- 1The Second Clinical Medical College of Lanzhou University, Lanzhou, Gansu, China
- 2Department of Endocrinology and Metabolism, Lanzhou University Second Hospital, Lanzhou, Gansu, China
Although pituitary tumors (PTs) are mostly benign, some PTs are characterized by low surgical resection rates, high recurrence rates, and poor response to conventional treatments and profoundly affect patients’ quality of life. Everolimus (EVE) is the only FDA-approved mTOR inhibitor, which can be used for oral treatment. It effectively inhibits tumor cell proliferation and angiogenesis. It has been administered for various neuroendocrine tumors of the digestive tract, lungs, and pancreas. EVE not only suppresses the growth and proliferation of APT cells but also enhances their sensitivity to radiotherapy and chemotherapy. This review introduces the role of the PI3K/AKT/mTOR pathway in the development of APTs, comprehensively explores the current status of preclinical and clinical research of EVE in APTs, and discusses the blood-brain barrier permeability and safety of EVE.
1 Introduction
Pituitary tumors (PTs) are a group of tumors originating from the adenohypophysis, neurohypophysis, and remnants of the squamous epithelial cells of the embryonic craniopharyngeal duct, accounting for nearly 10%-25% of all intracranial tumors (1–4). Although PTs are mostly benign, some adenomas present with radiological signs of invasion and progress much faster than typical adenomas. Despite administering standard treatments, these tumors continue to grow and/or secrete excessive hormones and are categorized as aggressive pituitary tumors (APTs) (5–7). APTs account for 0.5%-10% of all PAs, with an incidence rate of approximately 0.1 to 0.2 cases per 100,000 individuals (8–11). They are characterized by aggressive invasion and high recurrence rates and significantly affect patients’ quality of life, which makes clinical management challenging (12–14).
Surgery is the primary treatment modality for all PTs except Prolactinoma (PRL-PAs). Although surgery can ameliorate the compressive effects of the tumor and partly reduce hormone secretion, the low rate of complete resection and high recurrence rates are often attributed to the widespread infiltration of critical structures, such as the sole of the saddle, slopes, or cavernous sinuses (15, 16). Reports indicated that 77% of APTs patients underwent at least two surgeries, with 28% requiring a minimum of four procedures (17). Radiotherapy is recommended for PTs with postoperative tumor growth and inadequate drug therapy; however, its effectiveness remains uncertain. The study showed that 45% of 143 patients had tumor shrinkage after radiotherapy, however nearly 40% received repeat radiotherapy after 5.4 years due to tumor progression (18). Radiotherapy may also lead to hypopituitarism, optic nerve damage, or cognitive deficits (19–22). The 2018 update of the European Society of Endocrinology guidelines on APTs and pituitary carcinomas recommended temozolomide (TMZ), an alkylating agent, as the first-line chemotherapeutic agent for APTs (23–27). However, one study indicated that only 9.6% of patients achieved complete remission with TMZ (18). Moreover, the long-term use of alkylating agents may increase the risk of malignancies, such as lymphomas and leukemias (28). Therefore, effective treatment strategies for APTs are currently lacking.
As a critical regulator of cellular growth (29–32), metabolism (32–34), and apoptosis (35–38), the mTOR pathway has recently received much attention in tumorigenesis (39–42). Everolimus (EVE), the only approved oral mTOR inhibitor can effectively suppress tumor cell proliferation, ameliorate cellular oxidative stress, and show anti-angiogenic properties (43–47). The Food and Drug Administration has approved EVE for treating neuroendocrine tumors (NETs) originating from the digestive tract, lungs, or pancreas (48–50). Overactivation of the mTOR pathway is also evident in PTs, a NETs (51, 52). Compared to normal pituitary tissue, significantly higher phosphorylation levels of nuclear p-AKT and cytoplasmic p-S6 and overall phosphorylation of eukaryotic translation initiation factor 4E-binding protein 1 (4EBP1) have been observed in PTs (53). The study demonstrated that EVE inhibited adenoma cell proliferation and concurrently decreased hormone secretion (54). Several large-scale clinical trials have shown that EVE has good overall tolerability and leads to a few severe adverse reactions (55–59). Therefore, EVE may serve as a potential alternative treatment option for patients with PTs who are resistant to conventional treatments. This article reviewed the research progress of EVE in PAs and other NETs, and investigated its blood-brain barrier (BBB) permeability and drug safety, providing new options for managing PTs.
