- 1Department of Metabolism and Endocrinology, the First Affiliated Hospital, Hengyang Medical School, University of South China, Hengyang, Hunan, China
- 2Department of Metabolism and Endocrinology, Shenzhen Nanshan People's Hospital; The Sixth Affiliated Hospital of Shenzhen University Health Science Center, Shenzhen, Guangdong, China
- 3Department of Urology, Shenzhen Nanshan People's Hospital; The Sixth Affiliated Hospital of Shenzhen University Health Science Center, Shenzhen, Guangdong, China
- 4Department of pediatrics, Shenzhen Nanshan People's Hospital; The Sixth Affiliated Hospital of Shenzhen University Health Science Center, Shenzhen, Guangdong, China
- 5Department of Emergency, the First Affiliated Hospital, Hengyang Medical School, University of South China, Hengyang, Hunan, China
Incretin receptor agonists (IRAs), primarily composed of glucagon-like peptide-1 receptor agonists (GLP-1RAs) and glucose-dependent insulinotropic polypeptide receptor agonists (GIPRAs), work by mimicking the actions of the endogenous incretin hormones in the body. GLP-1RAs have been approved for use as monotherapy and in combination with GIPRAs for the management of type 2 diabetes mellitus (T2DM). In addition to their role in glucose regulation, IRAs have demonstrated various benefits such as cardiovascular protection, obesity management, and regulation of bone turnover. Some studies have suggested that IRAs not only aid in glycemic control but also exhibit anti-atherosclerotic effects. These agents have been shown to modulate lipid abnormalities, reduce blood pressure, and preserve the structural and functional integrity of the endothelium. Furthermore, IRAs have the ability to mitigate inflammation by inhibiting macrophage activation and promoting M2 polarization. Research has also indicated that IRAs can decrease macrophage foam cell formation and prevent vascular smooth muscle cell (VSMC) phenotype switching, which are pivotal in atheromatous plaque formation and stability. This review offers a comprehensive overview of the protective effects of IRAs in atherosclerotic disease, with a focus on their impact on atherogenesis.
1 Introduction
The increasing global prevalence of diabetes is a significant health concern (1). Individuals with T2DM often experience elevated blood sugar levels and lipid abnormalities, leading to vascular damage and an increased risk of cardiovascular (CV) diseases (2). Vascular complications related to diabetes are the primary cause of death in diabetic patients, with atherosclerotic cardiovascular disease (CVD) accounting for 75% of fatalities (3). Therefore, achieving glycemic control and positive CV outcome are essential objectives in the treatment of diabetes (4). In recent years, a novel class of diabetes medications called incretin receptor agonists (IRAs) has emerged. These agents bind to GLP-1 or GIP receptors, mimicking the action of natural incretin (5). During hyperglycemic conditions, they promote insulin secretion in a glucose-dependent manner and reduce glucagon secretion (6). Moreover, they slow down nutrient absorption by delaying gastric emptying, extending the feeling of fullness, and limiting food intake. The antihyperglycemic effect of incretin play a role in maintaining well-regulated glucose levels. Interestingly, there have been indications that incretin may possess vasculoprotective properties beyond its effects on glycemic control (7).
Recent basic and clinical studies have highlighted the protective effects of IRAs in atherogenesis (8–10). IRAs help in managing atherosclerotic risk factors by improving blood glucose, blood lipids, and reducing blood pressure (11, 12). GLP-1 receptors (GLP-1R) and GIP receptors (GIPR) are expressed in various cardiovascular cell types, including endothelial cells, monocytes/macrophages, vascular smooth muscle cells (VSMCs), and cardiomyocytes (13). The activation of GLP-1R and/or GIPR by incretins can protect against endothelial dysfunction, reduce intravascular inflammation mediated by macrophages, and decrease VSMCs proliferation (14, 15). Specifically, GLP-1R agonists (GLP-1RAs) like liraglutide and exenatide have shown significant clinical success in managing diabetes and its associated atherosclerotic complications (16). Other classes of IRAs, such as GIPR agonists (GIPRAs) like GIP[3-30]NH2, as well as dual GIPR and GLP-1R co-agonists (GIP/GLP-1RAs) like tirzepatide, have also gained attention for their effectiveness in treating diabetes and its atherosclerotic complications (17, 18). These findings suggest that incretin hormones may play a role in preventing atherosclerosis. In this review, we summarize the protective effects of incretin hormones on atherogenesis, providing insights into their clinical benefits and the underlying mechanisms involved.
2 Clinical effects of IRAs on cardiovascular disease
Currently, clinical researchers are primarily investigating three major classes of IRAs: GLP-1RAs, GIP-RAs, and GIP/GLP-1RAs (19). Among these, GLP-1RAs and GIP/GLP-1-RAs have shown favorable CV outcomes in patients with T2DM and are already being used in clinical practice (20). In addition to their positive effects on glucose levels, certain GLP-1RAs such as liraglutide, semaglutide, and dulaglutide have demonstrated vasoprotective effects independent of their glucose-lowering properties (21–23). These agents also have the potential to improve atherosclerotic risk factors and reduce the incidence of CV events.
2.1 Effect of IRAs on risk factors of atherosclerosis-related diseases
In addition to their effectiveness in reducing blood glucose levels, IRAs have also shown positive impacts on blood lipid profiles and blood pressure, ultimately contributing to improved CV health (Table 1).
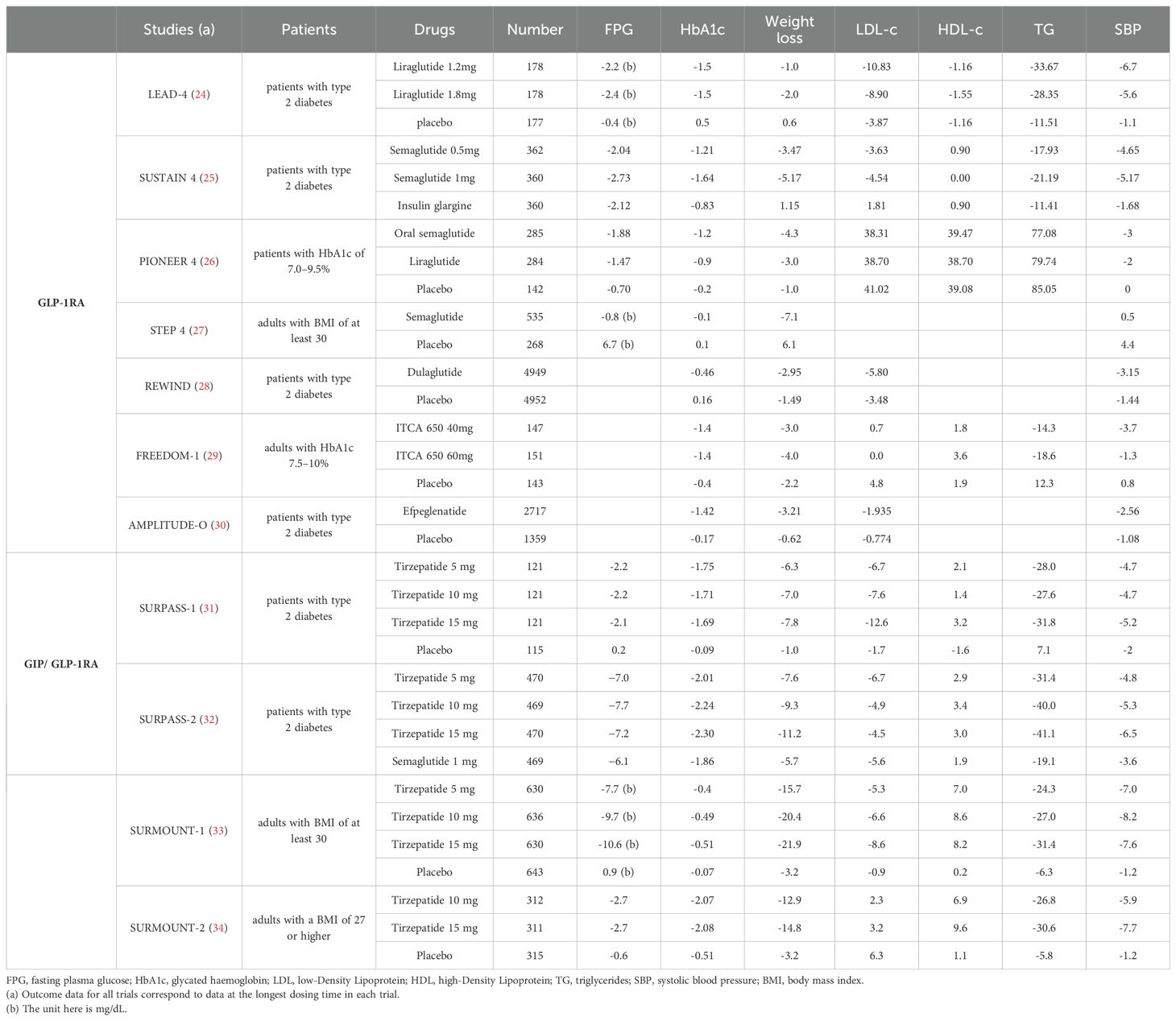
Table 1. Effects of incretin receptor agonists on changes from baseline FPG (mmol/L), HbA1c (%), body weight (kg),LDL-c (mg/dL), HDL-c (mg/dL),TG (mg/dL) and SBP (mmHg) in patients with T2DM: findings from randomized controlled trials.
Dyslipidemia, characterized by disruptions in lipoprotein levels, plays a significantly role in atherosclerosis-related conditions (35). Studies have consistently emphasized the ability of GLP-1RAs, such as exenatide, liraglutide, and semaglutide, not only to decrease fasting total cholesterol, triglycerides (TG), and low-density lipoprotein cholesterol (LDL-c) levels but also to moderately increase high-density lipoprotein cholesterol (HDL-c) levels (36). Additionally, these medications have been found to reduce postprandial plasma TG and celiac microparticle apolipoprotein β-48 levels in both non-diabetic individuals and those with T2DM (37). Regarding GIP, a notable study has shown that GIP infusion can lower circulating nonesterified fatty acids and increase TG levels, potentially aiding in preventing ectopic lipid accumulation and promoting fat storage in adipose tissue (38). Moreover, recent phase 2 clinical trials investigating the potential of tizepatide, a GIP/GLP-1RAs, have produced promising results for patients with T2DM. Known as the SURPASS research series, these trials have demonstrated the effectiveness of tizepatide in reducing TG levels, LDL-c levels, and very low-density lipoprotein cholesterol (VLDL-c) levels, while also significantly raising HDL-c levels (39). Overall, these findings underscore the potential of incretin receptor agonists in improving lipid metabolism.
Hypertension, a well-established risk factor for atherosclerosis, has been extensively studied in relation to IRAs (40). Clinical trials have shown that GLP-1RAs effectively reduce systolic blood pressure (SBP) in hypertensive patients with T2DM. However, the impact on diastolic blood pressure seems to be less significant (36, 41, 42). The infusion of GIP has shown potential in lowering mean arterial blood pressure in patients with impaired glucose tolerance or T2DM (43). Intriguingly, tirzepatide has exhibited a favorable effect on blood pressure, leading to an average decrease in SBP ranging from -6.1 to -12.6 mmHg in treated patients (44). These results emphasize the advantages of IRAs, not only in controlling blood sugar levels but also in addressing other CV risk factors.
2.2 Effect of IRAs on cardiovascular event outcomes
Intensive glucose lowering in patients with T2DM has been shown to reduce microvascular complications, although its impact on CV events or mortality remains a topic of debate (45). Numerous clinical trials have been conducted to evaluate the cardiovascular outcomes of T2DM therapy, focusing on major adverse cardiac events (MACE) such as CV death, non-fatal myocardial infarction (MI), and nonfatal stroke. Long-term multinational and multicenter CV outcome trials (CVOTs) have generated substantial evidence supporting the use of GLP-1RAs in effectively lowering the risk of CV events (Table 2).
