarity indicates that in dysfunctional adipose tissue, DCs function akin to macrophages (72, 73). Unlike many other immune cells that undergo substantial proliferation, DCs proliferate within tissues, albeit to a lesser extent (74, 75).
CDCs in adipose tissue can be subdivided into cDC1 (CD4- CD8α+ CD103+ CD205+ CD11b- CLEC9A+ XCR1+ CD24+ MHCII-) (76–78) and cDC2 (CD4+/- CD8α- CD205-CD11b+ CLEC4A4+ CD24+ MHCII+) (79, 80) based on descent. Both types of DCs increase in obese or inflamed tissues. cDC1 cells lacking MHCII are involved in cross-presentation to CD8+ T cells, leading to an expansion of CD8+ cells (81). The lack of MHCII expression in cDC1 and the decrease in the total number of CD11c+ cells enhance the body’s insulin sensitivity and lower the likelihood of IR. Nevertheless, this evidence alone does not imply a direct involvement of CD11c+ DCs in IR; CD11c+ macrophages also contribute to the reduction in inflammation levels (77).
Additionally, human monocyte-derived dendritic cells (moDCs) express CD14+ and are considered precursors of inflammatory dendritic cells (82). Conventional dendritic cell type 2 (cDC2) triggers CD4+ T-cell differentiation and exhibits a protective effect against adipose tissue inflammation. This effect induces IL-10 production through the activation of the Wnt/CD11b-catenin pathway in cDC2 cells [CD11c(hi) MHCII+ CD11b-], which typically express MHCII. Conversely, the previous pro-inflammatory function can be suppressed in dendritic cells [CD11c(hi) MHCII+ CD11b+] by activating the PPARγ pathway (83). Both mechanisms may contribute to IR in patients with PCOS. Moreover, the influence of serum estradiol levels on gonadotropins in PCOS patients impacts the maturation of CD11c+HLADR+ dendritic cells in human follicular fluid, consequently affecting IR levels (84). However, the precise molecular mechanism underlying this process remains unknown.
NK cells, which are innate lymphoid-like cells (ILCs) originating from the bone marrow, are widely distributed in various organ tissues and serve specific immune functions. Studies have shown that NK cells constitute 13% of immune cells in visceral fat and contribute to the inflammatory polarization of the immune response (85, 86). Inflammatory conditions predominantly regulate the local activity of NK cells through IL-12, IL-15, and IL-18 produced by dendritic cells and macrophages, with a particular focus on the extensively researched role of IL-15 (87–89).
IL-15 is highly expressed in the follicular fluid of PCOS patients and is positively correlated with serum testosterone levels. Treatment with IL-15 enhances the expression of Cytochrome P450 17A1 (CYP17A1) in granulosa cells, a key enzyme for androgen synthesis. Elevated levels of CYP17A1 lead to increased production of DHEA, a precursor for most androgens. Elevated androgen levels significantly contribute to inflammation and inflammation-induced IR (90).
In an inflammatory state, the expression of adipocyte NKp46 (NCR1) ligand is upregulated, and IL-15 binds to IL-15Rα on NK cell membranes, activating NK cells to produce IFN-γ. This promotes the polarization of CD4+ cells and macrophages towards an inflammatory state, contributing to the development of IR. Aggregated macrophages secrete large amounts of chemokines such as C-C motif chemokine ligand 3(CCL3), C-C motif chemokine ligand 4(CCL4), and chemokine CXC ligand 10 (CXCL10), which facilitate the recruitment of NK cells (91, 92).
Adipose tissue macrophage inflammation and IR can be improved by inhibiting NK cell function using neutralizing antibodies or through E4bp4 heterozygous knockout in mice (93). This is because inhibiting NK cell function leads to decreased expression of TNF-α and IL-1β, while increasing the expression of anti-inflammatory IL-10 and Arg1. Furthermore, leptin and lipocalin, linked to obesity, also regulate NK cell function. Short-term exposure to leptin enhances NK cell cytotoxicity and IFN-γ levels, whereas long-term exposure has the opposite effect. Conversely, lipocalin consistently suppresses NK cell function, irrespective of treatment duration (94, 95). This data indicates that in obese patients with PCOS, elevated leptin levels and/or decreased lipocalin levels activate NK cells, promoting the initiation and progression of inflammatory responses in adipose tissue, partially elucidating the development of IR in obese PCOS patients.
Due to significant phenotypic diversity and individual variances in PCOS patients, limited research has focused solely on NK cells, their cytokines, and their precise mechanisms.
Granulocytes are a type of leukocytes characterized by specific cytoplasmic granules. They are classified into major subgroups, including eosinophils, basophils, and neutrophils. In the complex inflammatory environment of PCOS patients, granulocytes are rapidly generated in the bloodstream, contributing to the immune response (96, 97). Specifically, the neutrophil-to-lymphocyte ratio (NLR) showed a positive correlation with HOMA-IR and serum insulin levels, irrespective of the obesity level in PCOS patients. This indicates a potential higher proliferation of granulocytes compared to lymphocytes in PCOS patients. Among the three types of granular leukocytes, neutrophil count and its proportion in leukocytes are commonly utilized as serum inflammation markers. Lourdes et al. observed that in PCOS patients with both hyperandrogenemia and hyperinsulinemia, the elevated white blood cell counts were primarily attributed to increased neutrophils. Moreover, glucose-lowering medications effectively suppressed the rise in neutrophils, thereby alleviating the inflammatory response in patients (98). Currently, it is believed that the mechanism of neutrophils affecting IR in PCOS is linked to myeloperoxidase (MPO). This belief stems from the higher MPO levels in leukocytes of PCOS patients compared to controls, with a more significant increase in the presence of IR (97, 99). However, due to the harsh culture conditions of granulocytes and the difficulty of detection, no authoritative study has yet revealed the specific mechanism of their role in IR in PCOS patients.
Overall, the mechanisms by which immune cells influence insulin resistance in PCOS patients are intricate and varied, necessitating further investigation into the functions of most immune cells (Figure 3).
Figure 3. The role of immune cells in IR in patients with PCOS: Abnormal proliferation of immune cells can cause immune dysfunction or imbalance in the ratio of immune-related factors. Several immune cells are involved in the inflammatory response, such as macrophages, T cells, B cells, granulocytes, dc, and NK cells, which can initiate or inhibit the host’s inflammatory response through the production of proinflammatory cytokines or suppressor cytokines. dc, dendritic cell; NK, natural killer cell.
The tumor necrosis factor (TNF) superfamily comprises 19 ligands and 29 receptors (100), all of which demonstrate proinflammatory activity. In the 1960s, a factor that induces tumor regression was discovered and named TNF-α. TNF-α was initially identified in macrophages and serves as a prototype of the TNF ligand family. Upon activation, TNF-α initially generates a transmembrane protein (tmTNF-α) in adipocytes and subsequently engages in signaling via two receptors, TNF-R1 and TNF-R2 (101, 102). Two transcription factors, NF-κB, and activator protein-1 (AP-1), have been identified to participate in the signaling process (103, 104). This factor was detectable in various components of the human oocyte-corona cumulus complex as early as fifty years ago (105, 106). Currently, TNF-α, a significant inflammation marker in vivo for PCOS patients, also impacts the clinical characterization of PCOS patients in diverse manners (107).
The molecular levels of GLUT-4 and IRS1, associated with glucose membrane transport and glucose uptake respectively, were significantly lower in PCOS patients with BMI compared to normal women (108–110). Conversely, TNF-α may contribute to insulin resistance in PCOS patients by inhibiting tyrosine kinase phosphorylation of IRS and reducing the biological activity of GLUT-4 (111). Statistically significant variations were also noted in the expression levels of TNF-α and lipocalin in obese women with PCOS compared to those without PCOS (112, 113). A negative correlation was observed between the two variables. In vitro experiments elucidate this relationship: TNF-α decreases lipocalin expression and secretion, while lipocalin influences TNF-α-induced proinflammatory and insulin inhibitory effects (114–117). It is evident that in patients with PCOS, TNF-α, known for its pro-inflammatory role, plays a predominant role. NF-κB, a transcription factor influenced by TNF-α, may also contribute to insulin resistance through a specific mechanism linked to its significant upregulation of inflammatory interleukins (e.g., IL-1β, IL-6) upon activation (118).
Interleukin-6 (IL-6), a multifunctional signaling molecule, is primarily produced by immune cells, epithelial cells, and tumor cells (119, 120). In the field of reproductive endocrinology, its key roles include regulating gonadotropin secretion, implantation, luteal function, and embryo development. IL-6’s activity is triggered by various pro-inflammatory molecules like TNF-α, interferon-γ (IFN-γ), and IL-1 (121). Initial studies indicate that IL-6 may enhance lipolysis in human adipocytes cultured for 48 hours. Sennet et al. found that short-term IL-6 treatment (30-90 minutes) increased socs-3 expression in HepG2 cells and rat hepatocytes, potentially affecting insulin-induced IRS-1 tyrosine phosphorylation, p85 binding, and downstream PKB/Akt phosphorylation, with a similar mechanism implicated in T2DM (122–124). Higher serum IL-6 levels in normal-weight polycystic subjects suggest that elevated IL-6 levels in nonobese subjects with PCOS may also contribute to IR (125). Two other studies on purely obese patients and obese PCOS patients also found higher IL-6 expression in obese PCOS patients compared to purely obese patients (126, 127). Therefore, in obese conditions, androgens trigger immune responses in patients with polycystic ovaries, creating a mutually reinforcing relationship between polycystic changes, hyperandrogenism, and insulin resistance. The more complex mechanisms of IL-6 need further exploration.
The pro-inflammatory cytokine IL-17 is associated with tissue inflammation induction. This cytokine family comprises six members, IL-17A to IL-17F, with IL-17A and IL-17F being closely related and co-expressed on linked genes. IL-17 is sourced from both Th17 and Th2 cells, while IL-17E is specifically from Th2 cells and enhances the Th2 pathway’s activity. Previous studies have demonstrated a significant increase in Th17 cells in PCOS patients, with high concentrations of IL-17A, a characteristic Th17 cytokine, compared to the control group.
IL-17 plays a role in adaptive immunity and in the production and interaction of innate immune cells on tissue cells, so it is reasonable to assume that it is involved in the low-grade chronic inflammation produced by adipose tissue as an important participating component. Furthermore, IL-6, a signaling molecule necessary for the differentiation of CD4+ cells into the Th17 lineage (128, 129), is a major downstream gene target of IL-17 (130, 131). A study by Fulghesu et al. showed that IL-6 concentrations were much higher in the sera of patients with the same PCOS who also had IR than in those without IR (132). Similar findings were obtained in obesity-promoting Th17 cells in expanded diet-induced obese mice, where Th17 cell numbers were significantly increased in wild-type genes, whereas the response of Th17 cells was not enhanced in IL-6-deficient mice (133). These studies suggest that, to some extent, the chronic inflammation that generates IR is dependent on the associated response of Th17 cells, which in turn is dependent on the activation of IL-6. CCAAT/enhancer binding protein (C/EBP) β and C/EBPδ are important transcription factors that promote adipocyte differentiation (134), and during adipogenesis, C/EPBβ is sequentially phosphorylated at three sites (Thr188, ser184 and Thr179) in its internal regulatory structural domain (135). Although IL-17 inhibits adipogenesis, it induces an increase in the abundance of C/EBPβ and C/EBPδ in lipogenic cell lines (130, 136) and induces phosphorylation at Thr188 and Thr179 sites (137). A study showed that IL -17 triggered a significant increase in IL-6 levels in differentiated adipocytes in culture of human mesenchymal cells (138). Increased IL-6 stimulates Th17 cell differentiation, which in turn may lead to an upregulation of IL-17-induced IL-6 levels. Although the relationship between IL-17 and IL-6 mutual stimulation has been demonstrated in many cells including adipocytes, the exact mechanism of IL-17 action on adipocytes and IR remains unknown, as does the promotion of CD4+ T cell differentiation into Th17 cells by IL-6.