2 Molecular mechanisms of function of EVE in PTs
2.1 Overview of the PI3K/AKT/mTOR pathway
The PI3K/AKT/mTOR pathway plays a central role in signal transduction in organisms. It is involved in several biological processes, such as cellular metabolism, proliferation, and angiogenesis (60–62). It plays a crucial role in the development and progression of tumors (Figure 1) (63). PI3K consists of the regulatory subunit p85α and the catalytic subunit p110α, functioning as an intracellular phosphoinositide kinase (64). Activated PI3K converts phosphatidylinositol-4,5-bisphosphate (PIP2) into triphosphoinositide (PIP3) in the plasmalemma (65, 66). PIP3, a second messenger, recruits 3-phosphoinositide-dependent protein kinase 1 (PDK1), Akt, and serum- and glucocorticoid-regulated kinase to the plasma membrane (67–69).
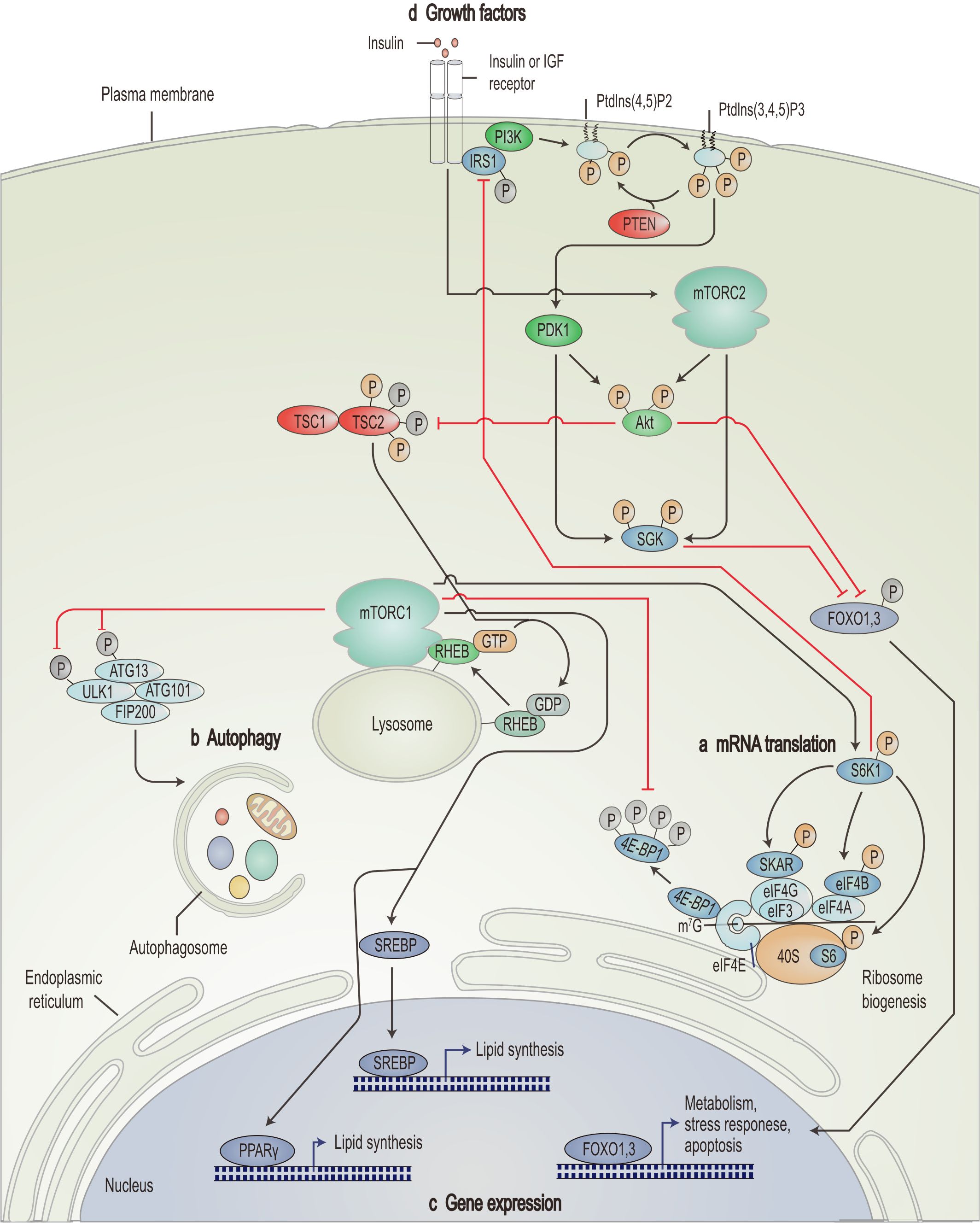
Figure 1. Schematic overview of the PI3K/AKT/mTOR signaling pathway. mTORC1 promotes mRNA translation (A), inhibits autophagy (B), promotes lipid synthesis-related genes and represses expression of apoptosis-related genes (C) through nutrient signals generated by growth factors such as insulin and insulin-like growth factor (D). IGF, insulin-like growth factor; Ptdlns(4,5)P2, phosphatidylinositol-4,5-bisphosphate; Ptdlns(3,4,5)P3, triphosphoinositide; IRS1, insulin receptor substrate 1; SGK, serum- and glucocorticoid-regulated kinase; FOXO, forkhead box protein O; RHEB, Ras homolog enriched in brain; ATG, autophagy-related; ULK1, UNC51-like kinase 1; FIP200, 200 kDa FAK family kinase-interacting protein; eIF, eukaryotic translation initiation factor; 4E-BP1, eIF4E-binding protein 1; S6K1, ribosomal S6 kinase; SKAR, S6K1 Aly/REF-like target; SREBP, sterol regulatory element-binding protein; PPARγ, peroxisome proliferator-activated receptor-γ. [Adapted from Zoncu et al. (139)].