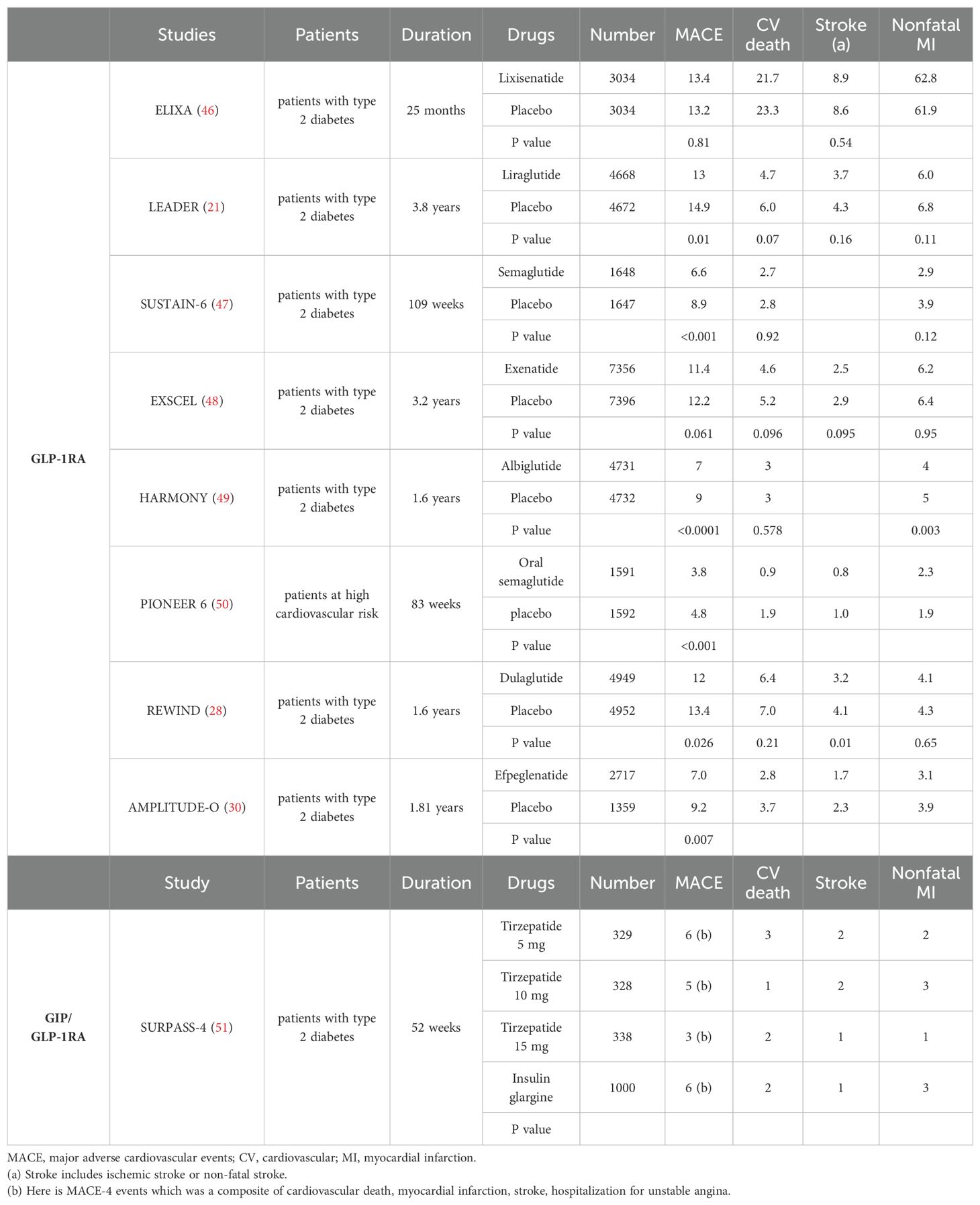
Table 2. The clinical cardiovascular events occurrence (%) of incretin receptor agonists, results from major randomized controlled trials.
The ELIXA trial assessed lixisenatide as a treatment for individuals with T2DM and acute coronary syndromes, finding that it had a similar 3-point MACE rate to placebo (46). Subsequent on CVOT research on liraglutide, semaglutide and dulaglutide showed positive results on 3-point MACE (28, 47). For instance, the LEADER trial indicated that liraglutide decreased MACE occurrence by 13%, CV mortality by 22%, and all-cause mortality by 15% in T2DM and CVD patients compared to placebo (21). Similarly, the SUSTAIN-6 study revealed a significant 26% risk reduction in the primary composite outcome (including CV death, nonfatal MI, or nonfatal stroke) for semaglutide-treated patients versus placebo. Furthermore, emerging evidence suggests that GLP-1RAs like semaglutide not only have cardioprotective effects but also potential benefits in reducing heart failure risk and preserving renal function (47, 50). In the REWIND study, dulaglutide reduced nonfatal stroke by 24% over 5 years in T2DM patients compared to placebo (28). Individuals with peripheral artery disease often have elevated levels of circulating GIP, a vascular protective peptide known to delay atherosclerosis progression (52). Tizepatide, another promising drug, has shown sustained glycemic and weight benefits over 52 weeks in high-risk diabetic patients without increasing MACE-4 risk (51). Ongoing research is investigating the specific cardiovascular effects of tizepatide, with the SURPASS-CVOT trial (ClinicalTrials.gov Identifier: NCT04255433) showing promise.
3 Mechanisms of the protective effect of IRAs on atherosclerosis
Atherosclerosis is a progressive disease that mainly affects large and medium-sized arteries (53). Factors such as hypertension, smoking, hyperlipidaemia, diabetes, and inflammation contribute to the development of atherosclerosis by increasing the permeability of blood vessels to LDL-c, commonly known as 'bad' cholesterol (54–56). Oxidized LDL (ox-LDL) plays a key role in this process by attracting monocytes into the inner layer of the artery wall, where they transform into pro-inflammatory macrophages. These macrophages engulf ox-LDL, forming foam cells and triggering inflammatory responses (57). Historically, foam cells were believed to originate mainly from monocyte-derived macrophages in early atherosclerotic lesions (58). However, smooth muscle cells can also transform into foam cells in atherosclerosis. In cases of chronic inflammation, smooth muscle cells can degrade the collagen fibrous cap of the plaque, making it unstable and prone to complications such as thrombosis, heart attack, and stroke (59).
Clinical and basic research studies have provided valuable insights into the effects of IRAs on risk factors associated with atherosclerosis and the intracellular pathways in key cell types involved in the disease process (12, 14). The findings indicate that IRAs may offer therapeutic benefits in alleviating atherosclerotic disease.
3.1 Mechanisms underlying the reduction of risk factors for atherosclerosis-related diseases
3.1.1 Improving blood lipid disorders
Lipid metabolism disorders are closely linked to atherosclerosis development, and clinical studies demonstrate that lipid lowering therapy significantly enhances CV outcomes (60). IRAs have been shown to reduce TG and LDL-c levels while increasing HDL-c levels (37). GLP-1RA reduces TG production and release in the liver and adipose tissues by inhibiting lipid synthesis, promoting weight loss and improving insulin sensitivity (61). It also reduces plasma levels of oxidized LDL cholesterol and stimulates genes involved in cholesterol efflux, preventing the formation of cholesterol-rich plaques in blood vessels (62).
As for GLP-RAs, such as Exendin-4 and Semaglutide, have similar effects on lipid metabolism, Exendin-4 can increase sympathetic nerve tension, leading to inhibition of liver and intestinal lipoprotein production (63). Semaglutide can decrease the production of β-48 apolipoprotein lipoprotein particles, resulting in decreased chylomicron formation and release. The weight loss and improved insulin resistance induced by semaglutide enhance the antilipolytic effects of insulin in adipose tissue, ultimately leading to a decrease in circulating free fatty acids. This overall process results in reduced synthesis and secretion of chylomicrons and VLDLs (64). Activation of GLP-1RAs can also stimulate brown adipose tissue (BAT) activation, which generates heat through thermogenesis (65). Exendin-4 can enhance BAT activation and promote thermogenesis, providing benifits for weight loss and lipid control (65).
GIP regulates lipid metabolism by affecting both lipogenesis and lipolysis (66). In the presence of insulin, GIP activates protein kinase B by increasing cAMP levels, leading to the phosphorylation of lipoprotein lipase (LPL) and promoting adipogenesis (67). GIP also increase the expression of LPL and GLUT4 by activating cAMP response element-binding protein (CREB) and PI3K (68). Conversely, in the absence of insulin, GIP-induced protein kinase A (PKA) activation stimulates hormone-sensitive lipase activity, promoting lipolysis (66). Therefore, the role of GIP in lipid metabolism has been a topic of controversial.
3.1.2 Lowering blood pressure
Hypertension occurs in more than 50% of T2DM patients and contributes to the development of both micro- and macro-vascular diseases (69). Studies have investigated the mechanisms by which GLP-1RAs impact blood pressure, revealing that they reduces sodium retention by inhibiting sodium ion reabsorption in the kidney's proximal tubules (70). Additionally, an acute renal infusion of GLP-1 was found to increase renal blood flow and promote urinary sodium excretion, resulting in a hypotensive effect (71). Furthermore, acute renal infusion of GLP-1 has been observed to enhance renal blood flow, promote urinary sodium excretion, and induce a hypotensive effect. These findings suggest that GLP-1RAs treatment may offer additional benefits for managing blood pressure (72). Further research have shown that Liraglutide treatment can induce renal vasodilation, diminish renal autoregulatory responses in hypertensive rats, and alleviate angiotensin II (Ang II)-induced blood pressure elevation (73). These findings suggest that GLP-1RAs may reduce blood pressure by decreasing sodium reabsorption, promoting vasodilation, and mitigating intrarenal Renin-Angiotensin System activation.
The effect and mechanism of GIP on blood pressure is unclear. Infusion of GIP has been shown to decrease mean arterial blood pressure (43). A study involving non-obese young men revealed that GIP infusion during hyperglycemia increased blood flow in the femoral artery, but no changes were observed during normoglycemia (74). Interestingly, GIP has been found to stimulate the release of nitric oxide (NO) from portal vein endothelial cells and human umbilical vein endothelial cells, leading to vasodilation (75, 76). This suggests that GIP may have the ability to lower blood pressure by dilating blood vessels. The observed reduction in systolic blood pressure and increase in arterial blood flow following GIP infusion may provide potential cardiovascular protection.
3.2 Maintenance of the integrity and stability of endothelial function
The endothelium, located as the innermost layer of blood vessels, acts as a protective barrier between the bloodstream and surrounding tissue (77). Prolonged exposure of endothelial cells to factors like ox-LDL, nicotine, cholesterol crystals, and inflammatory stimuli leads to endothelial dysfunction (78). This dysfunction is manifests as compromised vasodilation, reduced NO secretion, increased oxidative stress, enhanced leukocyte adhesion, and higher permeability. Consequently, the weakened barrier function allows infiltration of lipoproteins, immune cells, and platelets into the arterial wall, resulting in the formation of lipid-rich plaques known as atherosclerotic lesions (56, 79). Addressing endothelial dysfunction and restoring normal function may prevent or alleviate the development of CVD. IRAs have been demonstrated to offer significant protection for the endothelium through specific mechanisms as follows (Figure 1).
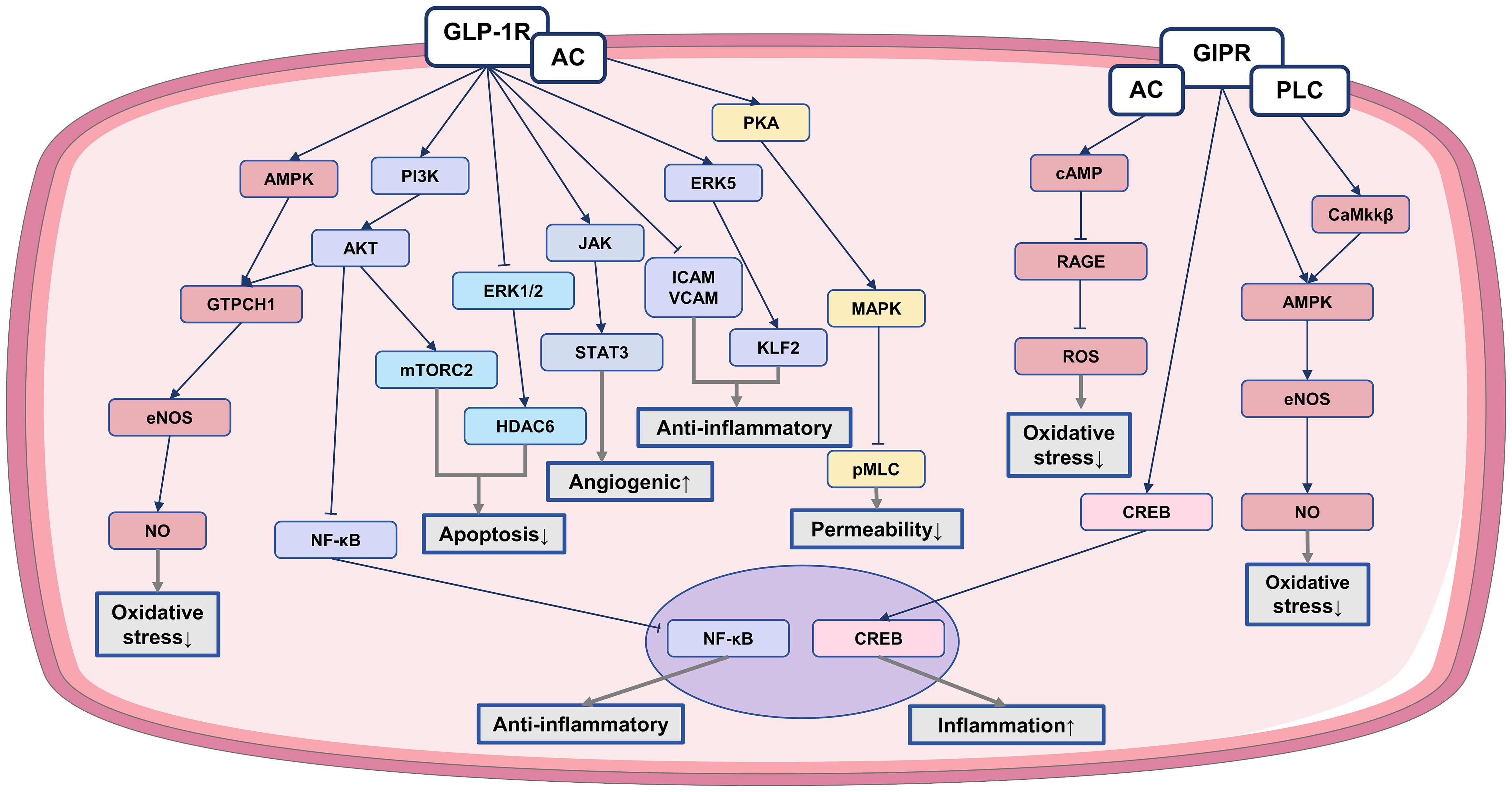
Figure 1. The anti-atherosclerotic effect of IRAs on endothelial cells. Protective effects of IRAs in atherosclerosis by ameliorating endothelial cell dysfunction. ↓, reduction; ↑, increase; AC, adenylate cyclase; PLC, phospholipase C; ATP, adenosine triphosphate; pMLC, phosphorylated myosin light chain; GTPCH1, GTP cyclohydrolase I; ERK5, extracellular signal-regulated kinase 5; CaMKKβ, calcium/calmodulin-dependent protein kinase kinase β.