Interleukin 18 (IL-18), also known as IF-γ-inducible factor, is a pro-inflammatory cytokine that in humans is encoded by the IL-18 gene. IL-18 belongs to the IL-1 superfamily and is produced by macrophages and other cells. Upon IL-18 stimulation, natural killer (NK) cells and some T cells release another important cytokine, IFN-γ or type II interferon, which in turn activates macrophages or other cells.
In adipose tissue, non-adipocytes, such as stromal vasculature and immune cells, are the primary source of IL-18 (139, 140). Simultaneously, immune cells synthesize IL-18Ra/b heterodimeric receptor complexes, to which IL-18 binds and plays a crucial role. Abnormal inflammation in adipose tissue leads to changes in IL-18R/IL-18 expression on CLS immune cells (141). Studies on animals have shown that mice with reduced IL-18 secretion exhibit elevated levels of adipose and pro-inflammatory factors in adipose tissue, resulting in glucose intolerance (142). However, a statistical analysis of diabetic patients revealed a positive correlation between elevated serum IL-18 and glycemic abnormalities after excluding the influence of BMI and adipokines. Similar results were observed in patients with PCOS and other low-grade chronic inflammatory conditions (107, 143, 144). This variation may be attributed to the diverse functions of IL-18 in different tissues (145). For instance, in bone marrow tissues, IL-18 primarily contributes to maintaining glucose homeostasis, while in tissues other than bone marrow, it mainly regulates the expansion of adipocytes (142). In individuals with adipose inflammation, IL-18 expression showed a negative correlation with IRS1, GLUT-4, lipocalin, and PPARγ expression (142), while demonstrating consistent changes with the severity of obesity, insulin resistance, lipid metabolism, and dyslipidemia, all indicating a significant role of IL-18 in insulin metabolism abnormalities (146–148).
Up to now, studies have identified nine members of the mammalian interferon regulatory factor (IRF) family: IRF1, IRF2, IRF3, IRF4 (PIP, LSIRF, or ICSAT), IRF5, IRF6, IRF7, IRF8 (ICSBP), and IRF9 (ISGF3γ) (149, 150). IRFs play a key role in the regulation of innate and acquired immunity as a transcriptional regulator of type I interferons and interferon-inducible genes (151). The structure of IRF and its function in tumor growth have been well described (152). This study aims to clarify the potential relationship among IRFs, immune cells, and IR in patients with PCOS (as shown in Table 1).
Secreted frizzled-related protein 4 (SFRP4) is a peptide hormone belonging to the SFRPs family, expressed in various tissues such as adipocytes, pancreas, and uterus (153–155). Elevated SFRP4 levels are observed in metabolic disorders linked to IR, like T2DM and gestational diabetes mellitus (GDM) (156, 157). SFRP4 plays a role in adipocyte differentiation, increasing adipokines, inducing oxidative stress in pancreatic cells with low antioxidant enzymes. Moreover, SFRP4 inhibits insulin extracellular secretion by reducing Ca2+ channel opening in pancreatic islet cells (153, 158). Wnt signaling (WNT5) showed a negative correlation with body weight in women with PCOS and controls (159). Acting as a Wnt signaling pathway antagonist, SFRP4 hinders Wnt proteins from binding to Wnt ligands or Fzd, thereby blocking the Wnt signaling pathway (160). These findings suggest that SFRP4 is directly or indirectly involved in the pathophysiologic pathway of IR.
Afamin was biochemically characterized as a vitamin E-binding protein found in follicular fluid, suggesting involvement in the reproductive system, particularly in follicle maturation (161, 162).
Vitamin E, a crucial antioxidant that combats oxidative stress in vivo, suggests that Afamin likely participates in anti-apoptotic processes related to oxidative stress (163). Two studies reported elevated levels of hyperglycemia-induced reactive oxygen species (ROS) and activated NF-kB in PCOS patients, resulting in TNF transcription, with the most significant impact observed in obese PCOS patients (164, 165).
Since the immune mechanism of PCOS is rarely studied and the research of immunotherapy for PCOS is still in the preliminary stage, so in this part, we summarized the existing immunotherapy methods and looked forward to more research and exploration in this field.
Tacrolimus (FK506) is an 822 kDa lipophilic macrolide antibiotic known for its potent immunosuppressive properties. It has been shown to inhibit the production of IFN-γ and IL-2 by activated T cells, as well as the aberrant expression of chemokine CXC ligand. This inhibition effectively suppresses the inflammatory response in target organs, such as the ovary and adipose tissue in murine models, thereby promoting ovarian ovulation and providing protection against IR (166–168).
Isotretinoin, a derivative of vitamin A, acts as an effective antiproliferative and antikeratinizing agent with certain immunosuppressive effects. A prospective clinical study conducted in Egypt investigated the impact of oral isotretinoin administration in patients with PCOS. The study reported a significant reduction in both free testosterone levels and acne scores following treatment. However, it also noted a considerable increase in serum triglyceride and cholesterol levels among the patients (169). Importantly, the study did not include measurements of insulin and glucose levels. These findings suggest that while isotretinoin may effectively modulate hormonal levels in PCOS patients, it does not appear to alleviate symptoms associated with metabolic disorders.
Mesenchymal stem cells (MSCs) significantly modulate the therapeutic immune response in a murine model of PCOS. Numerous studies have shown that MSC treatment markedly reduces serum expression of ovarian-specific genes and peripheral levels of the pro-inflammatory cytokine TNF-α in mice with PCOS. This effect may be mediated by the anti-inflammatory cytokine IL-10 (170–172). Peripheral blood flow cytometry analysis by Lamei Cheng et al. revealed that MSC treatment restored the proportions of macrophages and neutrophils in both peripheral blood and spleen to normal levels in the PCOS mouse model. Additionally, the ratio of pro-inflammatory M1 to anti-inflammatory M2 was appropriately adjusted. Variations in donor origin significantly affect the immunomodulatory effects of MSCs. Specifically, adipose-derived MSCs from obese or IR individuals show a markedly reduced ability to downregulate inflammatory factor expression and suppress CD4+ T cell activity. This reduced efficacy may result from intrinsic abnormalities in MSC function related to insulin resistance (173, 174). Currently, stem cell therapy targeting PCOS patients with IR, along with the underlying mechanisms affecting the immune system and stem cell abnormalities, has not progressed to the clinical research phase.
The clinical treatment strategy for IR induced by PCOS is comparable to that for type 2 diabetes. Lifestyle management is the preferred approach. Engaging in appropriate exercise and following a low glycemic index (LGI) diet can reduce waist circumference, total testosterone, low-density lipoprotein (LDL), fasting insulin, LDL cholesterol, triglycerides, and total cholesterol (175). The LGI diet decreases levels of growth hormone-releasing peptide while increasing glucagon levels. Physical activity and vigorous aerobic exercise enhance insulin sensitivity and androgen levels in patients with PCOS. Excessive fatty acid consumption and stabilization of hormone levels can effectively alleviate immune dysregulation in patients with PCOS. Lifestyle management is now widely promoted globally. Medications used as adjunctive treatments can effectively manage long-term complications.
Metformin (Met) is currently the primary therapeutic agent used for insulin sensitization in individuals with PCOS, while glucagon-like peptide-1 receptor agonists (GLP-1 RAs) are commonly employed in the treatment of T2DM. A study by Liao et al. investigated the efficacy of combining GLP-1 RAs and Met in reducing IL-6 and other molecules related to the inflammatory immune response, as evaluated through plasma proteomics (176). Additionally, Met has been shown to affect the levels of 11 cytokines in patients with PCOS, and its therapeutic efficacy is closely linked to androgen levels in these individuals (177).
The selection of pharmacological agents is crucial for improving insulin sensitivity and managing other metabolic disorders in patients with PCOS. As shown in the previous section, a significant association exists between the immune system and IR in patients with PCOS. Therefore, selecting medications that modulate inflammatory cytokine activity or inhibit the recruitment and proliferation of pro-inflammatory immune cells may offer a promising therapeutic strategy for future research.
In addition to medications, the incorporation of supplements may theoretically enhance the hypoglycemic effects of Met (e.g., sage, curcumin, quercetin, inositol, and various herbs) (178). However, aside from potential synergistic or additive effects with Met, no other known herb-drug interactions have been documented (179). Future therapeutic studies should investigate potential interactions between herbs or nutrients and drugs, as well as any immediate or long-term risks associated with these interactions.
The immune system is a crucial system present at birth that continuously undergoes adaptive changes and regulatory functions throughout an organism’s life. Several comorbidities of PCOS are associated with low-grade inflammation, indicating altered immune function (180). Significant alterations in cytokine levels, including TNF-α, IL-6, IL-17, IL-18, and ultrasensitive C-reactive protein (CRP), have been observed in the peripheral blood of PCOS patients. These changes may result from an increased proportion of abnormal immune cells, such as pro-inflammatory M1 macrophages, CD4+ T cells, NK cells, and neutrophils (181–183). This aberrant manifestation is present in specific tissues of PCOS patients, including adipose tissue, the endometrium, and certain ovarian cells (180). Consequently, inflammatory molecules have been defined as biomarkers of PCOS. Among the PCOS phenotypes discussed in this article, levels of CRP, TNF-α, and IL-6 correlate with IR, body weight, and adiposity in PCOS patients (184). TNF-α is found in the subcutaneous adipose tissue of PCOS patients, where it accumulates with macrophages, differentiates, and increases in density along the CLSS (185). Conversely, IL-6 functions as a signal transducer and transcriptional activator in the inflammatory response to IR, regulating various target genes, including IRS, AP-1, and NF-κB, to promote systemic and local inflammatory responses. However, large clinical cohort studies correlating multiple inflammatory cytokines with IR incidence and severity in PCOS patients are still needed to statistically correlate cytokines with IR incidence and severity before further interpretation of the potential of cytokines as immune markers for IR can be made.
Abnormal proportions of immune cells may serve as biological markers for the detection of PCOS. Currently, the proportions of T-cells, CD4+ T-cells, and NK-cells are recognized as independent risk factors for the development of PCOS (181). Although the mechanism of B-cells remains incompletely understood, studies indicate that the number of B-cells inclined to differentiate into plasma cells is elevated in the peripheral blood of PCOS patients (186). This differentiation tendency is characterized by the presence of CD19+. Furthermore, administering an anti-CD19 antibody in PCOS mouse models has been shown to reduce serum TNF-α levels and macrophage infiltration in adipose tissue (187). This finding not only suggests the potential for B-cell intervention in PCOS treatment but also indicates that flow cytometry analysis of peripheral blood cells may serve as an early immunodiagnostic marker for the disease. Immunodiagnosis during the pre-morbid phase of PCOS lacks substantial research support. However, surface leukocytes, the most prevalent type of immune cells, have been examined in this population during this timeframe. Investigating the clustering of differentiation antigens among immune cells during this period, as well as analyzing cytokine levels in various tissues of patients with different subclinical phenotypes, could yield more credible clinical evidence for early immune markers in PCOS patients.