Akt, a central element of the PI3K/AKT/mTOR pathway, can be completely activated dependent on two critical phosphorylation sites (70–73). The phosphorylation of Thr308 by PDK1 partly activates Akt, whereas mTORC2 phosphorylation of Ser473 fully activates Akt (74, 75). Once activated, Akt phosphorylates multiple downstream targets, such as tuberous sclerosis complex (TSC) 2 and forkhead box protein O, thereby directly or indirectly affecting cell growth and survival (76, 77).
mTOR is another crucial target of Akt. It is a highly conserved serine/threonine kinase (78), which forms two mTOR complexes, mTORC1 and mTORC2, by recruiting other proteins and active factors (79). Activation of mTORC1 relies on the phosphorylation of intracellular proteins, such as TSC (80). TSC possesses two subunits: TSC1 (hamartin) and TSC2 (tuberin) (81). Akt phosphorylates TSC2 and inhibits the negative regulatory effect of the TSC1-TSC2 complex on Ras homologue enriched in the brain, thereby activating mTORC1 (82). Once activated, mTORC1 also affects the phosphorylation of ribosomal S6 kinase (S6K1, p70S6k) and 4EBP1, further enhancing related gene expression and translation (Figure 1A). Besides, mTORC1 inhibits autophagy (Figure 1B), stimulates genes involved in lipogenesis (Figure 1C), and regulates apoptosis (Figure 1D), facilitating the rapid proliferation of cancer cells (83–86). mTORC1 effectively enhances angiogenesis by regulating hypoxia-inducible factor 1 alpha (87).
Phosphatase and tensin homologue (PTEN)-mediated hydrolysis of PIP3 to PIP2 and P70S6K-induced phosphorylation of insulin receptor substrate 1 (IRS1) negatively controls the PI3K/AKT/mTOR pathway (88, 89).