3.2.1 Promoting endothelial diastole and reducing oxidative stress
GLP-1RAs protect endothelial cell function through various mechanisms. For example, liraglutide activates AMP-activated protein kinase (AMPK) and PI3K/ Akt pathways, leading to increased guanosine triphosphate cyclohydrolase-1 (GTPCH1) levels. This elevation enhances endothelial NO synthase (eNOS) coupling, enhancing NO production (80). Additionally, liraglutide reduce s-glutathionylation, a marker of eNOS dysfunction, and enhances NO bioavailability. Fruthermore, it upgrates GLP-1R expression, inhibiting the activation of inhibitor of nuclear factor kappa B alpha (IκBα) and nuclear factor kappa B (NF-κB), thereby suppressing endothelin-1 (ET-1) expression and enhances eNOS expression (81). Liraglutide also inhibits protein kinase C-alpha and nicotinamide adenine dinucleotide phosphate (NADPH) oxidase, while increasing the upregulation of antioxidant enzymes. Similar functions have been observed with other GLP-1 analog, such as liraglutide and exendin-4. Overall, GLP-1RAs stimulate NO production and eNOS activation, facilitating arterial endothelial vasorelaxation (82–84). These effects help mitigate oxidative stress-induced damage to endothelial cell function.
GIP has also been shown to enhance NO production in endothelial cells (85). The activation of GIP significantly increases the phosphorylation levels of AMPK and eNOS, ultimately activating eNOS and increasing NO production, promoting the stretching of vascular endothelial cells (86). GIP also inhibits oxidative stress caused by advanced glycation end products (AGEs) through the GIPR-cAMP axis, reducing reactive oxygen species (ROS) generation and disrupting AGEs signaling within the vascular endothelium. This process helps in alleviating oxidative stress in endothelial cells (87). However, it is noteworthy that GIP has been observed to elevate CREB phosphorylation in endothelial cells, leading to endothelin-1 (ET-1) production (88). ET-1, acting as an angiotensin, regulates vasoconstriction, with elevated levels linked to atherosclerosis progression (89). Further research and investigation are necessary to fully understand the impact of GIP on endothelial cells.
3.2.2 Reducing endothelial cell inflammation
The endothelial inflammatory response leads to increased adhesion and migration of monocytes to the subendothelial space, initiating the development of atherosclerosis (78). Both liraglutide and dulaglutide inhibit the down-regulation of the transcription factor krüppel-like factor 2 (KLF2), reducing the expression of vascular adhesion molecules induced by ox-LDL. This inhibition prevents monocyte rolling and adhesion to endothelial cells (90). Additionally, GLP-1 promotes the secretion of lipocalin through the structural domain of the adaptor protein APPL1, exerting anti-inflammatory effects (91). GLP-1RAs also decrease the expression of adhesion molecules ICAM and VCAM in endothelial cell, inhibiting NF-κB phosphorylation (92). By modulating these pathways, GLP-1RAs effectively reduce endothelial cell inflammation and improve endothelial dysfunction, ultimately decrease leukocyte-endothelial interactions and inflammation (93).
3.2.3 Decreasing endothelial cell permeability
GLP-1RAs decrease endothelial cell permeability through the activation of the cAMP/PKA signaling pathway, which in turn influences the Rho/Rho kinase (ROCK) and MAPK signaling pathways. This cascade leads to a decrease in myosin light chain (MLC) phosphorylation and dephosphorylation, promoting improved cytoskeletal reorganization and ultimately reducing endothelial cell permeability (94). Studies have shown that liraglutide can reverse ox-LDL-induced decline in the endothelial tight junction and counteract the ox-LDL-induced increase in endothelial monolayer permeability, thereby reducing lipid deposition under the endothelium (90).
3.2.4 Regulating endothelial cell apoptosis and proliferation
The regeneration of endothelial cells post vascular injury is essential for maintaining vascular health. The balance between cell apoptosis and proliferation is crucial for this process (95).
GLP-1RAs increase the expression of the anti-apoptotic protein b-cell lymphoma 2 (Bcl-2) while reducing the expression of the pro-apoptotic protein Bcl-2-associated X protein (Bax) (96). GLP-1RAs also prevent endothelial cell apoptosis by disrupting the mitochondria-cytochrome c-cysteinase protease pathway triggered by AGEs (97). Moreover, GLP-1 can restore histone deacetylase 6 (HDAC6) expression by decreasing extracellular signal-regulated kinase 1/2 (ERK1/2) phosphorylation, thus reducing endothelial cell apoptosis (96). Liraglutide has been shown to have anti-apoptotic effects by enhancing the Bcl-2/Bax protein ratio through mechanistic target of rapamycin complex 2 (mTORC2)-Akt activation (98).
In addition to inhibit apoptosis, IRAs also play a crucial role in promoting endothelial cell proliferation. Exendin-4 boosts the expression of angiogenic genes, such as Ang1 and its receptor Tie-2 (TEK), which are vital for angiogenesis and endothelial cell survival (99). Liraglutide enhances the angiogenic potential of HUVECs by activating the janus kinase 2 (JAK2)/ signal transducer and activator of transcription-3 (STAT-3) pathway, leading to increased expression of factors like vascular endothelial growth factor (VEGF), basic fibroblast growth factor (bFGF), and eNOS (100). GIP shows the ability to stimulate endothelial cell mitosis, supporting the proliferation of HUVECs and ECV-304 cells for repairing damaged endothelial cells (101).
Collectively, GLP-1RAs possess various mechanisms that contribute to their protective effects on endothelial cells by regulating vasoactive factors, reducing oxidative stress and inflammation, improving permeability, and inhibiting apoptosis. These medications show promise in preserving endothelial function and reducing cardiovascular complications in patients with T2DM. Similarly, GIPRAs have also been shown to have a protective effect on endothelial cells. However, further research is necessary to fully understand the impact of GIP in improving vascular endothelial cell dysfunction.
3.3 Inhibition of foam cells formation and alleviation of inflammatory response in plaque
Macrophages engulf ox-LDL within the arterial wall, leading to the formation of cholesterol-laden foam cells, which are intergral to the development of atherosclerosis (102). Upon activation, macrophages can undergo phenotypic transformation and release cytokines and adhesion molecules, like ICAM and VCAM onto the endothelial surface of the artery. This process cause circulating immune cells adhering to the endothelial layer, migrating into the intima, and ultimately contributing to the growth of a plaque within the arterial wall (103). Therefore, macrophages play a key role in the pathogenesis of atherosclerosis (104). Interestingly, research has found that IRAs demonstrate inhibitory effects on foam cell formation and alleviate inflammatory responses within plaques.
3.3.1 Reducing foam cell formation
Liraglutide has been identified as a potential solution for promoting cholesterol excretion in macrophages by up-regulating ATP-binding cassette transporter A1 (ABCA1) in monocytes/macrophages, effectively reducing foam cell formation (105). It also has been observed to inhibit macrophage uptake of ox-LDL through the PKA/cluster of differentiation 36 (CD36) pathway (106). GLP-1 application was found to significantly inhibit ox-LDL-induced foam cell formation, primarily through down-regulation of acylcoenzyme A: cholesterol acyltransferase-1 (ACAT-1) in human monocyte-derived macrophages (107).
Similarly, GIP was observed to decrease the expression of CD36 and ACAT-1, hinder the absorption of ox-LDL, and reduce the formation of foam cells (107). Additionally, GIP infusion was shown to successfully decrease foam cell formation by stimulating GIP receptors and subsequently blocking the cyclin-dependent kinase 5 (Cdk 5)-CD36 pathway (108).
3.3.2 Inhibiting macrophage activation and attenuates inflammation
Exendin-4 demonstrates the ability to activate the cAMP/PKA pathway in macrophages, resulting in the inhibition of inflammatory responses, decrease levels of tumor necrosis factor alpha (TNF-α) and monocyte chemoattractant protein-1 (MCP-1), and reduced accumulation of monocytes/macrophages in the vascular intima (93).
Studies on ApoE-deficient and GIP receptor (ApoE-/-:Gipr-/-) knockout mice revealed an increase in the mRNA levels of Cxcl1 and Arg1 transcripts, indicating a potential increase in vascular inflammation. The findings suggest that GIP receptor activation could play a role in reducing vascular inflammation (109). While preclinical data support the beneficial impact of GIP receptor activation on vascular inflammation in mice, further studies are needed to elucidate the specific pathways involved.
3.3.3 Facilitating macrophage polarization to M2 phenotype
GLP-1R activation promotes macrophages polarization towards the M2 phenotype, known for its anti-inflammatory properties and role in tissue repair (110). Lixisenatide has been shown to activate STAT-3, essential for M2 macrophage differentiation, while downregulating STAT-1, a regulator of the M1 phenotype. Furthermore, lixisenatide treatment reduces inducible NO synthase levels in M1 macrophages and increases arginase I expression in M2 macrophages (111). Liraglutide also enhances M2 phenotypes, which have atheroprotective effects, by influencing the balance between M1 and M2 macrophages (112). These findings highlight the potential of GLP-1RAs in modulating macrophage polarization and fostering an anti-inflammatory milieu.
These research overall shows that GLP-1RA and GIP have the ability to decrease foam cell formation and inhibit inflammatory responses, suggesting their potential for anti-atherosclerotic benefits (Figure 2).
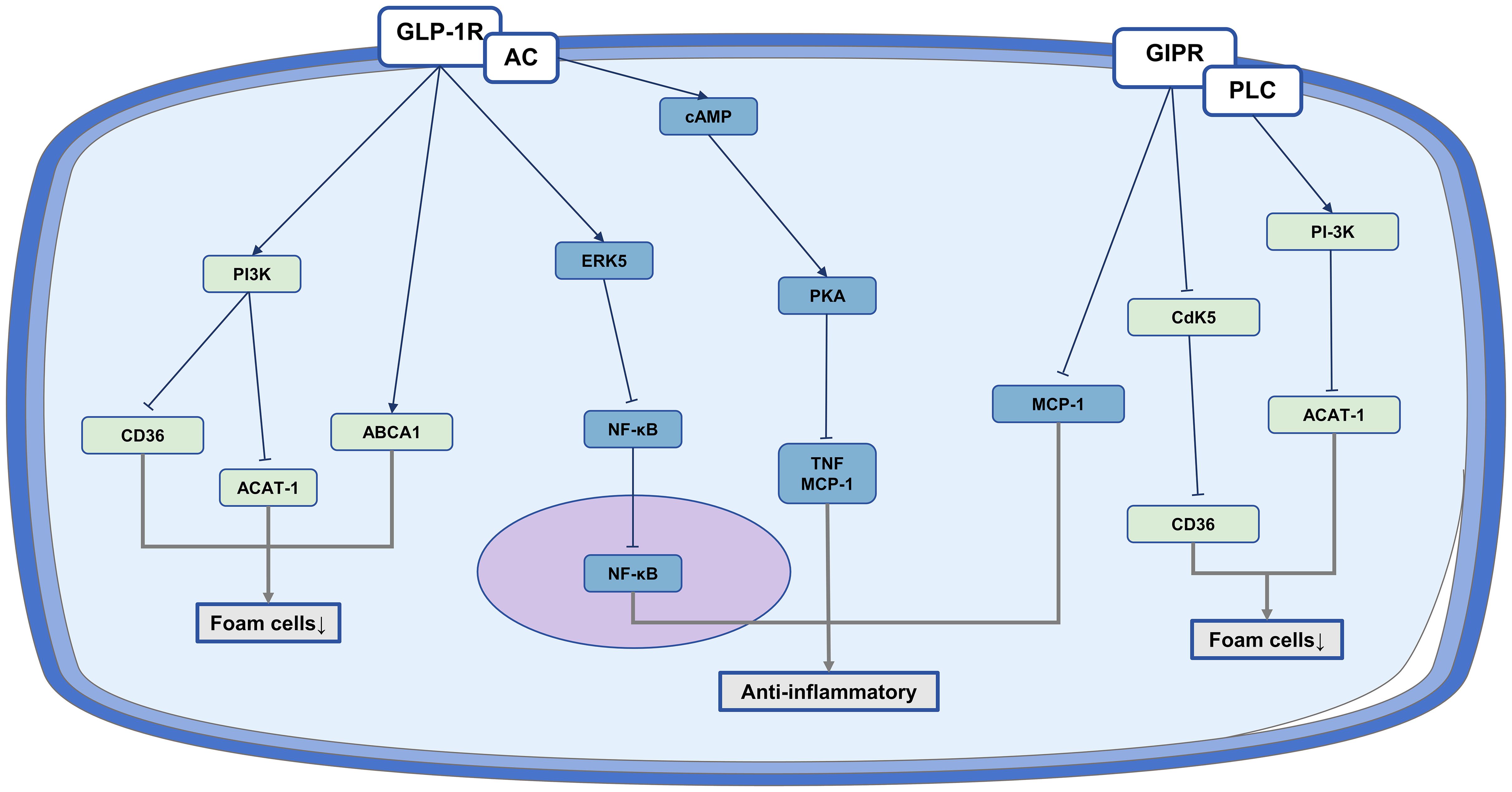
Figure 2. The anti-atherosclerotic effect of IRAs on monocyte/macrophages. Protective role of IRAs in atherosclerosis by reducing foam cell formation and inflammation production.