In recent decades, significant progress has been made in identifying the causative factors of PCOS and clarifying its clinical symptom clusters; however, substantial gaps remain in our understanding of its biological mechanisms. Although IR is just one of the many clinical symptoms of PCOS, its long-term effects on the body are complex, including an increased risk of T2DM and cardiovascular disease. Various clinical interventions have been employed to manage PCOS and its related complications; however, without understanding the underlying causes of the disease, early intervention and delaying the onset of complications are not feasible. The immune system plays a crucial role in the pathogenesis of PCOS, characterized by its widespread distribution, diverse functions, and complex regulatory mechanisms. Investigating the detailed mechanisms of immune system actions is essential for tracing the origins and developing treatments for PCOS.
The prevalence of obesity in PCOS patients is 50-80% (188, 189). Mendelian randomization studies indicate a causal link between high BMI and PCOS risk, suggesting severe PCOS may increase BMI (190). Ethnicity, geography, and diet significantly affect the proportion of PCOS patients with high BMI globally. While the immunologic connection between high BMI, IR, and PCOS is well-studied, more research is needed on IR mechanisms in individuals with normal BMI.
Current research on the immune system in patients with PCOS primarily examines the distribution and abundance of immune cells and molecules. This includes the recruitment of pro-inflammatory M1 macrophages in peripheral blood and follicular fluid, alterations in the T1/T2 cell ratio, and an increase in B cells. Subsequently, the specific surface antigens of these immune cells are identified, and appropriate antibody therapies are administered. This approach aims to manage the progression of the inflammatory state, alleviate patient symptoms, and address the challenges associated with irregular and incomplete medication regimens. In addition to pharmacological treatment, standardizing lifestyle habits in PCOS patients can help reduce the risk and progression rate of the disease, particularly in those who are obese. Healthy dietary practices, regular exercise, and adequate sleep are effective strategies for reducing inflammatory factors in patients and modulating the distribution of immune cells. In recent years, there has been a surge in clinical trials investigating the effects of plant-derived products on PCOS. Natural compounds can antagonize certain pro-inflammatory cytokines and participate in the post-receptor signaling pathways of immune factors. They also enhance tissue cell sensitivity to insulin by influencing related molecules, including GLUT4 and IRS. Therefore, future investigations should focus on natural components to evaluate the purification effects of active ingredients responsible for the beneficial effects of these plants. Additionally, combining these with existing drugs could lead to the development of more effective formulations for hormone regulation and insulin enhancement in PCOS.
GMAS has identified over 20 genetic loci associated with PCOS; however, correlating these loci with the existing pathological mechanisms of PCOS remains a significant challenge. PCOS, an umbrella term for a group of clinical syndromes, shares common disease targets with T2DM, nonalcoholic fatty liver disease, and obesity (191). This overlap suggests potential mechanisms involving compensatory hyperinsulinemia, insulin resistance, systemic inflammation, cardiovascular disease, and pregnancy complications in PCOS patients. This suggests underlying mechanisms related to compensatory hyperinsulinemia, insulin resistance, T2DM, systemic inflammation, cardiovascular disease, and pregnancy complications in PCOS patients. Therefore, immunological studies of PCOS should extend beyond the female reproductive system to encompass metabolic changes throughout the body. This broader approach may uncover complex cellular interactions that facilitate the identification of biomarkers for predictive and targeted therapies, as well as improve predictions of therapeutic responses.
PCOS, as a group of metabolic disorder syndromes with diverse and heterogeneous symptoms, has received widespread attention due to its sudden increase in prevalence in the general population. Imbalances in the immune system could potentially contribute significantly to the diverse symptoms of PCOS. This paper examined IR in PCOS, along with its related cellular and molecular mechanisms (Figure 4). Immune cells interact with immune molecules, triggering diverse signaling pathways in the body including NF-κB, JNK, and PI3K/AKT. This interaction influences cellular sensitivity to insulin and glucose transport efficiency by enhancing the transcription of inflammatory genes. Thus, assessing the expression of immune molecules and the ratio of immune cells in PCOS patients is crucial for evaluating their inflammatory status and preventing potential long-term complications symptomatically. Nonetheless, numerous immune-related molecules in PCOS patients have unidentified functions in insulin resistance, exhibiting intricate and diverse roles in pathways, as well as diverse functions of immune cells. Additional research is required to clarify the molecular biological mechanisms of the immune system in PCOS-related symptoms and to gradually integrate these findings into clinical practice. This integration aims to enhance the long-term prognosis and outcomes for patients, offering novel therapeutic approaches.
Figure 4. Relationship between immunity and insulin resistance: Immune cells secrete different cytokines in different states, and IL-6 affects the function of IRS-1 by regulating the expression of SOCS-3 in insulin receptor-containing cells. Meanwhile, TNF-α regulates the transcription of inflammatory genes through JNK and NF-kB signaling pathways. Excessive SFA in the body affects the nuclear translocation of NF-kB and inhibits IRS-1-related functions via IKKB.IL-18 has been shown to have an effect on the development of insulin resistance, but the exact pathway of action is unknown. All of these factors contribute to the blockade of insulin action and glucose glucose transport, resulting in IR. IL, interleukin; IRS, insulin receptor substrate; SOCS, suppressor of cytokine signaling; GLUT, glucose transporter protein.
QZ: Writing – original draft, Writing – review & editing. ZY: Investigation, Writing – original draft. XO: Writing – original draft. MZ: Writing – original draft. XQ: Visualization, Writing – original draft. GW: Project administration, Resources, Writing – review & editing.
The author(s) declare financial support was received for the research, authorship, and/or publication of this article. This article was funded by The National Natural Science Foundation of China (No. 81873817).
Figure 1 was created with adobe illustrator, Figure 3 was created with BioRender software (http://BioRender.com), Figures 2 and 4 was created with Figdraw software (https://www.figdraw.com).
The authors declare that the research was conducted in the absence of any commercial or financial relationships that could be construed as a potential conflict of interest.
All claims expressed in this article are solely those of the authors and do not necessarily represent those of their affiliated organizations, or those of the publisher, the editors and the reviewers. Any product that may be evaluated in this article, or claim that may be made by its manufacturer, is not guaranteed or endorsed by the publisher.
1. Asuncion M, Calvo RM, San Millan JL, Sancho J, Avila S, Escobar-Morreale HF. A prospective study of the prevalence of the polycystic ovary syndrome in unselected Caucasian women from Spain. J Clin Endocrinol Metab. (2000) 85:2434–8. doi: 10.1210/jc.85.7.2434
2. Li R, Yu G, Yang D, Li S, Lu S, Wu X, et al. Prevalence and predictors of metabolic abnormalities in Chinese women with PCOS: a cross- sectional study. BMC Endocr Disord. (2014) 14:76. doi: 10.1186/1472-6823-14-76
3. Teede HJ, Tay CT, Laven J, Dokras A, Moran LJ, Piltonen TT, et al. Recommendations from the 2023 international evidence-based guideline for the assessment and management of polycystic ovary syndrome. Fertil Steril. (2023) 120:767–93. doi: 10.1016/j.fertnstert.2023.07.025
4. Lewandowski KC, Płusajska J, Horzelski W, Bieniek E, Lewiński A. Limitations of insulin resistance assessment in polycystic ovary syndrome. Endocr Connect. (2018) 7:403–12. doi: 10.1530/EC-18-0021
5. Lewandowski KC, Skowrońska-Jóźwiak E, Łukasiak K, Gałuszko K, Dukowicz A, Cedro M, et al. How much insulin resistance in polycystic ovary syndrome? Comparison of HOMA-IR and insulin resistance (Belfiore) index models. Arch Med Sci. (2019) 15:613–8. doi: 10.5114/aoms.2019.82672
6. Dapas M, Lin FTJ, Nadkarni GN, Sisk R, Legro RS, Urbanek M, et al. Distinct subtypes of polycystic ovary syndrome with novel genetic associations: An unsupervised, phenotypic clustering analysis. PloS Med. (2020) 17:e1003132. doi: 10.1371/journal.pmed.1003132
7. Heilbronn LK, Campbell LV. Adipose tissue macrophages, low grade inflammation and insulin resistance in human obesity. Curr Pharm Des. (2008) 14:1225–30. doi: 10.2174/138161208784246153
8. Li H, Meng Y, He S, Tan X, Zhang Y, Zhang X, et al. Macrophages, chronic inflammation, and insulin resistance. Cells. (2022) 11:3001. doi: 10.3390/cells11193001
9. Shan H, Luo R, Guo X, Li R, Ye Z, Peng T, et al. Abnormal endometrial receptivity and oxidative stress in polycystic ovary syndrome. Front Pharmacol. (2022) 13:904942. doi: 10.3389/fphar.2022.904942
10. Legro RS. Obesity and PCOS: implications for diagnosis and treatment. Semin Reprod Med. (2012) 30:496–506. doi: 10.1055/s-0032-1328878
11. Mannerås-Holm L, Leonhardt H, Kullberg J, Jennische E, Odén A, Holm G, et al. Adipose tissue has aberrant morphology and function in PCOS: enlarged adipocytes and low serum adiponectin, but not circulating sex steroids, are strongly associated with insulin resistance. J Clin Endocrinol Metab. (2011) 96:E304–E11. doi: 10.1210/jc.2010-1290