2.2 The involvement of the PI3K/AKT/mTOR pathway in the development and progression of PTs
Extensive research has been conducted on the alterations of the PI3K/AKT/mTOR pathway components in PTs (90). In a study involving 53 PAs, growth hormone-secreting pituitary adenomas (GH-PAs) (10/14, 71%) and non-functional pituitary adenomas (NFPAs) (11/33, 33%) showed a significant association with the mTOR pathway, compared to the control group (1/5, 20%) (91).
PIK3CA encodes the PI3K protein p110 subunit, which shows marked amplification and mutation in PTs. Murat et al. (92) found that 12.1% of PTs harbor PIK3CA mutations, whereas 21.2% of cases show gene amplification. Lin et al. (93) reported that PIK3CA mutations can be found in 9% of APTs, with 20%-40% of PTs displaying PIK3CA amplification. Another study showed that compared to normal pituitary tissue, the expression and phosphorylation levels of AKT and mTOR are elevated in PTs, whereas the expression level of PTEN is decreased (94).
The downstream effectors of mTOR, pS6, and eukaryotic translation initiation factor 4E (eIF4E) have been widely investigated in PTs. Compared to normal pituitary tissue, the pS6/eIF4E is more often activated in PTs (33%-71% vs. 20%) (95). In mice carrying mutations predisposing to PTs, the unregulated overexpression of p70S6k/S6 in PTs tissues compared to adjacent brain tissue (96). Dworakowska et al. (97) found no significant differences in the expression of p-mTOR, total mTOR, TSC2, and p70S6K between PTs and the control group; However, they did find the expression of c-MYC, a target of AKT and an oncogene, is elevated in PTs.
The mTOR pathway and its regulators are significantly linked to PTs invasion, staging, and tumor growth, and provide important predictive and prognostic value for PTs patients (98). Cai et al. (94) found that invasive PTs exhibit higher levels of AKT and lower levels of PTEN compared to non-invasive PTs. Further study of 95 PTs revealed that the expression of mTOR regulation-related proteins was negatively correlated with cavernous sinus invasion (98). Zhang et al. (99) discovered that lactate secreted by PTs cells promotes the polarization of tumor-associated macrophages via the mTORC2 signaling pathway, thereby enhancing the release of CCL17. This event promotes the invasion of PTs cells through the CCL17/CCR4/mTORC1 axis. It is worth noting that another study assessed the relationship between mTOR activity and PTs size, volume, Ki-67%, Knosp grade, and expression of somatostatin receptors, but found no significant correlations (95). This variability may be attributable to differences in sample size, experimental methods, and the heterogeneity of study subjects. To better understand the role of the mTOR pathway in PTs, larger studies encompassing various stages, degrees of invasion, and molecular subtypes are required.
Notably, there are variations in the PI3K/AKT/mTOR pathway activity among different subtypes of PTs. Analysis of 53 pituitary samples, including GH-PAs, NFPAs, and ACTH-secreting pituitary adenomas (ACTH-PAs), revealed elevated mTOR activity (estimated by the pS6/eIF4E ratio). GH-PAs exhibited the highest mTOR pathway activity, followed by NFPAs, while ACTH-PAs showed lower mTOR pathway activity (95). In the future, it is necessary to further explore the differences in mTOR pathway activity among various PTs subtypes, which is important for the development of individualized therapeutic regimens. Additionally, further studies on molecular mechanisms are needed to elucidate the factors contributing to the low mTOR pathway activity in ACTH-PAs.
2.3 The anti-tumor mechanisms of EVE
There is an intricate mechanism underlying the anti-tumor effects of EVE. EVE binds to FK506 binding protein 12 in mTORC1 and the FKBP-rapamycin binding domain, thereby inhibiting the activity of mTORC1 and its downstream molecules, blocking mRNA translation, leading to cell cycle arrest in the G1 phase, and enhancing tumor cell apoptosis (100). In addition, EVE induces the dissociation of raptor from mTORC1, preventing the phosphorylation of raptor by S6K1 and 4EBP1, thereby inhibiting protein synthesis and transcription (100). Moreover, EVE effectively induces the formation of autophagosomes in tumor cells, thereby increasing the LC3II/I and Beclin 1 expression, decreasing p62 expression, and potentiating autophagy (101). EVE inhibits tumor angiogenesis by directly targeting the mTOR in vascular endothelial cells, thereby suppressing their proliferation (102). Additionally, EVE decreases the synthesis of angiogenesis-promoting factors induced by hypoxia-inducible factors (102). Some studies have shown that in addition to the AKT-dependent pathway, EVE can activate mTOR through non-AKT pathways, such as Ras/MEK/ERK, thus exhibiting antitumor effects (103).