3.4 Modulation of VSMCs proliferation, senescence, apoptosis, and phenotypic transition
In the pathogenesis of atherosclerosis, VSMCs demonstrate increased proliferation and migration capabilities, shifting from a contraction phenotype to a synthetic phenotype (113). These changes contribute to the thickening of arterial wall, accompanied by the release of various extracellular matrix (ECM) proteins and cytokines. Consequently, these processes induce inflammation and worsen the advancement of atherosclerosis (114). Additionally, disruptions in autophagy, apoptosis, and aging in VSMCs can result in dysfunction and increased vulnerability of blood vessels to injury (115). Intriguingly, treatment with IRAs shows testamental cardiovascular protective effects by modulating VSMCs proliferation, senescence, apoptosis, and phenotypic transition.
3.4.1 Suppressing the proliferation and migration of VSMCs
GLP-1RA treatment is associated with a reduction in intimal thickening after vascular injury, potentially due to decreased proliferation and migration of VSMCs (116). Liraglutide inhibit the ERK1/2 and PI3K/Akt pathways, which helps in reducing abnormal proliferation and migration of VSMCs induced by hyperglycemia (117). Exendin-4 has demonstrated the ability to prevent Ang II-induced VSMC proliferation by inhibiting the phosphorylation of ERK1/2 and JNK, key players in VSMC proliferation and migration (118). Liraglutide is found to inhibit homocysteine-induced proliferation, migration, and phenotypic switching of VSMCs by suppressing PCSK9/LDL receptor (119).
3.4.2 Inhibiting phenotypic transformation of VSMCs
Liraglutide has been shown to stimulate mitochondrial fusion via the PKA/ dynamin-related protein 1 (Drp1) signaling pathway, leading to increased mitochondrial activity and decreased platelet-derived growth factor BB (PDGF-bb)-induced dedifferentiation of VSMCs (120). It also suppresses the expression of osteoblast differentiation markers, consequently reducing osteoblast differentiation and calcification of human VSMCs by activating the PI3K/Akt/mTOR/ ribosomal protein S6 kinase beta-1 (S6K1) signaling pathway (121). Moreover, liraglutide inhibits age-induced phenotypic transformation of VSMCs by downregulating cardiac myosin, inhibiting the NF-κB pathway, activating the PKA signaling pathway, upregulating the expression of contractile markers in VSMCs, and decreasing collagen production (122). Treatment with Exendin-4 promotes a more typical shuttle shape and increases the protein levels of contractile VSMC markers such as calreticulin and smooth muscle 22-alpha (SM22α). Exendin-4 inhibits VSMC phenotypic transformation by activating the AMPK/sirtuin 1 (SIRT1)/ forkhead box O3a (FOXO3a) signaling pathway (123).
3.4.3 Inhibiting VSMCs senescence and apoptosis
Exendin-4 exerts a protective effect by inhibiting ras-related C3 botulinum toxin substrate 1 (Rac1) activation in a GLP-1R/cAMP/PKA-dependent manner, leading to a reduction in NADPH oxidase 1 (Nox1)-generated ROS levels and subsequently decreasing superoxide-induced VSMC senescence (124). Exendin-4 can also suppresse Ang II-induced premature senescence of VSMCs by promoting the recruitment of CREB-binding protein (CBP) to nuclear factor erythroid 2-related factor 2 (Nrf2) via PKA, resulting in enhanced Nrf2 acetylation that inhibits superoxide production and contributes to senescence inhibition in VSMCs (125). Liraglutide pretreatment has been shown to decrease high glucose-induced apoptosis in VSMCs by modulating levels of cleaved caspase-3, Bax, and Bcl-2. These effects are mediated through GLP-1R activation, which inhibits high glucose-induced phosphorylation of ERK1/2 and Akt, ultimately reducing apoptosis (117).
In short, GLP-1RAs show promise in inhibiting the abnormal proliferation and migration of VSMCs, as well as in preventing their phenotypic transformation. Furthermore, they can impede apoptosis and senescence of VSMCs, thus slowing down the progression of atherosclerosis (Figure 3).
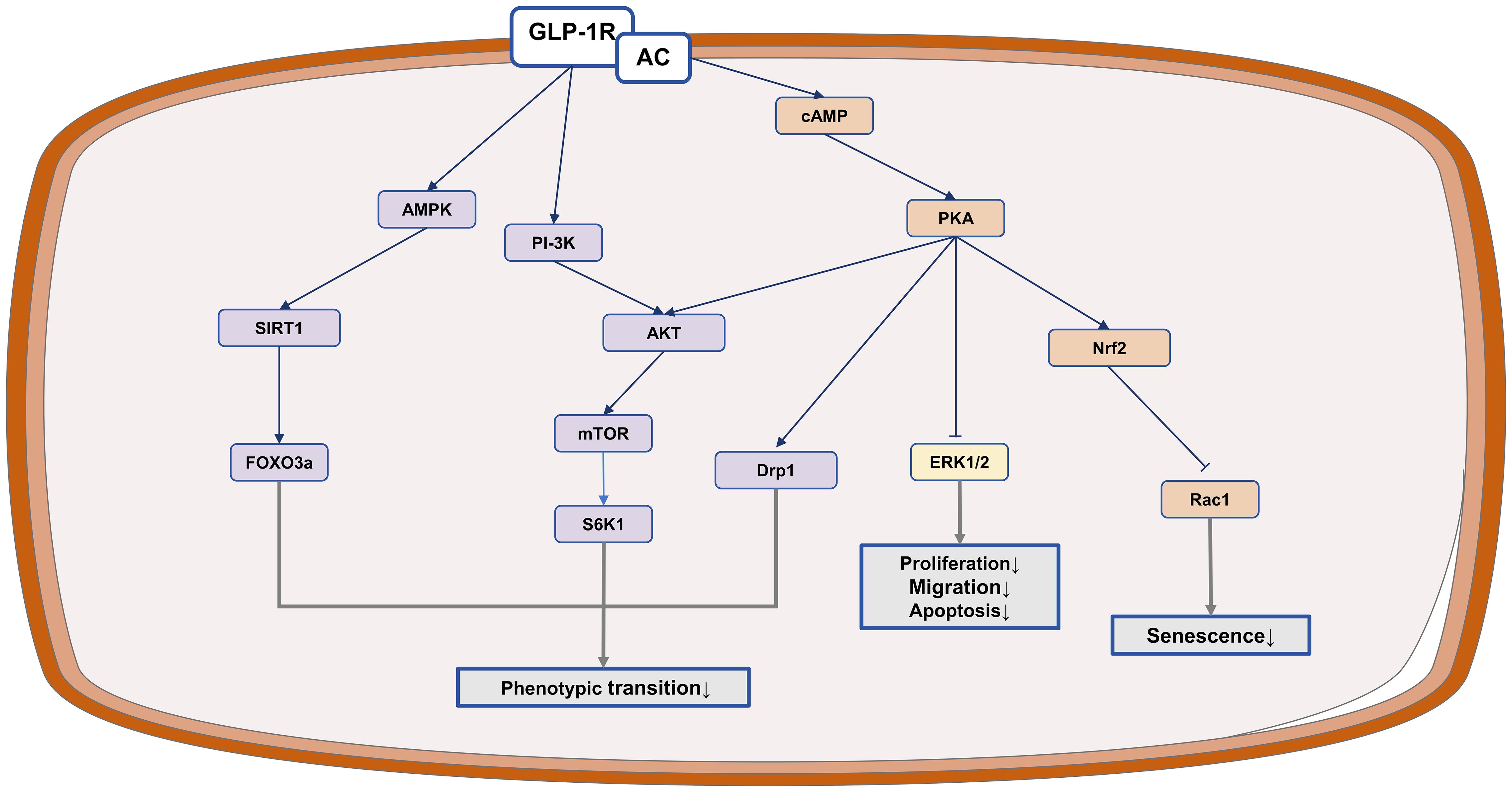
Figure 3. The anti-atherosclerotic potential of IRAs in VSMCs. GLP-1RAs exert protective effects in atherosclerosis by inhibiting VSMCs proliferation and migration.
3.5 Effect on the stability of atherosclerotic plaque
The rupture of atherosclerotic plaque can induce thrombosis and blockage of blood vessels, ultimately causing acute coronary syndromes (126). Several key factors contribute to the instability of a plaque and its susceptibility to rupture, including a thin or fragmented fibrous cap, a high concentration of macrophages within the plaque, and the presence of a lipid-filled necrotic core (127). Macrophages can exacerbate atherosclerosis and jeopardiz plaque stability, whereas VSMCs are typically viewed as a protective role in maintaining plaque stability (128). IRAs have been demonstrated to enhance the stability of atherosclerotic plaques through the following elaborated aspects.
3.5.1 Increasing the thickness of plaque fiber cap
GLP-1RAs treatment have shown a decrease in MMP-9 expression and an increase in plaque collagen content. It also reduces MMP-1 and MMP-9 levels while increasing TIMP-1 and TIMP-2 levels (129). Exenatide has been found to increase collagen content, prevent elastic lamina rupture, and inhibit neointimal formation. Moreover, it downregulates the expression of certain histone family members (CatL, CatK, and CatS) and MMP family members (MMP-2 and MMP-9) in stressed mice (130). The phenotypic transformation of VSMCs during atherosclerosis development leads to increased MMPs release, which can be inhibited by GLP-1RAs, thereby promoting plaque stability. Similarly, GIP treatment also reduces MMP-9 production and activity in mouse plaques, while enhancing plaque stability through increased collagen content (52).
3.5.2 Reducing the area of plaque lipid pool and alleviate plaque inflammation
GLP-1RAs have been shown to decrease the formation of foam cells from macrophages, thereby reducing macrophage-derived foam cells in plaques. This effect is accompanied by an increase in the expression of lipocalin, articulin PH protein, and leucine zipper containing 1 (APPL1), which in turn helps to decrease plaque inflammation (91). Similarly, GIP has been found to improve plaque stability by decreasing macrophage content and increasing collagen content in plaques, attributed to GIP's inhibitory effect on monocyte migration (52). Evidence also suggests that GIP directly inhibits plaque inflammation. These findings collectively indicate that GLP-1RAs plays a crucial role in promoting plaque stability.
Shortly, IRAs have demonstrated the ability to increase collagen content and thickness of the fibrous cap of plaques, maintaining a contracted phenotype of VSMCs. Simultaneously, they reduce plaque macrophage content, thereby decreasing the plaque lipid pool area and inflammation, ultimately enhancing plaque stability.
4 Polymorphisms of incretin receptor gene and the effect of cardiovascular protective
Both GLP-1RAs and GIPRAs belong to the class B1 G protein-coupled receptors. Upon activation by ligands, these receptors undergo structural changes from inactive to active states, initiating downstream signaling pathways. Variations in genes encoding these receptors can result in genotypic and allelic differences among populations, impacting individual responses to GLP-1RAs and GIPRAs (131). Specific genotypes and alleles may influence the efficacy of these drugs, leading to varying levels of blood glucose reduction. For instance, certain genotypes of the GLP-1R gene, such as the p.Arg131Gln mutation more commonly found in Japanese populations, have been associated with differences in glucose response to GLP-1RAs. These genetic variations in the GLP-1R gene could play a role in the diverse outcomes of blood glucose reduction observed among Japanese patients with T2DM (132).
The responsiveness to GLP-1 in vivo can be affected by genetic variations in the GLP-1R gene. Several nonsynonymous single nucleotide polymorphisms in this gene have been linked to changes in insulin secretion in response to injected GLP-1 during hyperglycemic conditions. For example, the single nucleotide polymorphism rs6923761 lead to the replacement of a glycine at position 168 with a serine, potentially reducing the responsiveness to injected GLP-1. Similarly, the GIP-R gene displays constitutive activity, and mutations that activate these receptors could increase the likelihood of functional abnormalities. These dysfunctional GIP-R activities may trigger physiological changes that could impact specific populations (133).
These studies emphasize the impact of genetic variations in the GIP-R and GLP-1R genes affect their response to their corresponding agonist treatment. The potential implications of these genetic variations for personalized medicine approaches are significant. Understanding a patient's enteric incretin receptor genotype and allele could be beneficial in individualized treatment.
5 The side effects of IRAs in clinical use
We have summarized several representative randomized controlled trials of IRAs and analyzed their adverse effects. Common side effects identified include gastrointestinal disorders, hypoglycemia, and cholelithiasis, while rare side effects encompass renal events, hepatic events, and pancreatitis (Table 3). Among these adverse effects, gastrointestinal reactions are the most prevalent, including symptoms such as nausea, vomiting, and diarrhea (136). The occurrence of these symptoms is dose-dependent and tends to diminish with continued use, which supports the recommendation to initiate therapy at a low dose and titrate upwards based on individual tolerability. Hypoglycemia is the second most common side effect that warrants attention, particularly when IRAs are used in combination with sulfonylureas or insulin (137, 138). This is especially important for certain elderly patients. Medical staff and families of patients should be vigilant regarding asymptomatic hypoglycemia during treatment (136).