12. Weisberg SP, McCann D, Desai M, Rosenbaum M, Leibel RL, Ferrante AW. Obesity is associated with macrophage accumulation in adipose tissue. J Clin Invest. (2003) 112:1796–808. doi: 10.1172/JCI200319246
13. Kratz M, Coats BR, Hisert KB, Hagman D, Mutskov V, Peris E, et al. Metabolic dysfunction drives a mechanistically distinct proinflammatory phenotype in adipose tissue macrophages. Cell Metab. (2014) 20:614–25. doi: 10.1016/j.cmet.2014.08.010
14. Ouchi N, Parker JL, Lugus JJ, Walsh K. Adipokines in inflammation and metabolic disease. Nat Rev Immunol. (2011) 11:85–97. doi: 10.1038/nri2921
15. Murano I, Barbatelli G, Parisani V, Latini C, Muzzonigro G, Castellucci M, et al. Dead adipocytes, detected as crown-like structures, are prevalent in visceral fat depots of genetically obese mice. J Lipid Res. (2008) 49:1562–8. doi: 10.1194/jlr.M800019-JLR200
16. Li N, Wang X, Wang X, Yu H, Lin L, Sun C, et al. Upregulation of foxO 1 signaling mediates the proinflammatory cytokine upregulation in the macrophage from polycystic ovary syndrome patients. Clin Lab. (2017) 63:301–11. doi: 10.7754/Clin.Lab.2016.160514
17. Su D, Coudriet GM, Hyun Kim D, Lu Y, Perdomo G, Qu S, et al. FoxO1 links insulin resistance to proinflammatory cytokine IL-1beta production in macrophages. Diabetes. (2009) 58:2624–33. doi: 10.2337/db09-0232
18. Wang YC, Ma HD, Yin XY, Wang YH, Liu QZ, Yang JB, et al. Forkhead box O1 regulates macrophage polarization following staphylococcus aureus infection: experimental murine data and review of the literature. Clin Rev Allergy Immunol. (2016) 51:353–69. doi: 10.1007/s12016-016-8531-1
19. Lee S, Usman TO, Yamauchi J, Chhetri G, Wang X, Coudriet GM, et al. Myeloid FoxO1 depletion attenuates hepatic inflammation and prevents nonalcoholic steatohepatitis. J Clin Invest. (2022) 132:e154333. doi: 10.1172/JCI154333
20. Hotamisligil GS. Inflammation and metabolic disorders. Nature. (2006) 444:860–7. doi: 10.1038/nature05485
21. Tesz GJ, Guilherme A, Guntur KVP, Hubbard AC, Tang XQ, Chawla A, et al. Tumor necrosis factor alpha (TNF alpha) stimulates Map4k4 expression through TNF alpha receptor 1 signaling to c-Jun and activating transcription factor 2. J Biol Chem. (2007) 282:19302–12. doi: 10.1074/jbc.M700665200
22. Samuel VT, Shulman GI. Mechanisms for insulin resistance: common threads and missing links. Cell. (2012) 148:852–71. doi: 10.1016/j.cell.2012.02.017
23. Dunaif A, Segal KR, Shelley DR, Green G, Dobrjansky A, Licholai T. Evidence for distinctive and intrinsic defects in insulin action in polycystic ovary syndrome. Diabetes. (1992) 41:1257–66. doi: 10.2337/diab.41.10.1257
24. Rosenbaum D, Haber RS, Dunaif A. Insulin resistance in polycystic ovary syndrome: decreased expression of GLUT-4 glucose transporters in adipocytes. Am J Physiol. (1993) 264:E197–202. doi: 10.1152/ajpendo.1993.264.2.E197
25. Vujicic M, Broderick I, Salmantabar P, Perian C, Nilsson J, Sihlbom Wallem C, et al. A macrophage-collagen fragment axis mediates subcutaneous adipose tissue remodeling in mice. Proc Natl Acad Sci U S A. (2024) 121:e2313185121. doi: 10.1073/pnas.2313185121
26. Jeanes YM, Reeves S. Metabolic consequences of obesity and insulin resistance in polycystic ovary syndrome: diagnostic and methodological challenges. Nutr Res Rev. (2017) 30:97–105. doi: 10.1017/S0954422416000287
27. He FF, Li YM. Role of gut microbiota in the development of insulin resistance and the mechanism underlying polycystic ovary syndrome: a review. J Ovarian Res. (2020) 13:73. doi: 10.1186/s13048-020-00670-3
28. Moro F, Morciano A, Tropea A, Sagnella F, Palla C, Scarinci E, et al. Effects of drospirenone-ethinylestradiol and/or metformin on CD4(+)CD28(null) T lymphocytes frequency in women with hyperinsulinemia having polycystic ovary syndrome: A randomized clinical trial. Reprod Sci. (2013) 20:1508–17. doi: 10.1177/1933719113488444
29. Michalek RD, Gerriets VA, Jacobs SR, Macintyre AN, MacIver NJ, Mason EF, et al. Cutting edge: distinct glycolytic and lipid oxidative metabolic programs are essential for effector and regulatory CD4+ T cell subsets. J Immunol. (2011) 186:3299–303. doi: 10.4049/jimmunol.1003613
30. Raphael I, Nalawade S, Eagar TN, Forsthuber TG. T cell subsets and their signature cytokines in autoimmune and inflammatory diseases. Cytokine. (2015) 74:5–17. doi: 10.1016/j.cyto.2014.09.011
31. Dumitru C, Kabat AM, Maloy KJ. Metabolic adaptations of CD4+ T cells in inflammatory disease. Front Immunol. (2018) 9:17. doi: 10.3389/fimmu.2018.00540
32. Wu H, Ghosh S, Perrard XD, Feng L, Garcia GE, Perrard JL, et al. T-cell accumulation and regulated on activation, normal T cell expressed and secreted upregulation in adipose tissue in obesity. Circulation. (2007) 115:1029–38. doi: 10.1161/CIRCULATIONAHA.106.638379
33. Wang Q, Wu H. T cells in adipose tissue: critical players in immunometabolism. Front Immunol. (2018) 9:2509. doi: 10.3389/fimmu.2018.02509
34. Nasri F, Doroudchi M, Namavar Jahromi B, Gharesi-Fard B. T helper cells profile and CD4+CD25+Foxp3+Regulatory T cells in polycystic ovary syndrome. Iran J Immunol. (2018) 15:175–85. doi: 10.22034/IJI.2018.39387
35. Ioan-Facsinay A, Kwekkeboom JC, Westhoff S, Giera M, Rombouts Y, van Harmelen V, et al. Adipocyte-derived lipids modulate CD4+ T-cell function. Eur J Immunol. (2013) 43:1578–87. doi: 10.1002/eji.201243096
36. Surendar J, Frohberger SJ, Karunakaran I, Schmitt V, Stamminger W, Neumann AL, et al. Adiponectin limits IFN-γ and IL-17 producing CD4 T cells in obesity by restraining cell intrinsic glycolysis. Front Immunol. (2019) 10:17. doi: 10.3389/fimmu.2019.02555
37. Rocha VZ, Folco EJ, Sukhova G, Shimizu K, Gotsman I, Vernon AH, et al. Interferon-gamma, a Th1 cytokine, regulates fat inflammation: a role for adaptive immunity in obesity. Circ Res. (2008) 103:467–76. doi: 10.1161/CIRCRESAHA.108.177105
38. McGillicuddy FC, Chiquoine EH, Hinkle CC, Kim RJ, Shah R, Roche HM, et al. Interferon gamma attenuates insulin signaling, lipid storage, and differentiation in human adipocytes via activation of the JAK/STAT pathway. J Biol Chem. (2009) 284:31936–44. doi: 10.1074/jbc.M109.061655
39. O’Rourke RW, White AE, Metcalf MD, Winters BR, Diggs BS, Zhu XX, et al. Systemic inflammation and insulin sensitivity in obese IFN-γ knockout mice. Metab-Clin Exp. (2012) 61:1152–61. doi: 10.1016/j.metabol.2012.01.018
40. Li Z, Peng A, Feng Y, Zhang X, Liu F, Chen C, et al. Detection of T lymphocyte subsets and related functional molecules in follicular fluid of patients with polycystic ovary syndrome. Sci Rep. (2019) 9:6040. doi: 10.1038/s41598-019-42631-x
41. Wang Z, Aguilar EG, Luna JI, Dunai C, Khuat LT, Le CT, et al. Paradoxical effects of obesity on T cell function during tumor progression and PD-1 checkpoint blockade. Nat Med. (2019) 25:141–51. doi: 10.1038/s41591-018-0221-5
42. Porsche CE, Delproposto JB, Geletka L, O’Rourke R, Lumeng CN. Obesity results in adipose tissue T cell exhaustion. JCI Insight. (2021) 6:e154333. doi: 10.1172/jci.insight.139793
43. Tsang JY, Li D, Ho D, Peng J, Xu A, Lamb J, et al. Novel immunomodulatory effects of adiponectin on dendritic cell functions. Int Immunopharmacol. (2011) 11:604–9. doi: 10.1016/j.intimp.2010.11.009
44. Dandona P, Aljada A, Mohanty P, Ghanim H, Hamouda W, Assian E, et al. Insulin inhibits intranuclear nuclear factor kappaB and stimulates IkappaB in mononuclear cells in obese subjects: evidence for an anti-inflammatory effect? J Clin Endocrinol Metab. (2001) 86:3257–65. doi: 10.1210/jcem.86.7.7623
45. Viardot A, Grey ST, Mackay F, Chisholm D. Potential antiinflammatory role of insulin via the preferential polarization of effector T cells toward a T helper 2 phenotype. Endocrinology. (2007) 148:346–53. doi: 10.1210/en.2006-0686
46. Proto JD, Doran AC, Gusarova G, Yurdagul A Jr., Sozen E, Subramanian M, et al. Regulatory T cells promote macrophage efferocytosis during inflammation resolution. Immunity. (2018) 49:666–77 e6. doi: 10.1016/j.immuni.2018.07.015
47. Sharma M, Schlegel MP, Afonso MS, Brown EJ, Rahman K, Weinstock A, et al. Regulatory T cells license macrophage pro-resolving functions during atherosclerosis regression. Circ Res. (2020) 127:335–53. doi: 10.1161/CIRCRESAHA.119.316461
48. Zou ML, Chen ZH, Teng YY, Liu SY, Jia Y, Zhang KW, et al. The smad dependent TGF-beta and BMP signaling pathway in bone remodeling and therapies. Front Mol Biosci. (2021) 8:593310. doi: 10.3389/fmolb.2021.593310
49. Vasanthakumar A, Chisanga D, Blume J, Gloury R, Britt K, Henstridge DC, et al. Sex-specific adipose tissue imprinting of regulatory T cells. Nature. (2020) 579:581–5. doi: 10.1038/s41586-020-2040-3
50. Korn T, Bettelli E, Oukka M, Kuchroo VK. IL-17 and th17 cells. Annu Rev Immunol. (2009) 27:485–517. doi: 10.1146/annurev.immunol.021908.132710
51. Kaminski DA, Randall TD. Adaptive immunity and adipose tissue biology. Trends Immunol. (2010) 31:384–90. doi: 10.1016/j.it.2010.08.001
52. Srikakulapu P, McNamara CA. B cells and atherosclerosis. Am J Physiol-Heart Circul Physiol. (2017) 312:H1060–H7. doi: 10.1152/ajpheart.00859.2016
53. Haas KM, Poe JC, Steeber DA, Tedder TF. B-1a and b-1b cells exhibit distinct developmental requirements and have unique functional roles in innate and adaptive immunity to S-pneumoniae. Immunity. (2005) 23:7–18. doi: 10.1016/j.immuni.2005.04.011
54. Allman D, Pillai S. Peripheral B cell subsets. Curr Opin Immunol. (2008) 20:149–57. doi: 10.1016/j.coi.2008.03.014
55. Duffaut C, Galitzky J, Lafontan M, Bouloumié A. Unexpected trafficking of immune cells within the adipose tissue during the onset of obesity. Biochem Biophys Res Commun. (2009) 384:482–5. doi: 10.1016/j.bbrc.2009.05.002
56. Mauri C, Ehrenstein MR. The ‘short’ history of regulatory B cells. Trends Immunol. (2008) 29:34–40. doi: 10.1016/j.it.2007.10.004
57. Xing G, Sun Y, Wang F, Gao G, Zhou X. Role of interleukin-10 polymorphisms and haplotypes in polycystic ovary syndrome risk. Int J Clin Exp Pathol. (2017) 10:7956–61.