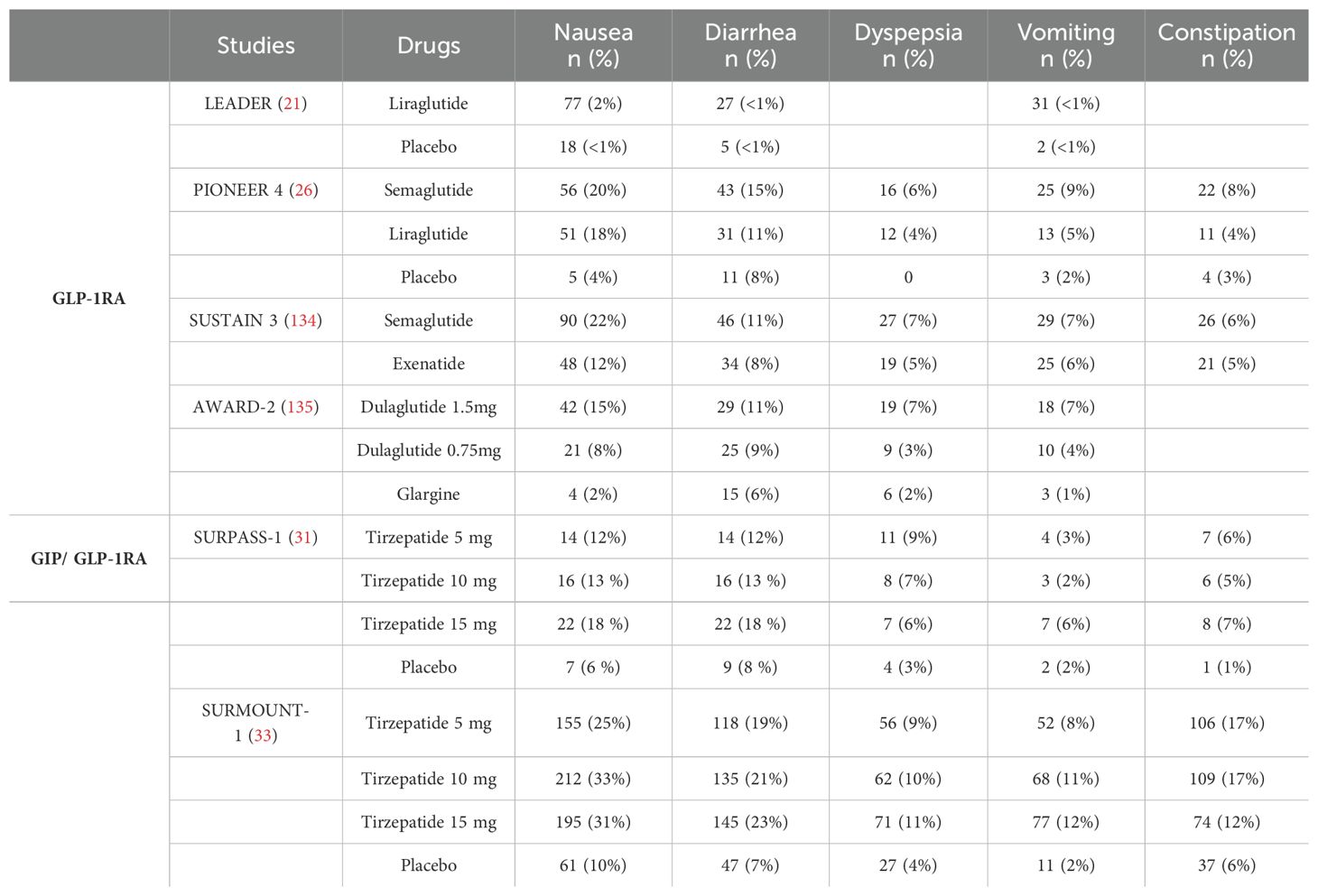
Table 3. Incidence of gastrointestinal adverse events in participants across major randomized controlled trials.
In comparison to control drugs or placebo, GLP-1RAs did not elevate the risk of renal damage with long-term use. While a small number of patients may experience renal impairment shortly after initiating treatment, this is likely linked to dehydration resulting from gastrointestinal symptoms or the patient's overall medication regimen (139). There is a paucity of pharmacokinetic data regarding patients undergoing treatment with IRAs. Although drug-induced hepatitis is a rare side effect associated with IRAs, their use is not advised for patients with significant liver impairment (139, 140). Furthermore, large meta-analyses provide little to no evidence concerning the effects of IRAs on pancreatitis, pancreatic cancer, and thyroid cancer. However, patients with a history of pancreatitis should exercise caution when using GLP-1RAs, and treatment should be discontinued immediately upon diagnosis of acute pancreatitis (141).
In summary, while IRAs provide substantial advantages in the management of T2DM, it is crucial to remain aware of potential side effects and to manage them effectively through careful monitoring and, when necessary, adjustments to the therapeutic regimen.
6 Future directions
Numerous studies have demonstrated the cardiovascular benefits of IRAs, which go beyond their blood sugar-lowering effects (142). The pleiotropic actions of IRAs are mediated through CV risk factor modification such as blood pressure, dyslipidemia, hyperglycemia along with direct effects on the functions of vascular cells. However, our understanding of the influence of IRAs on CVD is still limited. Several questions remain unanswered.
Firstly, the role of GIP in cardiovascular protection is a topic of debate in current research. Some studies suggest that GIP may have beneficial effects in reducing atherosclerosis by affecting macrophage infiltration and lipid deposition (143). However, other studies have shown that GIP/GIPR signaling could potentially promote atherosclerosis, as evidenced by increased osteopontin levels in cultured mouse aortas treated with GIP(1-42) (88). The impact of GIP on calcium signaling pathways, leading to the production of endothelin-1 (ET-1) and osteopontin (OPN), requires further investigation to understand its influence on endothelial cell function and atherosclerosis development (88). Secondly, the long-term safety and efficacy of GIP receptor agonists and antagonists in humans remain uncertain and further studies are necessary to evaluate their potential in reducing cardiovascular risk (15). Investigating the underlying mechanisms and clinical implications of GIP in atherosclerosis management is essential. Thirdly, the potential of genetic polymorphism in personalized IRAs treatment is highlighted in this study. By understanding a patient's GLP/GIP receptor genotypes and alleles, therapy can be personalized. Lastly, the possibility of non-diabetic patients benefiting from cardioprotection through the use of IRAs requires more investigation, and ongoing clinical trials are needed to address these questions.
7 Conclusions
The results presented in this review demonstrate that IRAs offer cardiovascular benefits beyond glycemic control, significantly improving CVD outcomes. Studies indicate that these agonists impact cardiovascular risk factors and outcomes, showing positive effects on lipid metabolism and hypertension. IRAs have been linked to reductions in LDL-c, TC, TG, and SBP. The mechanisms of action include reducing oxidative stress and apoptosis in endothelial cells, decreasing macrophage infiltration in plaques, and inhibiting VSMCs phenotypic switching, potentially slowing the progression of atherosclerosis. IRAs also reduce the lipid-rich core area and increase plaque collagen, enhancing plaque stability. These findings highlight the significance of IRAs in treating diabetic vascular complications. However, further research is necessary to fully understand the role of incretin in CVD and optimize its therapeutic applications.
Author contributions
XW: Visualization, Formal analysis, Data curation, Writing – review & editing, Writing – original draft. XY: Writing – review & editing, Writing – original draft. XQ: Writing – review & editing, Writing – original draft. GF: Writing – review & editing. LZ: Writing – review & editing. ZP: Writing – review & editing. JY: Funding acquisition, Writing – review & editing, Writing – original draft.
Funding
The author(s) declare financial support was received for the research, authorship, and/or publication of this article. The present study was supported by the National Natural Science Foundation of China (82170852), the Medicine Plus Program of Shenzhen University(2024YG017), the Major Science and Technology Project of Nanshan District Health System, Shenzhen City (NSZD2023044), and the Hunan Provincial Natural Science Foundation General Project (2021JJ30619).
Conflict of interest
The authors declare that the research was conducted in the absence of any commercial or financial relationships that could be construed as a potential conflict of interest.
Publisher’s note
All claims expressed in this article are solely those of the authors and do not necessarily represent those of their affiliated organizations, or those of the publisher, the editors and the reviewers. Any product that may be evaluated in this article, or claim that may be made by its manufacturer, is not guaranteed or endorsed by the publisher.
Abbreviations
IRAs, Incretin receptor agonists; GLP-1, glucagon-like peptide-1; GIP, glucose-dependent insulinotropic polypeptide; T2DM, type 2 diabetes mellitus; GLP-1RAs, GLP-1 receptor agonists; GIPRAs, GIP receptor agonists; GIP/GLP-1RAs, the dual GIP and GLP-1 co-agonists; GLP-1R, GLP-1 receptors; GIPR, GIP receptors; VSMCs, vascular smooth muscle cells; LDL, low-density lipoprotein; HDL, high-density lipoprotein; VLDL, very low-density lipoprotein; SBP, systolic blood pressure; CVOTs, cardiovascular outcome trials; MACE, major adverse cardiovascular events; ox-LDL, oxidized LDL; PKA, protein kinase A; cAMP, cyclic adenosine monophosphate; LPL, lipoprotein lipase; CREB, cAMP response element-binding protein; PI3K, phosphatidylinositol-3-kinase; Ang II, angiotensin II; NO, nitric oxide; ROS, reactive oxygen species; AMPK, AMP-activated protein kinase Akt, AMP-activated protein kinase; eNOS, endothelial NO synthase; NF-κB, nuclear factor kappa B; ET-1, endothelin-1; AGEs, advanced glycation end products; ICAM, intercellular adhesion molecule; VCAM, vascular cell adhesion molecule; MAPK, mitogen-activated protein kinase; SDF, stromal cell-derived factor; MLC, myosin light chain; Bcl-2, b-cell lymphoma 2; Bax, Bcl-2-associated X protein; ERK1/2, extracellular signal-regulated kinase 1/2; SIRT1, sirtuin 1; JNK, c-Jun N-terminal kinase; CD36, cluster of differentiation 36; ACAT-1, acyl-CoA,cholesterol acyltransferase 1; TNF-α, tumor necrosis factor alpha; MCP-1, monocyte chemoattractant protein-1; STAT, signal transducer and activator of transcription; mTOR, mechanistic target of rapamycin; MMPs, matrix metalloproteinases; TIMPs, inhibitors of metalloproteinases.