58. Zhai Y, Pang Y. Systemic and ovarian inflammation in women with polycystic ovary syndrome. J Reprod Immunol. (2022) 151:103628. doi: 10.1016/j.jri.2022.103628
59. Kowalski GM, Nicholls HT, Risis S, Watson NK, Kanellakis P, Bruce CR, et al. Deficiency of haematopoietic-cell-derived IL-10 does not exacerbate high-fat-diet-induced inflammation or insulin resistance in mice. Diabetologia. (2011) 54:888–99. doi: 10.1007/s00125-010-2020-5
60. Dagdeviren S, Jung DY, Friedline RH, Noh HL, Kim JH, Patel PR, et al. IL-10 prevents aging-associated inflammation and insulin resistance in skeletal muscle. FASEB J. (2017) 31:701–10. doi: 10.1096/fj.201600832R
61. Kawai T, Autieri MV, Scalia R. Adipose tissue inflammation and metabolic dysfunction in obesity. Am J Physiol Cell Physiol. (2021) 320:C375–C91. doi: 10.1152/ajpcell.00379.2020
62. Winer S, Chan Y, Paltser G, Truong D, Tsui H, Bahrami J, et al. Normalization of obesity-associated insulin resistance through immunotherapy. Nat Med. (2009) 15:921–U126. doi: 10.1038/nm.2001
63. Catalan D, Mansilla MA, Ferrier A, Soto L, Oleinika K, Aguillon JC, et al. Immunosuppressive mechanisms of regulatory B cells. Front Immunol. (2021) 12:611795. doi: 10.3389/fimmu.2021.611795
64. Li J, Niu QM, Wu AW, Zhang YC, Hong LQ, Wang H. Causal relationship between circulating immune cells and the risk of type 2 diabetes: a Mendelian randomization study. Front Endocrinol. (2023) 14:6. doi: 10.3389/fendo.2023.1210415
65. Ying W, Wollam J, Ofrecio JM, Bandyopadhyay G, El Ouarrat D, Lee YS, et al. Adipose tissue B2 cells promote insulin resistance through leukotriene LTB4/LTB4R1 signaling. J Clin Invest. (2017) 127:1019–30. doi: 10.1172/JCI90350
66. Ascani A, Torstensson S, Risal S, Lu H, Eriksson G, Li C, et al. The role of B cells in immune cell activation in polycystic ovary syndrome. Elife. (2023) 12:e86454. doi: 10.7554/eLife.86454.sa2
67. Xiang Y, Wang H, Ding H, Xu T, Liu X, Huang Z, et al. Hyperandrogenism drives ovarian inflammation and pyroptosis: A possible pathogenesis of PCOS follicular dysplasia. Int Immunopharmacol. (2023) 125:111141. doi: 10.1016/j.intimp.2023.111141
68. Dabravolski SA, Nikiforov NG, Eid AH, Nedosugova LV, Starodubova AV, Popkova TV, et al. Mitochondrial dysfunction and chronic inflammation in polycystic ovary syndrome. Int J Mol Sci. (2021) 22:3923. doi: 10.3390/ijms22083923
69. Lai Y, Ye Z, Mu L, Zhang Y, Long X, Zhang C, et al. Elevated levels of follicular fatty acids induce ovarian inflammation via ERK1/2 and inflammasome activation in PCOS. J Clin Endocrinol Metab. (2022) 107:2307–17. doi: 10.1210/clinem/dgac281
70. Rui K, Che N, Ma K, Zou H, Xiao F, Lu L. Coming of age: the formation and function of age-associated B cells. Cell Mol Immunol. (2024) 21:311–2. doi: 10.1038/s41423-024-01143-z
71. Wu L, Liu YJ. Development of dendritic-cell lineages. Immunity. (2007) 26:741–50. doi: 10.1016/j.immuni.2007.06.006
72. Bertola A, Ciucci T, Rousseau D, Bourlier V, Duffaut C, Bonnafous S, et al. Identification of adipose tissue dendritic cells correlated with obesity-associated insulin-resistance and inducing Th17 responses in mice and patients. Diabetes. (2012) 61:2238–47. doi: 10.2337/db11-1274
73. Su Z, Sun JY, Gao M, Sun W, Kong X. Molecular mechanisms and potential therapeutic targets in the pathogenesis of hypertension in visceral adipose tissue induced by a high-fat diet. Front Cardiovasc Med. (2024) 11:1380906. doi: 10.3389/fcvm.2024.1380906
74. Cho KW, Zamarron BF, Muir LA, Singer K, Porsche CE, DelProposto JB, et al. Adipose tissue dendritic cells are independent contributors to obesity-induced inflammation and insulin resistance. J Immunol. (2016) 197:3650–61. doi: 10.4049/jimmunol.1600820
75. Silva HM, Bafica A, Rodrigues-Luiz GF, Chi J, Santos PDA, Reis BS, et al. Vasculature-associated fat macrophages readily adapt to inflammatory and metabolic challenges. J Exp Med. (2019) 216:786–806. doi: 10.1084/jem.20181049
76. Bedoui S, Whitney PG, Waithman J, Eidsmo L, Wakim L, Caminschi I, et al. Cross-presentation of viral and self antigens by skin-derived CD103+ dendritic cells. Nat Immunol. (2009) 10:488–95. doi: 10.1038/ni.1724
77. Stefanovic-Racic M, Yang X, Turner MS, Mantell BS, Stolz DB, Sumpter TL, et al. Dendritic cells promote macrophage infiltration and comprise a substantial proportion of obesity-associated increases in CD11c+ cells in adipose tissue and liver. Diabetes. (2012) 61:2330–9. doi: 10.2337/db11-1523
78. Meredith MM, Liu K, Darrasse-Jeze G, Kamphorst AO, Schreiber HA, Guermonprez P, et al. Expression of the zinc finger transcription factor zDC (Zbtb46, Btbd4) defines the classical dendritic cell lineage. J Exp Med. (2012) 209:1153–65. doi: 10.1084/jem.20112675
79. Tussiwand R, Everts B, Grajales-Reyes GE, Kretzer NM, Iwata A, Bagaitkar J, et al. Klf4 expression in conventional dendritic cells is required for T helper 2 cell responses. Immunity. (2015) 42:916–28. doi: 10.1016/j.immuni.2015.04.017
80. Noubade R, Majri-Morrison S, Tarbell KV. Beyond cDC1: emerging roles of DC crosstalk in cancer immunity. Front Immunol. (2019) 10:1014. doi: 10.3389/fimmu.2019.01014
81. Porsche CE, Delproposto JB, Patrick E, Zamarron BF, Lumeng CN. Adipose tissue dendritic cell signals are required to maintain T cell homeostasis and obesity-induced expansion. Mol Cell Endocrinol. (2020) 505:110740. doi: 10.1016/j.mce.2020.110740
82. Collin M, McGovern N, Haniffa M. Human dendritic cell subsets. Immunology. (2013) 140:22–30. doi: 10.1111/imm.2013.140.issue-1
83. Macdougall CE, Wood EG, Loschko J, Scagliotti V, Cassidy FC, Robinson ME, et al. Visceral adipose tissue immune homeostasis is regulated by the crosstalk between adipocytes and dendritic cell subsets. Cell Metab. (2018) 27:588–601.e4. doi: 10.1016/j.cmet.2018.02.007
84. Vankelecom H, Carmeliet P, Van Damme J, Billiau A, Denef C. Production of interleukin-6 by folliculo-stellate cells of the anterior pituitary gland in a histiotypic cell aggregate culture system. Neuroendocrinology. (1989) 49:102–6. doi: 10.1159/000125097
85. O’Rourke RW, Gaston GD, Meyer KA, White AE, Marks DL. Adipose tissue NK cells manifest an activated phenotype in human obesity. Metab-Clin Exp. (2013) 62:1557–61. doi: 10.1016/j.metabol.2013.07.011
86. Wensveen FM, Jelencic V, Valentic S, Sestan M, Wensveen TT, Theurich S, et al. NK cells link obesity-induced adipose stress to inflammation and insulin resistance. Nat Immunol. (2015) 16:376–85. doi: 10.1038/ni.3120
87. Ma A, Koka R, Burkett P. Diverse functions of IL-2, IL-15, and IL-7 in lymphoid homeostasis. Annu Rev Immunol. (2006) 24:657–79. doi: 10.1146/annurev.immunol.24.021605.090727
88. Vivier E, Raulet DH, Moretta A, Caligiuri MA, Zitvogel L, Lanier LL, et al. Innate or adaptive immunity? The example of natural killer cells. Science. (2011) 331:44–9. doi: 10.1126/science.1198687
89. Peng H, Tian Z. Natural killer cell memory: progress and implications. Front Immunol. (2017) 8:1143. doi: 10.3389/fimmu.2017.01143
90. Liu Y, Li Z, Wang Y, Cai Q, Liu H, Xu C, et al. IL-15 participates in the pathogenesis of polycystic ovary syndrome by affecting the activity of granulosa cells. Front Endocrinol (Lausanne). (2022) 13:787876. doi: 10.3389/fendo.2022.787876
91. Li Y, Wang F, Imani S, Tao L, Deng Y, Cai Y. Natural killer cells: friend or foe in metabolic diseases? Front Immunol. (2021) 12:614429. doi: 10.3389/fimmu.2021.614429
92. Lee H, Da Silva IP, Palendira U, Scolyer RA, Long GV, Wilmott JS. Targeting NK cells to enhance melanoma response to immunotherapies. Cancers (Basel). (2021) 13:1363. doi: 10.3390/cancers13061363
93. Lee BC, Kim MS, Pae M, Yamamoto Y, Eberle D, Shimada T, et al. Adipose natural killer cells regulate adipose tissue macrophages to promote insulin resistance in obesity. Cell Metab. (2016) 23:685–98. doi: 10.1016/j.cmet.2016.03.002
94. Wrann CD, Laue T, Hubner L, Kuhlmann S, Jacobs R, Goudeva L, et al. Short-term and long-term leptin exposure differentially affect human natural killer cell immune functions. Am J Physiol Endocrinol Metab. (2012) 302:E108–16. doi: 10.1152/ajpendo.00057.2011
95. Nosalski R, Guzik TJ. Perivascular adipose tissue inflammation in vascular disease. Br J Pharmacol. (2017) 174:3496–513. doi: 10.1111/bph.v174.20
96. Yilmaz MA, Duran C, Basaran M. The mean platelet volume and neutrophil to lymphocyte ratio in obese and lean patients with polycystic ovary syndrome. J Endocrinol Invest. (2016) 39:45–53. doi: 10.1007/s40618-015-0335-2
97. Victor VM, Rovira-Llopis S, Banuls C, Diaz-Morales N, de Maranon AM, Rios-Navarro C, et al. Insulin resistance in PCOS patients enhances oxidative stress and leukocyte adhesion: role of myeloperoxidase. PloS One. (2016) 11:e0151960. doi: 10.1371/journal.pone.0151960
98. Ibanez L, Jaramillo AM, Ferrer A, de Zegher F. High neutrophil count in girls and women with hyperinsulinaemic hyperandrogenism: normalization with metformin and flutamide overcomes the aggravation by oral contraception. Hum Reprod. (2005) 20:2457–62. doi: 10.1093/humrep/dei072
99. Ribeiro AL, Scapinelli A, Tamanaha S, de Oliveira RM, Kowastch I, Mathias W, et al. Myeloperoxidases and polycystic ovary syndrome. Gynecol Endocrinol. (2012) 28:3–6. doi: 10.3109/09513590.2011.579656
100. Aggarwal BB, Gupta SC, Kim JH. Historical perspectives on tumor necrosis factor and its superfamily: 25 years later, a golden journey. Blood. (2012) 119:651–65. doi: 10.1182/blood-2011-04-325225
101. Moller DE. Potential role of TNF-alpha in the pathogenesis of insulin resistance and type 2 diabetes. Trends Endocrinol Metab. (2000) 11:212–7. doi: 10.1016/S1043-2760(00)00272-1
102. Olmos G, Lladó J. Tumor necrosis factor alpha: a link between neuroinflammation and excitotoxicity. Mediators Inflamm. (2014) 2014:861231. doi: 10.1155/2014/861231