References
1. Morrish NJ, Wang SL, Stevens LK, Fuller JH, Keen H. Mortality and causes of death in the WHO Multinational Study of Vascular Disease in Diabetes. Diabetologia. (2001) 44 Suppl 2:S14–21. doi: 10.1007/PL00002934
2. Liu R, Li L, Shao C, Cai H, Wang Z. The impact of diabetes on vascular disease: progress from the perspective of epidemics and treatments. J Diabetes Res. (2022) 2022:1531289. doi: 10.1155/2022/1531289
3. Carr ME. Diabetes mellitus: a hypercoagulable state. J Diabetes Complications. (2001) 15:44–54. doi: 10.1016/S1056-8727(00)00132-X
4. Marx N, Davies MJ, Grant PJ, Mathieu C, Petrie JR, Cosentino F, et al. Guideline recommendations and the positioning of newer drugs in type 2 diabetes care. Lancet Diabetes Endocrinol. (2021) 9:46–52. doi: 10.1016/S2213-8587(20)30343-0
5. Campbell JE, Drucker DJ. Pharmacology, physiology, and mechanisms of incretin hormone action. Cell Metab. (2013) 17:819–37. doi: 10.1016/j.cmet.2013.04.008
6. El K, Gray SM, Capozzi ME, Knuth ER, Jin E, Svendsen B, et al. GIP mediates the incretin effect and glucose tolerance by dual actions on α cells and β cells. Sci Adv. (2021) 7:eabf1948. doi: 10.1126/sciadv.abf1948
7. White WB, Baker WL. Cardiovascular effects of incretin-based therapies. Annu Rev Med. (2016) 67:245–60. doi: 10.1146/annurev-med-050214-013431
8. Dandona P, Ghanim H, Chaudhuri A. Incretins: Beyond type 2 diabetes. Diabetes Obes Metab. (2018) 20 Suppl 1:59–67. doi: 10.1111/dom.13153
9. Sposito AC, Berwanger O, de Carvalho LSF, Saraiva JFK. GLP-1RAs in type 2 diabetes: mechanisms that underlie cardiovascular effects and overview of cardiovascular outcome data. Cardiovasc Diabetol. (2018) 17:157. doi: 10.1186/s12933-018-0800-2
10. Jonik S, Marchel M, Grabowski M, Opolski G, Mazurek T. Gastrointestinal incretins-glucose-dependent insulinotropic polypeptide (GIP) and glucagon-like peptide-1 (GLP-1) beyond pleiotropic physiological effects are involved in pathophysiology of atherosclerosis and coronary artery disease-state of the art. Biology. (2022) 11:288. doi: 10.3390/biology11020288
11. Rizzo M, Nikolic D, Patti AM, Mannina C, Montalto G, McAdams BS, et al. GLP-1 receptor agonists and reduction of cardiometabolic risk: Potential underlying mechanisms. Biochim Biophys Acta Mol Basis Dis. (2018) 1864:2814–21. doi: 10.1016/j.bbadis.2018.05.012
12. Heimbürger SM, Bergmann NC, Augustin R, Gasbjerg LS, Christensen MB, Knop FK. Glucose-dependent insulinotropic polypeptide (GIP) and cardiovascular disease. Peptides. (2020) 125:170174. doi: 10.1016/j.peptides.2019.170174
13. Baggio LL, Drucker DJ. Biology of incretins: GLP-1 and GIP. Gastroenterology. (2007) 132:2131–57. doi: 10.1053/j.gastro.2007.03.054
14. Ma X, Liu Z, Ilyas I, Little PJ, Kamato D, Sahebka A, et al. GLP-1 receptor agonists (GLP-1RAs): cardiovascular actions and therapeutic potential. Int J Biol Sci. (2021) 17:2050–68. doi: 10.7150/ijbs.59965
15. Mori Y, Matsui T, Hirano T, Yamagishi SI. GIP as a potential therapeutic target for atherosclerotic cardiovascular disease-A systematic review. Int J Mol Sci. (2020) 21:1509. doi: 10.3390/ijms21041509
16. Owens DR, Monnier L, Hanefeld M. A review of glucagon-like peptide-1 receptor agonists and their effects on lowering postprandial plasma glucose and cardiovascular outcomes in the treatment of type 2 diabetes mellitus. Diabetes Obes Metab. (2017) 19:1645–54. doi: 10.1111/dom.2017.19.issue-12
17. Nauck MA, Quast DR, Wefers J, Pfeiffer AFH. The evolving story of incretins (GIP and GLP-1) in metabolic and cardiovascular disease: A pathophysiological update. Diabetes Obes Metab. (2021) 23 Suppl 3:5–29. doi: 10.1111/dom.14496
18. Wilson JM, Nikooienejad A, Robins DA, Roell WC, Riesmeyer JS, Haupt A, et al. The dual glucose-dependent insulinotropic peptide and glucagon-like peptide-1 receptor agonist, tirzepatide, improves lipoprotein biomarkers associated with insulin resistance and cardiovascular risk in patients with type 2 diabetes. Diabetes Obes Metab. (2020) 22:2451–9. doi: 10.1111/dom.v22.12
19. Tan Q, Akindehin SE, Orsso CE, Waldner RC, DiMarchi RD, Müller TD, et al. Recent advances in incretin-based pharmacotherapies for the treatment of obesity and diabetes. Front Endocrinol. (2022) 13:838410. doi: 10.3389/fendo.2022.838410
20. Baggio LL, Drucker DJ. Glucagon-like peptide-1 receptor co-agonists for treating metabolic disease. Mol Metab. (2021) 46:101090. doi: 10.1016/j.molmet.2020.101090
21. Marso SP, Daniels GH, Brown-Frandsen K, Kristensen P, Mann JFE, Nauck MA, et al. Liraglutide and cardiovascular outcomes in type 2 diabetes. N Engl J Med. (2016) 375:311–22. doi: 10.1056/NEJMoa1603827
22. Williams TC, Stewart E. Semaglutide and cardiovascular outcomes in patients with type 2 diabetes. N Engl J Med. (2017) 376:891. doi: 10.1056/NEJMc1615712
23. Branch KRH, Dagenais GR, Avezum A, Basile J, Conget I, Cushman WC, et al. Dulaglutide and cardiovascular and heart failure outcomes in patients with and without heart failure: a post-hoc analysis from the REWIND randomized trial. Eur J Heart Failure. (2022) 24:1805–12. doi: 10.1002/ejhf.v24.10
24. Zinman B, Gerich J, Buse JB, Lewin A, Schwartz S, Raskin P, et al. Efficacy and safety of the human glucagon-like peptide-1 analog liraglutide in combination with metformin and thiazolidinedione in patients with type 2 diabetes (LEAD-4 Met+TZD). Diabetes Care. (2009) 32:1224–30. doi: 10.2337/dc08-2124
25. Aroda VR, Bain SC, Cariou B, Piletič M, Rose L, Axelsen M, et al. Efficacy and safety of once-weekly semaglutide versus once-daily insulin glargine as add-on to metformin (with or without sulfonylureas) in insulin-naive patients with type 2 diabetes (SUSTAIN 4): a randomised, open-label, parallel-group, multicentre, multinational, phase 3a trial. Lancet Diabetes Endocrinol. (2017) 5:355–66. doi: 10.1016/S2213-8587(17)30085-2
26. Pratley R, Amod A, Hoff ST, Kadowaki T, Lingvay I, Nauck M, et al. Oral semaglutide versus subcutaneous liraglutide and placebo in type 2 diabetes (PIONEER 4): a randomised, double-blind, phase 3a trial. Lancet (London England). (2019) 394:39–50. doi: 10.1016/S0140-6736(19)31271-1
27. Rubino D, Abrahamsson N, Davies M, Hesse D, Greenway FL, Jensen C, et al. Effect of continued weekly subcutaneous semaglutide vs placebo on weight loss maintenance in adults with overweight or obesity: the STEP 4 randomized clinical trial. Jama. (2021) 325:1414–25. doi: 10.1001/jama.2021.3224
28. Gerstein HC, Colhoun HM, Dagenais GR, Diaz R, Lakshmanan M, Pais P, et al. Dulaglutide and cardiovascular outcomes in type 2 diabetes (REWIND): a double-blind, randomised placebo-controlled trial. Lancet (London England). (2019) 394:121–30. doi: 10.1016/S0140-6736(19)31149-3
29. Rosenstock J, Buse JB, Azeem R, Prabhakar P, Kjems L, Huang H, et al. Efficacy and safety of ITCA 650, a novel drug-device GLP-1 receptor agonist, in type 2 diabetes uncontrolled with oral antidiabetes drugs: the FREEDOM-1 trial. Diabetes Care. (2018) 41:333–40. doi: 10.2337/dc17-1306
30. Gerstein HC, Sattar N, Rosenstock J, Ramasundarahettige C, Pratley R, Lopes RD, et al. Cardiovascular and renal outcomes with efpeglenatide in type 2 diabetes. N Engl J Med. (2021) 385:896–907. doi: 10.1056/NEJMoa2108269
31. Rosenstock J, Wysham C, Frías JP, Kaneko S, Lee CJ, Fernández Landó L, et al. Efficacy and safety of a novel dual GIP and GLP-1 receptor agonist tirzepatide in patients with type 2 diabetes (SURPASS-1): a double-blind, randomised, phase 3 trial. Lancet (London England). (2021) 398:143–55. doi: 10.1016/S0140-6736(21)01324-6
32. Frías JP, Davies MJ, Rosenstock J, Pérez Manghi FC, Fernández Landó L, Bergman BK, et al. Tirzepatide versus semaglutide once weekly in patients with type 2 diabetes. N Engl J Med. (2021) 385:503–15. doi: 10.1056/NEJMoa2107519
33. Jastreboff AM, Aronne LJ, Ahmad NN, Wharton S, Connery L, Alves B, et al. Tirzepatide once weekly for the treatment of obesity. N Engl J Med. (2022) 387:205–16. doi: 10.1056/NEJMoa2206038
34. Garvey WT, Frias JP, Jastreboff AM, le Roux CW, Sattar N, Aizenberg D, et al. Tirzepatide once weekly for the treatment of obesity in people with type 2 diabetes (SURMOUNT-2): a double-blind, randomised, multicentre, placebo-controlled, phase 3 trial. Lancet (London England). (2023) 402:613–26. doi: 10.1016/S0140-6736(23)01200-X
35. Choy PC, Siow YL, Mymin D, O K. Lipids and atherosclerosis. Biochem Cell Biol = Biochimie biologie cellulaire. (2004) 82:212–24. doi: 10.1139/o03-085
36. Sun F, Chai S, Li L, Yu K, Yang Z, Wu S, et al. Effects of glucagon-like peptide-1 receptor agonists on weight loss in patients with type 2 diabetes: a systematic review and network meta-analysis. J Diabetes Res. (2015) 2015:157201. doi: 10.1155/2015/157201
37. Stemmer K, Finan B, DiMarchi RD, Tschöp MH, Müller TD. Insights into incretin-based therapies for treatment of diabetic dyslipidemia. Adv Drug Deliv Rev. (2020) 159:34–53. doi: 10.1016/j.addr.2020.05.008
38. Getty-Kaushik L, Song DH, Boylan MO, Corkey BE, Wolfe MM. Glucose-dependent insulinotropic polypeptide modulates adipocyte lipolysis and reesterification. Obes (Silver Spring Md). (2006) 14:1124–31. doi: 10.1038/oby.2006.129
39. Nauck MA, D'Alessio DA. Tirzepatide, a dual GIP/GLP-1 receptor co-agonist for the treatment of type 2 diabetes with unmatched effectiveness regrading glycaemic control and body weight reduction. Cardiovasc Diabetol. (2022) 21:169. doi: 10.1186/s12933-022-01604-7
40. Stamler J, Neaton JD, Wentworth DN. Blood pressure (systolic and diastolic) and risk of fatal coronary heart disease. Hypertension (Dallas Tex 1979). (1989) 13:I2–12. doi: 10.1161/01.HYP.13.5_Suppl.I2
41. Sun F, Wu S, Guo S, Yu K, Yang Z, Li L, et al. Impact of GLP-1 receptor agonists on blood pressure, heart rate and hypertension among patients with type 2 diabetes: A systematic review and network meta-analysis. Diabetes Res Clin Practice. (2015) 110:26–37. doi: 10.1016/j.diabres.2015.07.015
42. Wilding JPH, Batterham RL, Calanna S, Davies M, Van Gaal LF, Lingvay I, et al. Once-weekly semaglutide in adults with overweight or obesity. N Engl J Med. (2021) 384:989–1002. doi: 10.1056/NEJMoa2032183
43. Wice BM, Reeds DN, Tran HD, Crimmins DL, Patterson BW, Dunai J, et al. Xenin-25 amplifies GIP-mediated insulin secretion in humans with normal and impaired glucose tolerance but not type 2 diabetes. Diabetes. (2012) 61:1793–800. doi: 10.2337/db11-1451
44. Dahl D, Onishi Y, Norwood P, Huh R, Bray R, Patel H, et al. Effect of subcutaneous tirzepatide vs placebo added to titrated insulin glargine on glycemic control in patients with type 2 diabetes: the SURPASS-5 randomized clinical trial. Jama. (2022) 327:534–45. doi: 10.1001/jama.2022.0078
45. Tsioufis C, Andrikou E, Thomopoulos C, Papanas N, Tousoulis D. Oral glucose-lowering drugs and cardiovascular outcomes: from the negative RECORD and ACCORD to neutral TECOS and promising EMPA-REG. Curr Vasc Pharmacol. (2017) 15:457–68. doi: 10.2174/1570161114666161208150642
46. Pfeffer MA, Claggett B, Diaz R, Dickstein K, Gerstein HC, Køber LV, et al. Lixisenatide in patients with type 2 diabetes and acute coronary syndrome. N Engl J Med. (2015) 373:2247–57. doi: 10.1056/NEJMoa1509225
47. Marso SP, Bain SC, Consoli A, Eliaschewitz FG, Jódar E, Leiter LA, et al. Semaglutide and cardiovascular outcomes in patients with type 2 diabetes. N Engl J Med. (2016) 375:1834–44. doi: 10.1056/NEJMoa1607141
48. Holman RR, Bethel MA, Mentz RJ, Thompson VP, Lokhnygina Y, Buse JB, et al. Effects of once-weekly exenatide on cardiovascular outcomes in type 2 diabetes. N Engl J Med. (2017) 377:1228–39. doi: 10.1056/NEJMoa1612917