103. Leong KG, Karsan A. Signaling pathways mediated by tumor necrosis factor alpha. Histol Histopathol. (2000) 15:1303–25. doi: 10.14670/HH-15.1303
104. Oeckinghaus A, Ghosh S. The NF-kappaB family of transcription factors and its regulation. Cold Spring Harb Perspect Biol. (2009) 1:a000034. doi: 10.1101/cshperspect.a000034
105. Emoto N, Baird A. The effect of tumor necrosis factor cachectin on follicle-stimulating hormone-induced aromatase-activity in cultured rat granulosa-cells. Biochem Biophys Res Commun. (1988) 153:792–8. doi: 10.1016/S0006-291X(88)81165-3
106. Brannstrom M, Bonello N, Wang LJ, Norman RJ. Effects of tumor-necrosis-factor-alpha (tnf-alpha) on ovulation in the rat ovary. Reprod Fertil Dev. (1995) 7:67–73. doi: 10.1071/RD9950067
107. Rudnicka E, Suchta K, Grymowicz M, Calik-Ksepka A, Smolarczyk K, Duszewska AM, et al. Chronic low grade inflammation in pathogenesis of PCOS. Int J Mol Sci. (2021) 22:3789. doi: 10.3390/ijms22073789
108. Rosas C, Gabler F, Vantman D, Romero C, Vega M. Levels of Rabs and WAVE family proteins associated with translocation of GLUT4 to the cell surface in endometria from hyperinsulinemic PCOS women. Hum Reprod. (2010) 25:2870–7. doi: 10.1093/humrep/deq232
109. Fornes R, Ormazabal P, Rosas C, Gabler F, Vantman D, Romero C, et al. Changes in the expression of insulin signaling pathway molecules in endometria from polycystic ovary syndrome women with or without hyperinsulinemia. Mol Med. (2010) 16:129–36. doi: 10.2119/molmed.2009.00118
110. Carvajal R, Rosas C, Kohan K, Gabler F, Vantman D, Romero C, et al. Metformin augments the levels of molecules that regulate the expression of the insulin-dependent glucose transporter GLUT4 in the endometria of hyperinsulinemic PCOS patients. Hum Reprod. (2013) 28:2235–44. doi: 10.1093/humrep/det116
111. Lonardo MS, Cacciapuoti N, Guida B, Di Lorenzo M, Chiurazzi M, Damiano S, et al. Hypothalamic-ovarian axis and adiposity relationship in polycystic ovary syndrome: physiopathology and therapeutic options for the management of metabolic and inflammatory aspects. Curr Obes Rep. (2024) 13:51–70. doi: 10.1007/s13679-023-00531-2
112. Baldani DP, Skrgatic L, Kasum M, Zlopasa G, Oguic SK, Herman M. Altered leptin, adiponectin, resistin and ghrelin secretion may represent an intrinsic polycystic ovary syndrome abnormality. Gynecol Endocrinol. (2019) 35:401–5. doi: 10.1080/09513590.2018.1534096
113. Bannigida DM, Nayak SB, Vijayaragavan R. Serum visfatin and adiponectin - markers in women with polycystic ovarian syndrome. Arch Physiol Biochem. (2020) 126:283–6. doi: 10.1080/13813455.2018.1518987
114. Wang BH, Jenkins JR, Trayhurn P. Expression and secretion of inflammation-related adipokines by human adipocytes differentiated in culture: integrated response to TNF-alpha. Am J Physiol-Endocrinol Metab. (2005) 288:E731–E40. doi: 10.1152/ajpendo.00475.2004
115. Wang BH, Trayhurn P. Acute and prolonged effects of TNF-alpha on the expression and secretion of inflammation-related adipokines by human adipocytes differentiated in culture. Pflugers Arch. (2006) 452:418–27. doi: 10.1007/s00424-006-0055-8
116. Guerre-Millo M. Adiponectin: an update. Diabetes Metab. (2008) 34:12–8. doi: 10.1016/j.diabet.2007.08.002
117. Mirza SS, Shafique K, Shaikh AR, Khan NA, Qureshi MA. Association between circulating adiponectin levels and polycystic ovarian syndrome. J Ovarian Res. (2014) 7:7. doi: 10.1186/1757-2215-7-18
118. Orostica L, Garcia P, Vera C, Garcia V, Romero C, Vega M. Effect of TNF-alpha on molecules related to the insulin action in endometrial cells exposed to hyperandrogenic and hyperinsulinic conditions characteristics of polycystic ovary syndrome. Reprod Sci. (2018) 25:1000–9. doi: 10.1177/1933719117732157
119. Nilsson MB, Langley RR, Fidler IJ. Interleukin-6, secreted by human ovarian carcinoma cells, is a potent proangiogenic cytokine. Cancer Res. (2005) 65:10794–800. doi: 10.1158/0008-5472.CAN-05-0623
120. Hrvat A, Schmidt M, Wagner B, Zwanziger D, Kimmig R, Volbracht L, et al. Electrolyte imbalance causes suppression of NK and T cell effector function in Malignant ascites. J Exp Clin Cancer Res. (2023) 42:235. doi: 10.1186/s13046-023-02798-8
121. Mukherjee AG, Wanjari UR, Kannampuzha S, Murali R, Namachivayam A, Ganesan R, et al. The implication of mechanistic approaches and the role of the microbiome in polycystic ovary syndrome (PCOS): A review. Metabolites. (2023) 13:129. doi: 10.3390/metabo13010129
122. Trujillo ME, Kalyoussef S, Brolin RE, Schneider SH, Greenberg AS, Fried SK. Interleukin-6 increases lipolysis and leptin release in cultured human adipose tissue. Diabetes. (2001) 50:A12–A.
123. Klover P, Zimmers T, Koniaris L, Nowak I, Senn J, Mooney R. Acute or chronic exposure to interleukin-6 induces hepatic insulin resistance in vivo. Diabetes. (2003) 52:A290–A. doi: 10.2337/diabetes.52.11.2784
124. Rehman K, Akash MSH, Liaqat A, Kamal S, Qadir MI, Rasul A. Role of interleukin-6 in development of insulin resistance and type 2 diabetes mellitus. Crit Rev Eukaryot Gene Expr. (2017) 27:229–36. doi: 10.1615/CritRevEukaryotGeneExpr.2017019712
125. Tarkun I, Cetinarslan B, Turemen E, Canturk Z, Biyikli M. Association between circulating tumor necrosis factor-alpha, interleukin-6, and insulin resistance in normal-weight women with polycystic ovary syndrome. Metab syndrome related Disord. (2006) 4:122–8. doi: 10.1089/met.2006.4.122
126. Bruun JM, Verdich C, Toubro S, Astrup A, Richelsen B. Association between measures of insulin sensitivity and circulating levels of interleukin-8, interleukin-6 and tumor necrosis factor-alpha. Effect of weight loss in obese men. Eur J Endocrinol. (2003) 148:535–42. doi: 10.1530/eje.0.1480535
127. Gonzalez F, Sia CL, Stanczyk FZ, Blair HE, Krupa ME. Hyperandrogenism exerts an anti-inflammatory effect in obese women with polycystic ovary syndrome. Endocrine. (2012) 42:726–35. doi: 10.1007/s12020-012-9728-6
128. Harrington LE, Hatton RD, Mangan PR, Turner H, Murphy TL, Murphy KM, et al. Interleukin 17-producing CD4(+) effector T cells develop via a lineage distinct from the T helper type 1 and 2 lineages. Nat Immunol. (2005) 6:1123–32. doi: 10.1038/ni1254
129. Park H, Li Z, Yang XO, Chang SH, Nurieva R, Wang YH, et al. A distinct lineage of CD4 T cells regulates tissue inflammation by producing interleukin 17. Nat Immunol. (2005) 6:1133–41. doi: 10.1038/ni1261
130. Ruddy MJ, Wong GC, Liu XKK, Yamamoto H, Kasayama S, Kirkwood KL, et al. Functional cooperation between interleukin-17 and tumor necrosis factor-alpha is mediated by CCAAT/enhancer-binding protein family members. J Biol Chem. (2004) 279:2559–67. doi: 10.1074/jbc.M308809200
131. Shen F, Ruddy MJ, Plamondon P, Gaffen SL. Cytokines link osteoblasts and inflammation: microarray analysis of interleukin-17-and TNF-alpha-induced genes in bone cells. J Leuk Biol. (2005) 77:388–99. doi: 10.1189/jlb.0904490
132. Fulghesu AM, Sanna F, Uda S, Magnini R, Portoghese E, Batetta B. IL-6 serum levels and production is related to an altered immune response in polycystic ovary syndrome girls with insulin resistance. Mediators Inflamm. (2011) 2011:389317. doi: 10.1155/2011/389317
133. Winer S, Paltser G, Chan Y, Tsui H, Engleman E, Winer D, et al. Obesity predisposes to Th17 bias. Eur J Immunol. (2009) 39:2629–35. doi: 10.1002/eji.200838893
134. Rosen ED, MacDougald OA. Adipocyte differentiation from the inside out. Nat Rev Mol Cell Bio. (2006) 7:885–96. doi: 10.1038/nrm2066
135. Tang QQ, Gronborg M, Huang HY, Kim JW, Otto TC, Pandey A, et al. Sequential phosphorylation of CCAAT enhancer-binding protein beta by MAPK and glycogen synthase kinase 3 beta is required for adipogenesis. Proc Natl Acad Sci U S A. (2005) 102:9766–71. doi: 10.1073/pnas.0503891102
136. Ding J, Nagai K, Woo JT. Insulin-dependent adipogenesis in stromal ST2 cells derived from murine bone marrow. Biosci Biotechnol Biochem. (2003) 67:314–21. doi: 10.1271/bbb.67.314
137. Shen F, Li N, Gade P, Kalvakolanu DV, Weibley T, Doble B, et al. IL-17 receptor signaling inhibits C/EBP beta by sequential phosphorylation of the regulatory 2 domain. Sci Signal. (2009) 2:10. doi: 10.1126/scisignal.2000066
138. Shin JH, Shin DW, Noh M. Interleukin-17A inhibits adipocyte differentiation in human mesenchymal stem cells and regulates pro-inflammatory responses in adipocytes. Biochem Pharmacol. (2009) 77:1835–44. doi: 10.1016/j.bcp.2009.03.008
139. Fain JN, Tichansky DS, Madan AK. Most of the interleukin 1 receptor antagonist, cathepsin S, macrophage migration inhibitory factor, nerve growth factor, and interleukin 18 release by explants of human adipose tissue is by the non-fat cells, not by the adipocytes. Metab-Clin Exp. (2006) 55:1113–21. doi: 10.1016/j.metabol.2006.04.008
140. Landy E, Carol H, Ring A, Canna S. Biological and clinical roles of IL-18 in inflammatory diseases. Nat Rev Rheumatol. (2024) 20:33–47. doi: 10.1038/s41584-023-01053-w
141. Gerdes N, Sukhova GK, Libby P, Reynolds RS, Young JL, Schonbeck U. Expression of interleukin (IL)-18 and functional IL-18 receptor on human vascular endothelial cells, smooth muscle cells, and macrophages: implications for atherogenesis. J Exp Med. (2002) 195:245–57. doi: 10.1084/jem.20011022
142. Lana JP, de Oliveira MC, Silveira ALM, Yamada LTP, Costa KA, da Silva SV, et al. Role of IL-18 in adipose tissue remodeling and metabolic dysfunction. Int J Obes (Lond). (2024) 48:964–72. doi: 10.1038/s41366-024-01507-5
143. Hivert MF, Sun Q, Shrader P, Mantzoros CS, Meigs JB, Hu FB. Circulating IL-18 and the risk of type 2 diabetes in women. Diabetologia. (2009) 52:2101–8. doi: 10.1007/s00125-009-1455-z
144. Mochol M, Taubøll E, Aukrust P, Ueland T, Andreassen OA, Svalheim S. Interleukin 18 (IL-18) and its binding protein (IL-18BP) are increased in patients with epilepsy suggesting low-grade systemic inflammation. Seizure. (2020) 80:221–5. doi: 10.1016/j.seizure.2020.05.018
145. Zhang X, Luo S, Wang M, Cao Q, Zhang Z, Huang Q, et al. Differential IL18 signaling via IL18 receptor and Na-Cl co-transporter discriminating thermogenesis and glucose metabolism regulation. Nat Commun. (2022) 13:7582. doi: 10.1038/s41467-022-35256-8
146. Leick L, Lindegaard B, Stensvold D, Plomgaard P, Saltin B, Pilegaard H. Adipose tissue interleukin-18 mRNA and plasma interleukin-18: effect of obesity and exercise. Obes (Silver Spring). (2007) 15:356–63. doi: 10.1038/oby.2007.528
147. Straczkowski M, Kowalska I, Nikolajuk A, Otziomek E, Adamska A, Karolczuk-Zarachowicz M, et al. Increased serum interleukin-18 concentration is associated with hypoadiponectinemia in obesity, independently of insulin resistance. Int J Obes. (2007) 31:221–5. doi: 10.1038/sj.ijo.0803421
148. Yamanishi K, Maeda S, Kuwahara-Otani S, Watanabe Y, Yoshida M, Ikubo K, et al. Interleukin-18-deficient mice develop dyslipidemia resulting in nonalcoholic fatty liver disease and steatohepatitis. Transl Res. (2016) 173:101–14 e7. doi: 10.1016/j.trsl.2016.03.010
149. Mamane Y, Heylbroeck C, Génin P, Algarté M, Servant MJ, LePage C, et al. Interferon regulatory factors: the next generation. Gene. (1999) 237:1–14. doi: 10.1016/S0378-1119(99)00262-0
150. Taniguchi T, Ogasawara K, Takaoka A, Tanaka N. IRF family of transcription factors as regulators of host defense. Annu Rev Immunol. (2001) 19:623–55. doi: 10.1146/annurev.immunol.19.1.623
151. Janeway CA Jr., Medzhitov R. Innate immune recognition. Annu Rev Immunol. (2002) 20:197–216. doi: 10.1146/annurev.immunol.20.083001.084359
152. Tamura T, Yanai H, Savitsky D, Taniguchi T. The IRF family transcription factors in immunity and oncogenesis. Annu Rev Immunol. (2008) 26:535–84. doi: 10.1146/annurev.immunol.26.021607.090400
153. Mahdi T, Hanzelmann S, Salehi A, Muhammed SJ, Reinbothe TM, Tang YZ, et al. Secreted frizzled-related protein 4 reduces insulin secretion and is overexpressed in type 2 diabetes. Cell Metab. (2012) 16:625–33. doi: 10.1016/j.cmet.2012.10.009
154. Piltonen TT, Chen J, Erikson DW, Spitzer TLB, Barragan F, Rabban JT, et al. Mesenchymal stem/progenitors and other endometrial cell types from women with polycystic ovary syndrome (PCOS) display inflammatory and oncogenic potential. J Clin Endocrinol Metab. (2013) 98:3765–75. doi: 10.1210/jc.2013-1923
155. Garufi G, Seyhan AA, Pasarica M. Elevated secreted frizzled-related protein 4 in obesity: A potential role in adipose tissue dysfunction. Obesity. (2015) 23:24–7. doi: 10.1002/oby.20915
156. Anand K, Vidyasagar S, Lasrado I, Pandey GK, Amutha A, Ranjani H, et al. Secreted frizzled-related protein 4 (SFRP4): A novel biomarker of beta-cell dysfunction and insulin resistance in individuals with prediabetes and type 2 diabetes. Diabetes Care. (2016) 39:E147–E8. doi: 10.2337/dc16-0756
157. Baldane S, Ipekci SH, Kebapcilar AG, Abusoglu A, Beyhekim H, Ilhan TT, et al. Prorenin and secreted frizzled-related protein 4 levels in women with gestational diabetes mellitus. Bratisl Med J. (2018) 119:450–3. doi: 10.4149/BLL_2018_083
158. Guan H, Zheng H, Zhang J, Xiang A, Li Y, Zheng H, et al. Secreted frizzled-related protein 4 promotes brown adipocyte differentiation. Exp Ther Med. (2021) 21:637. doi: 10.3892/etm.2021.10069
159. Almario RU, Karakas SE. Roles of circulating WNT-signaling proteins and WNT-inhibitors in human adiposity, insulin resistance, insulin secretion, and inflammation. Horm Metab Res. (2015) 47:152–7. doi: 10.1055/s-0034-1384521
160. Zhang J, Yang Z, Liang Z, Wang M, Hu C, Chang C, et al. Secreted frizzled-related protein 4 exerts anti-atherosclerotic effects by reducing inflammation and oxidative stress. Eur J Pharmacol. (2022) 923:174901. doi: 10.1016/j.ejphar.2022.174901
161. Voegele AF, Jerkovic L, Wellenzohn B, Eller P, Kronenberg F, Liedl KR, et al. Characterization of the vitamin E-binding properties of human plasma afamin. Biochemistry. (2002) 41:14532–8. doi: 10.1021/bi026513v
162. Jerkovic L, Voegele AF, Chwatal S, Kronenberg F, Radcliffe CM, Wormald MR, et al. Afamin is a novel human vitamin E-binding glycoprotein characterization and in vitro expression. J Proteome Res. (2005) 4:889–99. doi: 10.1021/pr0500105
163. Heiser M, Hutter-Paier B, Jerkovic L, Pfragner R, Windisch M, Becker-Andre M, et al. Vitamin E binding protein afamin protects neuronal cells in vitro. J Neural Transm Suppl. (2002) 62: 337–45. doi: 10.1007/978-3-7091-6139-5_32
164. Gonzalez F, Rote NS, Minium J, Kirwan JP. Reactive oxygen species-induced oxidative stress in the development of insulin resistance and hyperandrogenism in polycystic ovary syndrome. J Clin Endocrinol Metab. (2006) 91:336–40. doi: 10.1210/jc.2005-1696
165. Gonzalez F, Rote NS, Minium J, Kirwan JP. Increased activation of nuclear factor kappa B triggers inflammation and insulin resistance in polycystic ovary syndrome. J Clin Endocrinol Metab. (2006) 91:1508–12. doi: 10.1210/jc.2005-2327
166. Wang M, Li N, Zhang Q, Ma J, Li Q. Acute influence of FK506 on T-lymphocyte populations of peripheral blood and spleen in rats. Transplant Proc. (2007) 39:292–4. doi: 10.1016/j.transproceed.2006.10.213
167. Lumeng CN, Bodzin JL, Saltiel AR. Obesity induces a phenotypic switch in adipose tissue macrophage polarization. J Clin Invest. (2007) 117:175–84. doi: 10.1172/JCI29881
168. Albaghdadi AJH, Feeley CA, Kan FWK. Low-dose tacrolimus prevents dysregulated peri-conceptional ovarian and systemic immune cellular homeostasis in subjects with PCOS. Sci Rep. (2019) 9:6528. doi: 10.1038/s41598-019-42960-x
169. Elnagar HI, Hashem OA, Aboelwafa HO, Elhelw E, Elsaie ML. The impact of oral isotretinoin on ovarian functions of acne patients complaining of polycystic ovarian syndrome: a prospective study. J Ovarian Res. (2024) 17:21. doi: 10.1186/s13048-024-01347-x
170. Kalhori Z, Azadbakht M, Soleimani Mehranjani M, Shariatzadeh MA. Improvement of the folliculogenesis by transplantation of bone marrow mesenchymal stromal cells in mice with induced polycystic ovary syndrome. Cytotherapy. (2018) 20:1445–58. doi: 10.1016/j.jcyt.2018.09.005
171. Xie Q, Xiong X, Xiao N, He K, Chen M, Peng J, et al. Mesenchymal stem cells alleviate DHEA-induced polycystic ovary syndrome (PCOS) by inhibiting inflammation in mice. Stem Cells Int. (2019) 2019:9782373. doi: 10.1155/2019/9782373
172. Chugh RM, Park HS, El Andaloussi A, Elsharoud A, Esfandyari S, Ulin M, et al. Mesenchymal stem cell therapy ameliorates metabolic dysfunction and restores fertility in a PCOS mouse model through interleukin-10. Stem Cell Res Ther. (2021) 12:388. doi: 10.1186/s13287-021-02472-w
173. Mahmoud M, Juntunen M, Adnan A, Kummola L, Junttila IS, Kelloniemi M, et al. Immunomodulatory functions of adipose mesenchymal stromal/stem cell derived from donors with type 2 diabetes and obesity on CD4 T cells. Stem Cells. (2023) 41:505–19. doi: 10.1093/stmcls/sxad021
174. Pham DV, Nguyen TK, Park PH. Adipokines at the crossroads of obesity and mesenchymal stem cell therapy. Exp Mol Med. (2023) 55:313–24. doi: 10.1038/s12276-023-00940-2
175. Szczuko M, Zapałowska-Chwyć M, Drozd A, Maciejewska D, Starczewski A, Wysokiński P, et al. Changes in the IGF-1 and TNF-α synthesis pathways before and after three-month reduction diet with low glicemic index in women with PCOS. Ginekol Pol. (2018) 89:295–303. doi: 10.5603/GP.a2018.0051
176. Liao M, Li X, Zhang H, Zhou L, Shi L, Li W, et al. Effects and plasma proteomic analysis of GLP-1RA versus CPA/EE, in combination with metformin, on overweight PCOS women: a randomized controlled trial. Endocrine. (2024) 83:227–41. doi: 10.1007/s12020-023-03487-4
177. Ryssdal M, Vanky E, Stokkeland LMT, Jarmund AH, Steinkjer B, Løvvik TS, et al. Immunomodulatory effects of metformin treatment in pregnant women with PCOS. J Clin Endocrinol Metab. (2023) 108:e743–e53. doi: 10.1210/clinem/dgad145
178. Ríos JL, Francini F, Schinella GR. Natural products for the treatment of type 2 diabetes mellitus. Planta Med. (2015) 81:975–94. doi: 10.1055/s-0035-1546131
179. Gupta RC, Chang D, Nammi S, Bensoussan A, Bilinski K, Roufogalis BD. Interactions between antidiabetic drugs and herbs: an overview of mechanisms of action and clinical implications. Diabetol Metab Syndr. (2017) 9:59. doi: 10.1186/s13098-017-0254-9
180. Velez LM, Seldin M, Motta AB. Inflammation and reproductive function in women with polycystic ovary syndrome†. Biol Reprod. (2021) 104:1205–17. doi: 10.1093/biolre/ioab050
181. He S, Mao X, Lei H, Dong B, Guo D, Zheng B, et al. Peripheral blood inflammatory-immune cells as a predictor of infertility in women with polycystic ovary syndrome. J Inflammation Res. (2020) 13:441–50. doi: 10.2147/JIR.S260770
182. Aboeldalyl S, James C, Seyam E, Ibrahim EM, Shawki HE, Amer S. The role of chronic inflammation in polycystic ovarian syndrome-A systematic review and meta-analysis. Int J Mol Sci. (2021) 22:2734. doi: 10.3390/ijms22052734
183. Vasyukova E, Zaikova E, Kalinina O, Gorelova I, Pyanova I, Bogatyreva E, et al. Inflammatory and anti-inflammatory parameters in PCOS patients depending on body mass index: A case-control study. Biomedicines. (2023) 11:2791. doi: 10.3390/biomedicines11102791
184. Shi Y, Han T, Cui L, Wu G, Zheng R, Xia M, et al. White blood cell differential counts in patients with polycystic ovary syndrome: a pilot study on Chinese women. Eur J Obstet Gynecol Reprod Biol. (2013) 170:162–4. doi: 10.1016/j.ejogrb.2013.06.002