49. Hernandez AF, Green JB, Janmohamed S, D'Agostino RB, Granger CB, Jones NP, et al. Albiglutide and cardiovascular outcomes in patients with type 2 diabetes and cardiovascular disease (Harmony Outcomes): a double-blind, randomised placebo-controlled trial. Lancet (London England). (2018) 392:1519–29. doi: 10.1016/S0140-6736(18)32261-X
50. Husain M, Birkenfeld AL, Donsmark M, Dungan K, Eliaschewitz FG, Franco DR, et al. Oral semaglutide and cardiovascular outcomes in patients with type 2 diabetes. N Engl J Med. (2019) 381:841–51. doi: 10.1056/NEJMoa1901118
51. Del Prato S, Kahn SE, Pavo I, Weerakkody GJ, Yang Z, Doupis J, et al. Tirzepatide versus insulin glargine in type 2 diabetes and increased cardiovascular risk (SURPASS-4): a randomised, open-label, parallel-group, multicentre, phase 3 trial. Lancet (London England). (2021) 398:1811–24. doi: 10.1016/S0140-6736(21)02188-7
52. Kahles F, Liberman A, Halim C, Rau M, Möllmann J, Mertens RW, et al. The incretin hormone GIP is upregulated in patients with atherosclerosis and stabilizes plaques in ApoE(-/-) mice by blocking monocyte/macrophage activation. Mol Metab. (2018) 14:150–7. doi: 10.1016/j.molmet.2018.05.014
54. Manduteanu I, Simionescu M. Inflammation in atherosclerosis: a cause or a result of vascular disorders? J Cell Mol Med. (2012) 16:1978–90. doi: 10.1111/j.1582-4934.2012.01552.x
55. Teo KK, Rafiq T. Cardiovascular risk factors and prevention: A perspective from developing countries. Can J Cardiol. (2021) 37:733–43. doi: 10.1016/j.cjca.2021.02.009
56. Eelen G, de Zeeuw P, Simons M, Carmeliet P. Endothelial cell metabolism in normal and diseased vasculature. Circ Res. (2015) 116:1231–44. doi: 10.1161/CIRCRESAHA.116.302855
57. Chistiakov DA, Melnichenko AA, Myasoedova VA, Grechko AV, Orekhov AN. Mechanisms of foam cell formation in atherosclerosis. J Mol Med (Berl). (2017) 95:1153–65. doi: 10.1007/s00109-017-1575-8
58. Khatana C, Saini NK, Chakrabarti S, Saini V, Sharma A, Saini RV, et al. Mechanistic insights into the oxidized low-density lipoprotein-induced atherosclerosis. Oxid Med Cell Longev. (2020) 2020:5245308. doi: 10.1155/2020/5245308
59. Bennett MR, Sinha S, Owens GK. Vascular smooth muscle cells in atherosclerosis. Circ Res. (2016) 118:692–702. doi: 10.1161/CIRCRESAHA.115.306361
60. Vincent J. Lipid lowering therapy for atherosclerotic cardiovascular disease: it is not so simple. Clin Pharmacol Ther. (2018) 104:220–4. doi: 10.1002/cpt.2018.104.issue-2
61. Ruiz-Grande C, Alarcón C, Mérida E, Valverde I. Lipolytic action of glucagon-like peptides in isolated rat adipocytes. Peptides. (1992) 13:13–6. doi: 10.1016/0196-9781(92)90134-O
62. Wójcicka G, Zaręba M, Warpas A, Jamroz-Wiśniewska A, Rusek M, Czechowska G, et al. The effect of exenatide (a GLP-1 analog) and sitagliptin (a DPP-4 inhibitor) on plasma platelet-activating factor acetylhydrolase (PAF-AH) activity and concentration in normal and fructose-fed rats. Eur J Pharmacol. (2019) 850:180–9. doi: 10.1016/j.ejphar.2019.02.014
63. Hsieh J, Longuet C, Baker CL, Qin B, Federico LM, Drucker DJ, et al. The glucagon-like peptide 1 receptor is essential for postprandial lipoprotein synthesis and secretion in hamsters and mice. Diabetologia. (2010) 53:552–61. doi: 10.1007/s00125-009-1611-5
64. Dahl K, Brooks A, Almazedi F, Hoff ST, Boschini C, Baekdal TA. Oral semaglutide improves postprandial glucose and lipid metabolism, and delays gastric emptying, in subjects with type 2 diabetes. Diabetes Obes Metab. (2021) 23:1594–603. doi: 10.1111/dom.14373
65. Kooijman S, Wang Y, Parlevliet ET, Boon MR, Edelschaap D, Snaterse G, et al. Central GLP-1 receptor signalling accelerates plasma clearance of triacylglycerol and glucose by activating brown adipose tissue in mice. Diabetologia. (2015) 58:2637–46. doi: 10.1007/s00125-015-3727-0
66. Hauner H, Glatting G, Kaminska D, Pfeiffer EF. Effects of gastric inhibitory polypeptide on glucose and lipid metabolism of isolated rat adipocytes. Ann Nutr Metab. (1988) 32:282–8. doi: 10.1159/000177467
67. Kim SJ, Nian C, McIntosh CH. Activation of lipoprotein lipase by glucose-dependent insulinotropic polypeptide in adipocytes. A role for a protein kinase B, LKB1, and AMP-activated protein kinase cascade. J Biol Chem. (2007) 282:8557–67. doi: 10.1074/jbc.M609088200
68. Kim SJ, Nian C, McIntosh CH. GIP increases human adipocyte LPL expression through CREB and TORC2-mediated trans-activation of the LPL gene. J Lipid Res. (2010) 51:3145–57. doi: 10.1194/jlr.M006841
69. Lastra G, Syed S, Kurukulasuriya LR, Manrique C, Sowers JR. Type 2 diabetes mellitus and hypertension: an update. Endocrinol Metab Clin North Am. (2014) 43:103–22. doi: 10.1016/j.ecl.2013.09.005
70. Moreno C, Mistry M, Roman RJ. Renal effects of glucagon-like peptide in rats. Eur J Pharmacol. (2002) 434:163–7. doi: 10.1016/S0014-2999(01)01542-4
71. Skov J, Dejgaard A, Frøkiær J, Holst JJ, Jonassen T, Rittig S, et al. Glucagon-like peptide-1 (GLP-1): effect on kidney hemodynamics and renin-angiotensin-aldosterone system in healthy men. J Clin Endocrinol Metab. (2013) 98:E664–E71. doi: 10.1210/jc.2012-3855
72. Jensen EP, Møller S, Hviid AV, Veedfald S, Holst JJ, Pedersen J, et al. GLP-1-induced renal vasodilation in rodents depends exclusively on the known GLP-1 receptor and is lost in prehypertensive rats. Am J Physiol Renal Physiol. (2020) 318:F1409–17. doi: 10.1152/ajprenal.00579.2019
73. Helmstädter J, Frenis K, Filippou K, Grill A, Dib M, Kalinovic S, et al. Endothelial GLP-1 (Glucagon-like peptide-1) receptor mediates cardiovascular protection by liraglutide in mice with experimental arterial hypertension. Arterioscler Thromb Vasc Biol. (2020) 40:145–58. doi: 10.1161/atv.0000615456.97862.30
74. Karstoft K, Mortensen SP, Knudsen SH, Solomon TPJ. Direct effect of incretin hormones on glucose and glycerol metabolism and hemodynamics. Am J Physiol Endocrinol Metab. (2015) 308:E426–E33. doi: 10.1152/ajpendo.00520.2014
75. Ding KH, Zhong Q, Xu J, Isales CM. Glucose-dependent insulinotropic peptide: differential effects on hepatic artery vs. portal vein endothelial cells. Am J Physiol Endocrinol Metab. (2004) 286:E773–9. doi: 10.1152/ajpendo.00507.2003
76. Mori Y, Kushima H, Koshibu M, Saito T, Hiromura M, Kohashi K, et al. Glucose-dependent insulinotropic polypeptide suppresses peripheral arterial remodeling in male mice. Endocrinology. (2018) 159:2717–32. doi: 10.1210/en.2018-00336
77. Rajendran P, Rengarajan T, Thangavel J, Nishigaki Y, Sakthisekaran D, Sethi G, et al. The vascular endothelium and human diseases. Int J Biol Sci. (2013) 9:1057–69. doi: 10.7150/ijbs.7502
78. Gimbrone MA Jr., García-Cardeña G. Endothelial cell dysfunction and the pathobiology of atherosclerosis. Circ Res. (2016) 118:620–36. doi: 10.1161/CIRCRESAHA.115.306301
79. Madden JA. Role of the vascular endothelium and plaque in acute ischemic stroke. Neurology. (2012) 79:S58–62. doi: 10.1212/WNL.0b013e3182695836
80. Le Y, Wei R, Yang K, Lang S, Gu L, Liu J, et al. Liraglutide ameliorates palmitate-induced oxidative injury in islet microvascular endothelial cells through GLP-1 receptor/PKA and GTPCH1/eNOS signaling pathways. Peptides. (2020) 124:170212. doi: 10.1016/j.peptides.2019.170212
81. Dai Y, Mehta JL, Chen M. Glucagon-like peptide-1 receptor agonist liraglutide inhibits endothelin-1 in endothelial cell by repressing nuclear factor-kappa B activation. Cardiovasc Drugs Ther. (2013) 27:371–80. doi: 10.1007/s10557-013-6463-z
82. Shiraki A, Oyama J, Komoda H, Asaka M, Komatsu A, Sakuma M, et al. The glucagon-like peptide 1 analog liraglutide reduces TNF-α-induced oxidative stress and inflammation in endothelial cells. Atherosclerosis. (2012) 221:375–82. doi: 10.1016/j.atherosclerosis.2011.12.039
83. Zhang Y, Wang S, Chen X, Wang Z, Wang X, Zhou Q, et al. Liraglutide prevents high glucose induced HUVECs dysfunction via inhibition of PINK1/Parkin-dependent mitophagy. Mol Cell Endocrinol. (2022) 545:111560. doi: 10.1016/j.mce.2022.111560
84. Cheng CK, Luo JY, Lau CW, Cho WC, Ng CF, Ma RCW, et al. A GLP-1 analog lowers ER stress and enhances protein folding to ameliorate homocysteine-induced endothelial dysfunction. Acta Pharmacologica Sinica. (2021) 42:1598–609. doi: 10.1038/s41401-020-00589-x
85. Förstermann U, Closs EI, Pollock JS, Nakane M, Schwarz P, Gath I, et al. Nitric oxide synthase isozymes. Characterization, purification, molecular cloning, and functions. Hypertension (Dallas Tex 1979). (1994) 23:1121–31. doi: 10.1161/01.HYP.23.6.1121
86. Zhong Q, Bollag RJ, Dransfield DT, Gasalla-Herraiz J, Ding KH, Min L, et al. Glucose-dependent insulinotropic peptide signaling pathways in endothelial cells. Peptides. (2000) 21:1427–32. doi: 10.1016/S0196-9781(00)00287-4
87. Ojima A, Matsui T, Maeda S, Takeuchi M, Yamagishi S. Glucose-dependent insulinotropic polypeptide (GIP) inhibits signaling pathways of advanced glycation end products (AGEs) in endothelial cells via its antioxidative properties. Hormone Metab Res = Hormon- und Stoffwechselforschung = Hormones metabolisme. (2012) 44:501–5. doi: 10.1055/s-0032-1312595
88. Berglund LM, Lyssenko V, Ladenvall C, Kotova O, Edsfeldt A, Pilgaard K, et al. Glucose-dependent insulinotropic polypeptide stimulates osteopontin expression in the vasculature via endothelin-1 and CREB. Diabetes. (2016) 65:239–54. doi: 10.2337/db15-0122
89. Ding KH, Zhong Q, Isales CM. Glucose-dependent insulinotropic peptide stimulates thymidine incorporation in endothelial cells: role of endothelin-1. Am J Physiol Endocrinol Metab. (2003) 285:E390–6. doi: 10.1152/ajpendo.00509.2002
90. Yue W, Li Y, Ou D, Yang Q. The GLP-1 receptor agonist liraglutide protects against oxidized LDL-induced endothelial inflammation and dysfunction via KLF2. IUBMB Life. (2019) 71:1347–54. doi: 10.1002/iub.v71.9
91. Barbieri M, Marfella R, Esposito A, Rizzo MR, Angellotti E, Mauro C, et al. Incretin treatment and atherosclerotic plaque stability: Role of adiponectin/APPL1 signaling pathway. J Diabetes Complications. (2017) 31:295–303. doi: 10.1016/j.jdiacomp.2016.10.001
92. Gaspari T, Liu H, Welungoda I, Hu Y, Widdop RE, Knudsen LB, et al. A GLP-1 receptor agonist liraglutide inhibits endothelial cell dysfunction and vascular adhesion molecule expression in an ApoE-/- mouse model. Diabetes Vasc Dis Res. (2011) 8:117–24. doi: 10.1177/1479164111404257
93. Arakawa M, Mita T, Azuma K, Ebato C, Goto H, Nomiyama T, et al. Inhibition of monocyte adhesion to endothelial cells and attenuation of atherosclerotic lesion by a glucagon-like peptide-1 receptor agonist, exendin-4. Diabetes. (2010) 59:1030–7. doi: 10.2337/db09-1694
94. Tang ST, Tang HQ, Su H, Wang Y, Zhou Q, Zhang Q, et al. Glucagon-like peptide-1 attenuates endothelial barrier injury in diabetes via cAMP/PKA mediated down-regulation of MLC phosphorylation. Biomedicine pharmacotherapy = Biomedecine pharmacotherapie. (2019) 113:108667. doi: 10.1016/j.biopha.2019.108667
95. Zampetaki A, Kirton JP, Xu Q. Vascular repair by endothelial progenitor cells. Cardiovasc Res. (2008) 78:413–21. doi: 10.1093/cvr/cvn081
96. Cai X, She M, Xu M, Chen H, Li J, Chen X, et al. GLP-1 treatment protects endothelial cells from oxidative stress-induced autophagy and endothelial dysfunction. Int J Biol Sci. (2018) 14:1696–708. doi: 10.7150/ijbs.27774
97. Zhan Y, Sun HL, Chen H, Zhang H, Sun J, Zhang Z, et al. Glucagon-like peptide-1 (GLP-1) protects vascular endothelial cells against advanced glycation end products (AGEs)-induced apoptosis. Med Sci Monitor Int Med J Exp Clin Res. (2012) 18:Br286–91. doi: 10.12659/MSM.883207
98. Wu H, Xiao C, Zhao Y, Yin H, Yu M. Liraglutide Improves Endothelial Function via the mTOR Signaling Pathway. J Diabetes Res. (2021) 2021:2936667. doi: 10.1155/2021/2936667
99. Erdogdu Ö, Eriksson L, Nyström T, Sjöholm Å, Zhang Q. Exendin-4 restores glucolipotoxicity-induced gene expression in human coronary artery endothelial cells. Biochem Biophys Res Commun. (2012) 419:790–5. doi: 10.1016/j.bbrc.2012.02.106