185. Huang ZH, Manickam B, Ryvkin V, Zhou XJ, Fantuzzi G, Mazzone T, et al. PCOS is associated with increased CD11c expression and crown-like structures in adipose tissue and increased central abdominal fat depots independent of obesity. J Clin Endocrinol Metab. (2013) 98:E17–24. doi: 10.1210/jc.2012-2697
186. Xiao N, He K, Gong F, Xie Q, Peng J, Su X, et al. Altered subsets and activities of B lymphocytes in polycystic ovary syndrome. J Allergy Clin Immunol. (2019) 143:1943–5.e4. doi: 10.1016/j.jaci.2019.01.007
187. Wang T, Xiong X, Xiao N, Yan Y, Liu X, Xie Q, et al. The therapeutic effect of anti-CD19 antibody on DHEA-induced PCOS mice. Int Immunopharmacol. (2024) 130:111711. doi: 10.1016/j.intimp.2024.111711
188. Diamanti-Kandarakis E, Dunaif A. Insulin resistance and the polycystic ovary syndrome revisited: an update on mechanisms and implications. Endocr Rev. (2012) 33:981–1030. doi: 10.1210/er.2011-1034
189. Lewandowski KC, Płusajska J, Horzelski W, Lewiński A. Prevalence of dyslipidaemia and pre-diabetes among women with polycystic ovary syndrome (PCOS): do we overestimate cardiovascular risk? Horm Metab Res. (2019) 51:539–45. doi: 10.1055/a-0896-4144
190. Fang Y, Liu L, Yang Y, Zhang B, Xie S. Causal association between BMI and polycystic ovarian syndrome: Bidirectional two-sample Mendelian randomization study. J Clin Endocrinol Metab. (2024) 110:41–47. doi: 10.1210/clinem/dgae446
191. Stener-Victorin E, Teede H, Norman RJ, Legro R, Goodarzi MO, Dokras A, et al. Polycystic ovary syndrome. Nat Rev Dis Primers. (2024) 10:27. doi: 10.1038/s41572-024-00511-3
192. Liu CC, PeRussia B, Young JD. The emerging role of IL-15 in NK-cell development. Immunol Today. (2000) 21:113–6. doi: 10.1016/S0167-5699(99)01581-9
193. Lin Y, Zhu X, McLntee FL, Xiao H, Zhang J, Fu M, et al. Interferon regulatory factor-1 mediates PPARgamma-induced apoptosis in vascular smooth muscle cells. Arterioscler Thromb Vasc Biol. (2004) 24:257–63. doi: 10.1161/01.ATV.0000109170.43400.2f
194. Caso JR, Pradillo JM, Hurtado O, Lorenzo P, Moro MA, Lizasoain I. Toll-like receptor 4 is involved in brain damage and inflammation after experimental stroke. Circulation. (2007) 115:1599–608. doi: 10.1161/CIRCULATIONAHA.106.603431
195. Li H, Tang QZ, Liu C, Moon M, Chen M, Yan L, et al. Cellular FLICE-inhibitory protein protects against cardiac remodeling induced by angiotensin II in mice. Hypertension. (2010) 56:1109–17. doi: 10.1161/HYPERTENSIONAHA.110.157412
196. Jiang DS, Li L, Huang L, Gong J, Xia H, Liu X, et al. Interferon regulatory factor 1 is required for cardiac remodeling in response to pressure overload. Hypertension. (2014) 64:77–86. doi: 10.1161/HYPERTENSIONAHA.114.03229
197. Liu M, Hummitzsch K, Hartanti MD, Rosario R, Bastian NA, Hatzirodos N, et al. Analysis of expression of candidate genes for polycystic ovary syndrome in adult and fetal human and fetal bovine ovaries†. Biol Reprod. (2020) 103:840–53. doi: 10.1093/biolre/ioaa119
198. Azumah R, Liu M, Hummitzsch K, Bastian NA, Hartanti MD, Irving-Rodgers HF, et al. Candidate genes for polycystic ovary syndrome are regulated by TGFβ in the bovine foetal ovary. Hum Reprod. (2022) 37:1244–54. doi: 10.1093/humrep/deac049
199. Zhang J, Zhang FJ, Zhang L, Xian DX, Wang SA, Peng M, et al. Identification of key genes and molecular pathways in type 2 diabetes mellitus and polycystic ovary syndrome via bioinformatics analyses. Eur Rev Med Pharmacol Sci. (2023) 27:3255–69. doi: 10.26355/eurrev_202304_32097
200. Jia W, Guo Q. Gene structures and promoter characteristics of interferon regulatory factor 1 (IRF-1), IRF-2 and IRF-7 from snakehead Channa argus. Mol Immunol. (2008) 45:2419–28. doi: 10.1016/j.molimm.2007.11.011
201. Klune JR, Dhupar R, Kimura S, Ueki S, Cardinal J, Nakao A, et al. Interferon regulatory factor-2 is protective against hepatic ischemia-reperfusion injury. Am J Physiol Gastrointest Liver Physiol. (2012) 303:G666–73. doi: 10.1152/ajpgi.00050.2012
202. Holland JW, Bird S, Williamson B, Woudstra C, Mustafa A, Wang T, et al. Molecular characterization of IRF3 and IRF7 in rainbow trout, Oncorhynchus mykiss: functional analysis and transcriptional modulation. Mol Immunol. (2008) 46:269–85. doi: 10.1016/j.molimm.2008.08.265
203. Stevens SL, Leung PY, Vartanian KB, Gopalan B, Yang T, Simon RP, et al. Multiple preconditioning paradigms converge on interferon regulatory factor-dependent signaling to promote tolerance to ischemic brain injury. J Neurosci. (2011) 31:8456–63. doi: 10.1523/JNEUROSCI.0821-11.2011
204. Wang XA, Zhang R, She ZG, Zhang XF, Jiang DS, Wang T, et al. Interferon regulatory factor 3 constrains IKKβ/NF-κB signaling to alleviate hepatic steatosis and insulin resistance. Hepatology. (2014) 59:870–85. doi: 10.1002/hep.26751
205. Kumari M, Wang X, Lantier L, Lyubetskaya A, Eguchi J, Kang S, et al. IRF3 promotes adipose inflammation and insulin resistance and represses browning. J Clin Invest. (2016) 126:2839–54. doi: 10.1172/JCI86080
206. Tang P, Virtue S, Goie JYG, Png CW, Guo J, Li Y, et al. Regulation of adipogenic differentiation and adipose tissue inflammation by interferon regulatory factor 3. Cell Death Differ. (2021) 28:3022–35. doi: 10.1038/s41418-021-00798-9
207. Patel SJ, Liu N, Piaker S, Gulko A, Andrade ML, Heyward FD, et al. Hepatic IRF3 fuels dysglycemia in obesity through direct regulation of Ppp2r1b. Sci Transl Med. (2022) 14:eabh3831. doi: 10.1126/scitranslmed.abh3831
208. Pernis AB. The role of IRF-4 in B and T cell activation and differentiation. J Interferon Cytokine Res. (2002) 22:111–20. doi: 10.1089/107999002753452728
209. Schiavoni G, Mattei F, Sestili P, Borghi P, Venditti M, Morse HC 3rd, et al. ICSBP is essential for the development of mouse type I interferon-producing cells and for the generation and activation of CD8alpha(+) dendritic cells. J Exp Med. (2002) 196:1415–25. doi: 10.1084/jem.20021263
210. Kanno Y, Levi BZ, Tamura T, Ozato K. Immune cell-specific amplification of interferon signaling by the IRF-4/8-PU.1 complex. J Interferon Cytokine Res. (2005) 25:770–9. doi: 10.1089/jir.2005.25.770
211. Eguchi J, Kong X, Tenta M, Wang X, Kang S, Rosen ED. Interferon regulatory factor 4 regulates obesity-induced inflammation through regulation of adipose tissue macrophage polarization. Diabetes. (2013) 62:3394–403. doi: 10.2337/db12-1327
212. Yao T, Yan H, Zhu X, Zhang Q, Kong X, Guo S, et al. Obese skeletal muscle-expressed interferon regulatory factor 4 transcriptionally regulates mitochondrial branched-chain aminotransferase reprogramming metabolome. Diabetes. (2022) 71:2256–71. doi: 10.2337/db22-0260
213. Courties G, Heidt T, Sebas M, Iwamoto Y, Jeon D, Truelove J, et al. In vivo silencing of the transcription factor IRF5 reprograms the macrophage phenotype and improves infarct healing. J Am Coll Cardiol. (2014) 63:1556–66. doi: 10.1016/j.jacc.2013.11.023
214. Sindhu S, Kochumon S, Thomas R, Bennakhi A, Al-Mulla F, Ahmad R. Enhanced adipose expression of interferon regulatory factor (IRF)-5 associates with the signatures of metabolic inflammation in diabetic obese patients. Cells. (2020) 9:730. doi: 10.3390/cells9030730
215. Leung PY, Stevens SL, Packard AE, Lessov NS, Yang T, Conrad VK, et al. Toll-like receptor 7 preconditioning induces robust neuroprotection against stroke by a novel type I interferon-mediated mechanism. Stroke. (2012) 43:1383–9. doi: 10.1161/STROKEAHA.111.641522
216. Wang XA, Zhang R, Zhang S, Deng S, Jiang D, Zhong J, et al. Interferon regulatory factor 7 deficiency prevents diet-induced obesity and insulin resistance. Am J Physiol Endocrinol Metab. (2013) 305:E485–95. doi: 10.1152/ajpendo.00505.2012
217. Kuroda M, Nishiguchi M, Ugawa N, Ishikawa E, Kawabata Y, Okamoto S, et al. Interferon regulatory factor 7 mediates obesity-associated MCP-1 transcription. PloS One. (2020) 15:e0233390. doi: 10.1371/journal.pone.0233390
218. Hu M, Zhang Y, Li X, Cui P, Sferruzzi-Perri AN, Brännström M, et al. TLR4-associated IRF-7 and NFκB signaling act as a molecular link between androgen and metformin activities and cytokine synthesis in the PCOS endometrium. J Clin Endocrinol Metab. (2021) 106:1022–40. doi: 10.1210/clinem/dgaa951
Keywords: polycystic ovary syndrome, insulin resistance, immune molecules, immune cells, endocrine disorder
Citation: Zhang Q, Yang Z, Ou X, Zhang M, Qin X and Wu G (2025) The role of immunity in insulin resistance in patients with polycystic ovary syndrome. Front. Endocrinol. 15:1464561. doi: 10.3389/fendo.2024.1464561
Received: 14 July 2024; Accepted: 31 December 2024;
Published: 22 January 2025.
Edited by:
Claus Yding Andersen, University of Copenhagen, DenmarkReviewed by:
Krzysztof Cezary Lewandowski, Medical University of Lodz, PolandCopyright © 2025 Zhang, Yang, Ou, Zhang, Qin and Wu. This is an open-access article distributed under the terms of the Creative Commons Attribution License (CC BY). The use, distribution or reproduction in other forums is permitted, provided the original author(s) and the copyright owner(s) are credited and that the original publication in this journal is cited, in accordance with accepted academic practice. No use, distribution or reproduction is permitted which does not comply with these terms.
*Correspondence: Gengxiang Wu, eHB3dWdlbmd4aWFuZ0AxMjYuY29t
Disclaimer: All claims expressed in this article are solely those of the authors and do not necessarily represent those of their affiliated organizations, or those of the publisher, the editors and the reviewers. Any product that may be evaluated in this article or claim that may be made by its manufacturer is not guaranteed or endorsed by the publisher.
Research integrity at Frontiers
Learn more about the work of our research integrity team to safeguard the quality of each article we publish.