100. Di Y, He J, Ma P, Shen N, Niu C, Liu X, et al. Liraglutide promotes the angiogenic ability of human umbilical vein endothelial cells through the JAK2/STAT3 signaling pathway. Biochem Biophys Res Commun. (2020) 523:666–71. doi: 10.1016/j.bbrc.2020.01.004
101. Jujić A, Nilsson PM, Atabaki-Pasdar N, Dieden A, Tuomi T, Franks PW, et al. Glucose-dependent insulinotropic peptide in the high-normal range is associated with increased carotid intima-media thickness. Diabetes Care. (2021) 44:224–30. doi: 10.2337/dc20-1318
102. Ross R. Atherosclerosis–an inflammatory disease. N Engl J Med. (1999) 340:115–26. doi: 10.1056/NEJM199901143400207
103. Koelwyn GJ, Corr EM, Erbay E, Moore KJ. Regulation of macrophage immunometabolism in atherosclerosis. Nat Immunol. (2018) 19:526–37. doi: 10.1038/s41590-018-0113-3
104. Wilson HM. The intracellular signaling pathways governing macrophage activation and function in human atherosclerosis. Biochem Soc Trans. (2022) 50:1673–82. doi: 10.1042/BST20220441
105. Tashiro Y, Sato K, Watanabe T, Nohtomi K, Terasaki M, Nagashima M, et al. A glucagon-like peptide-1 analog liraglutide suppresses macrophage foam cell formation and atherosclerosis. Peptides. (2014) 54:19–26. doi: 10.1016/j.peptides.2013.12.015
106. Dai Y, Dai D, Wang X, Ding Z, Li C, Mehta JL. GLP-1 agonists inhibit ox-LDL uptake in macrophages by activating protein kinase A. J Cardiovasc Pharmacol. (2014) 64:47–52. doi: 10.1097/FJC.0000000000000087
107. Nagashima M, Watanabe T, Terasaki M, Tomoyasu M, Nohtomi K, Kim-Kaneyama J, et al. Native incretins prevent the development of atherosclerotic lesions in apolipoprotein E knockout mice. Diabetologia. (2011) 54:2649–59. doi: 10.1007/s00125-011-2241-2
108. Terasaki M, Yashima H, Mori Y, Saito T, Shiraga Y, Kawakami R, et al. Glucose-dependent insulinotropic polypeptide suppresses foam cell formation of macrophages through inhibition of the cyclin-dependent kinase 5-CD36 pathway. Biomedicines. (2021) 9:832. doi: 10.3390/biomedicines9070832
109. Pujadas G, Baggio LL, Kaur KD, McLean BA, Cao X, Drucker DJ. Genetic disruption of the Gipr in Apoe(-/-) mice promotes atherosclerosis. Mol Metab. (2022) 65:101586. doi: 10.1016/j.molmet.2022.101586
110. Chen J, Mei A, Liu X, Braunstein Z, Wei Y, Wang B, et al. Glucagon-like peptide-1 receptor regulates macrophage migration in monosodium urate-induced peritoneal inflammation. Front Immunol. (2022) 13:772446. doi: 10.3389/fimmu.2022.772446
111. Vinué Á, Navarro J, Herrero-Cervera A, García-Cubas M, Andrés-Blasco I, Martínez-Hervás S, et al. The GLP-1 analogue lixisenatide decreases atherosclerosis in insulin-resistant mice by modulating macrophage phenotype. Diabetologia. (2017) 60:1801–12. doi: 10.1007/s00125-017-4330-3
112. Bruen R, Curley S, Kajani S, Crean D, O'Reilly ME, Lucitt MB, et al. Liraglutide dictates macrophage phenotype in apolipoprotein E null mice during early atherosclerosis. Cardiovasc Diabetol. (2017) 16:143. doi: 10.1186/s12933-017-0626-3
113. Rzucidlo EM, Martin KA, Powell RJ. Regulation of vascular smooth muscle cell differentiation. J Vasc Surg. (2007) 45 Suppl A:A25–32. doi: 10.1016/j.jvs.2007.03.001
114. Basatemur GL, Jørgensen HF, Clarke MCH, Bennett MR, Mallat Z. Vascular smooth muscle cells in atherosclerosis. Nat Rev Cardiol. (2019) 16:727–44. doi: 10.1038/s41569-019-0227-9
115. Grootaert MOJ, Moulis M, Roth L, Martinet W, Vindis C, Bennett MR, et al. Vascular smooth muscle cell death, autophagy and senescence in atherosclerosis. Cardiovasc Res. (2018) 114:622–34. doi: 10.1093/cvr/cvy007
116. Goto H, Nomiyama T, Mita T, Yasunari E, Azuma K, Komiya K, et al. Exendin-4, a glucagon-like peptide-1 receptor agonist, reduces intimal thickening after vascular injury. Biochem Biophys Res Commun. (2011) 405:79–84. doi: 10.1016/j.bbrc.2010.12.131
117. Shi L, Ji Y, Jiang X, Zhou L, Xu Y, Li Y, et al. Liraglutide attenuates high glucose-induced abnormal cell migration, proliferation, and apoptosis of vascular smooth muscle cells by activating the GLP-1 receptor, and inhibiting ERK1/2 and PI3K/Akt signaling pathways. Cardiovasc Diabetol. (2015) 14:18. doi: 10.1186/s12933-015-0177-4
118. Nagayama K, Kyotani Y, Zhao J, Ito S, Ozawa K, Bolstad FA, et al. Exendin-4 prevents vascular smooth muscle cell proliferation and migration by angiotensin II via the inhibition of ERK1/2 and JNK signaling pathways. PloS One. (2015) 10:e0137960. doi: 10.1371/journal.pone.0137960
119. Ji J, Feng M, Niu X, Zhang X, Wang Y. Liraglutide blocks the proliferation, migration and phenotypic switching of Homocysteine (Hcy)-induced vascular smooth muscle cells (VSMCs) by suppressing proprotein convertase subtilisin kexin9 (PCSK9)/ low-density lipoprotein receptor (LDLR). Bioengineered. (2021) 12:8057–66. doi: 10.1080/21655979.2021.1982304
120. Torres G, Morales PE, García-Miguel M, Norambuena-Soto I, Cartes-Saavedra B, Vidal-Peña G, et al. Glucagon-like peptide-1 inhibits vascular smooth muscle cell dedifferentiation through mitochondrial dynamics regulation. Biochem Pharmacol. (2016) 104:52–61. doi: 10.1016/j.bcp.2016.01.013
121. Zhan JK, Wang YJ, Wang Y, Tang ZY, Tan P, Huang W, et al. The protective effect of GLP-1 analogue in arterial calcification through attenuating osteoblastic differentiation of human VSMCs. Int J Cardiol. (2015) 189:188–93. doi: 10.1016/j.ijcard.2015.04.086
122. Di B, Li HW, Li W, Hua B. Liraglutide inhibited AGEs induced coronary smooth muscle cell phenotypic transition through inhibiting the NF-κB signal pathway. Peptides. (2019) 112:125–32. doi: 10.1016/j.peptides.2018.11.008
123. Liu Z, Zhang M, Zhou T, Shen Q, Qin X. Exendin-4 promotes the vascular smooth muscle cell re-differentiation through AMPK/SIRT1/FOXO3a signaling pathways. Atherosclerosis. (2018) 276:58–66. doi: 10.1016/j.atherosclerosis.2018.07.016
124. Zhao L, Li AQ, Zhou TF, Zhang MQ, Qin XM. Exendin-4 alleviates angiotensin II-induced senescence in vascular smooth muscle cells by inhibiting Rac1 activation via a cAMP/PKA-dependent pathway. Am J Physiol Cell Physiol. (2014) 307:C1130–C41. doi: 10.1152/ajpcell.00151.2014
125. Zhou T, Zhang M, Zhao L, Li A, Qin X. Activation of Nrf2 contributes to the protective effect of Exendin-4 against angiotensin II-induced vascular smooth muscle cell senescence. Am J Physiol Cell Physiol. (2016) 311:C572–C82. doi: 10.1152/ajpcell.00093.2016
126. Grønholdt ML, Dalager-Pedersen S, Falk E. Coronary atherosclerosis: determinants of plaque rupture. Eur Heart J. (1998) 19 Suppl C:C24–C9.
127. Ylä-Herttuala S, Bentzon JF, Daemen M, Falk E, Garcia-Garcia HM, Herrmann J, et al. Stabilisation of atherosclerotic plaques. Position paper of the European Society of Cardiology (ESC) Working Group on atherosclerosis and vascular biology. Thromb Haemostasis. (2011) 106:1–19. doi: 10.1160/th10-12-0784
128. Yurdagul A. Crosstalk between macrophages and vascular smooth muscle cells in atherosclerotic plaque stability. Arterioscler Thromb Vasc Biol. (2022) 42:372–80. doi: 10.1161/ATVBAHA.121.316233
129. Burgmaier M, Liberman A, Möllmann J, Kahles F, Reith S, Lebherz C, et al. Glucagon-like peptide-1 (GLP-1) and its split products GLP-1(9-37) and GLP-1(28-37) stabilize atherosclerotic lesions in apoe⁻/⁻ mice. Atherosclerosis. (2013) 231:427–35. doi: 10.1016/j.atherosclerosis.2013.08.033
130. Yang G, Lei Y, Inoue A, Piao L, Hu L, Jiang H, et al. Exenatide mitigated diet-induced vascular aging and atherosclerotic plaque growth in ApoE-deficient mice under chronic stress. Atherosclerosis. (2017) 264:1–10. doi: 10.1016/j.atherosclerosis.2017.07.014
131. Fortin JP, Schroeder JC, Zhu Y, Beinborn M, Kopin AS. Pharmacological characterization of human incretin receptor missense variants. J Pharmacol Exp Ther. (2010) 332:274–80. doi: 10.1124/jpet.109.160531
132. Suzuki K, Akiyama M, Ishigaki K, Kanai M, Hosoe J, Shojima N, et al. Identification of 28 new susceptibility loci for type 2 diabetes in the Japanese population. Nat Genet. (2019) 51:379–86. doi: 10.1038/s41588-018-0332-4
133. Sathananthan A, Man CD, Micheletto F, Zinsmeister AR, Camilleri M, Giesler PD, et al. Common genetic variation in GLP1R and insulin secretion in response to exogenous GLP-1 in nondiabetic subjects: a pilot study. Diabetes Care. (2010) 33:2074–6. doi: 10.2337/dc10-0200
134. Ahmann AJ, Capehorn M, Charpentier G, Dotta F, Henkel E, Lingvay I, et al. Efficacy and safety of once-weekly semaglutide versus exenatide ER in subjects with type 2 diabetes (SUSTAIN 3): A 56-week, open-label, randomized clinical trial. Diabetes Care. (2018) 41:258–66. doi: 10.2337/dc17-0417
135. Giorgino F, Benroubi M, Sun J-H, Zimmermann AG, Pechtner V. Efficacy and safety of once-weekly dulaglutide versus insulin glargine in patients with type 2 diabetes on metformin and glimepiride (AWARD-2). Diabetes Care. (2015) 38:2241–9. doi: 10.2337/dc14-1625
136. Aroda VR, Ratner R. The safety and tolerability of GLP-1 receptor agonists in the treatment of type 2 diabetes: a review. Diabetes/metabolism Res Rev. (2011) 27:528–42. doi: 10.1002/dmrr.v27.6
137. Moretto TJ, Milton DR, Ridge TD, Macconell LA, Okerson T, Wolka AM, et al. Efficacy and tolerability of exenatide monotherapy over 24 weeks in antidiabetic drug-naive patients with type 2 diabetes: a randomized, double-blind, placebo-controlled, parallel-group study. Clin Ther. (2008) 30:1448–60. doi: 10.1016/j.clinthera.2008.08.006
138. de Heer J, Holst JJ. Sulfonylurea compounds uncouple the glucose dependence of the insulinotropic effect of glucagon-like peptide 1. Diabetes. (2007) 56:438–43. doi: 10.2337/db06-0738
139. Soldevila B, Puig-Domingo M. Safety and tolerability of GLP-1 receptor agonists. Med Clin (Barc). (2014) 143 Suppl 2:35–40. doi: 10.1016/S0025-7753(14)70107-1
140. Parks M, Rosebraugh C. Weighing risks and benefits of liraglutide–the FDA's review of a new antidiabetic therapy. N Engl J Med. (2010) 362:774–7. doi: 10.1056/NEJMp1001578
141. Nathan DM, Buse JB, Davidson MB, Ferrannini E, Holman RR, Sherwin R, et al. Medical management of hyperglycemia in type 2 diabetes: a consensus algorithm for the initiation and adjustment of therapy: a consensus statement of the American Diabetes Association and the European Association for the Study of Diabetes. Diabetes Care. (2009) 32:193–203. doi: 10.2337/dc08-9025
142. Reed J, Bain S, Kanamarlapudi V. Recent advances in understanding the role of glucagon-like peptide 1. F1000Research. (2020) 9:239. doi: 10.12688/f1000research
Keywords: incretin receptor agonists, type 2 diabetes mellitus, cardiovascular disease, atherosclerosis, vasculoprotective effects
Citation: Wang X, Yang X, Qi X, Fan G, Zhou L, Peng Z and Yang J (2024) Anti-atherosclerotic effect of incretin receptor agonists. Front. Endocrinol. 15:1463547. doi: 10.3389/fendo.2024.1463547
Received: 12 July 2024; Accepted: 30 September 2024;
Published: 18 October 2024.
Edited by:
Rudolf Lucas, Augusta University, United StatesReviewed by:
Cosmin Mihai Vesa, University of Oradea, RomaniaYinan Jiang, University of Pittsburgh, United States
Copyright © 2024 Wang, Yang, Qi, Fan, Zhou, Peng and Yang. This is an open-access article distributed under the terms of the Creative Commons Attribution License (CC BY). The use, distribution or reproduction in other forums is permitted, provided the original author(s) and the copyright owner(s) are credited and that the original publication in this journal is cited, in accordance with accepted academic practice. No use, distribution or reproduction is permitted which does not comply with these terms.
*Correspondence: Jing Yang, eWFuZ2ppbmc5ODAzQGhvdG1haWwuY29t; Zhengliang Peng, cGVuZ3pobC0yMDAxQDE2My5jb20=
†These authors have contributed equally to this work