3 Current research status of EVE in PTs
After searching databases including pubmed, web of science, embase, and scopus, we included 12 studies from six countries on EVE treatment of PTs. These studies covered cellular, animal and human levels, with interventions including EVE monotherapy and combination therapies. Detailed data on EVE treatment for PTs are presented in Table 1. This paper provides a comprehensive review and discussion of previous studies across cellular, animal, and human levels.
3.1 Cellular level
EVE has been extensively studied for its efficacy in treating PTs. Gorshtein et al. (104) first demonstrated that EVE inhibits the phosphorylation of p70S6K by blocking the mTOR pathway, thereby inducing G0/G1 cell cycle arrest and decreasing the survival of GH3 cells. Another study observed that EVE may inhibit IGF-1-induced GH3 cell survival and suppress GH secretion via the PI3K/Akt/mTOR pathway. Zatelli et al. (105) further support these findings. They applied different concentrations of EVE (1 nM-1 μM) to the primary cultures of human NFPAs, revealing that EVE inhibited p70S6K activity (-20%), reduced cell viability (70%), promoted apoptosis (+30%), and inhibited the proliferative and anti-apoptotic effects of IGF-1. Regazzo et al. (106) found that EVE can significantly decrease the survival of quiescent gonadotroph adenoma cells, further validating its potential for widespread application in different subtypes of PTs.
To improve the efficacy of EVE monotherapy and mitigate resistance, researchers have started exploring its combination with additional drugs or therapeutic strategies. Previous studies have indicated that the combination of EVE with Torin1 can markedly decrease the viability of MtT/E pituitary cells and human-derived NFPAs cells and the expression of cyclin D3 and p21, surpassing the effects of single-agent therapy (107). Compared to EVE monotherapy, EVE combined with pasireotide yielded similar cumulative effects in NFPAs cells (105). EVE combined with PI3K inhibitors (PI3Ki) exerted synergistic effects on cell and colony survival in rat GH3 and human GH-PAs cell lines, which may be attributed to the fact that PI3Ki enhanced the anti-tumor effect of EVE by modulating the PI3K/Akt/mTOR and MAPK pathways (108). However, some studies indicate that EVE combined with cabergoline (CAB) showed only additive effects in inhibiting PRL secretion, without significant synergistic effects on tumor cell proliferation (53). Moreover, EVE had been shown to enhance the sensitivity of GH3 cell lines to radiotherapy (109). These studies offer new insights into EVE combination therapy for treating PTs, promising more effective and personalized options. However, despite its significant anti-tumor effects, the therapy’s effectiveness depends on factors like drugs choice, cell line specificity, and treatment stage. Future research should explore optimal combinations, focusing on the mechanisms of combined therapy and validating their feasibility and efficacy through clinical trials.
Recent studies have revealed key findings regarding the role of EVE combination therapy in overcoming PTs resistance. Studies have shown that nearly 64% of primary cultured NFPAs cells are resistant to EVE (90), often associated with a significant increase in p-AKT (110) and p-AKT/total-AKT ratio (90). Researchers have tried EVE in combination with drugs like CAB (90) or Torin1 (107), which has proven effective in attenuating EVE-induced Akt-Ser473 phosphorylation levels and reversing approximately 78% of the resistance (90). However, another study presented an alternative view, showing that EVE combined with Gleevec may further activate p-AKT and exacerbate drug resistance. These findings highlight the complexity and challenges in selecting combination therapy strategies, suggesting that the treatments effectiveness may vary with patient populations or specific drug combinations. Further studies on molecular mechanisms are needed to clarify the underlying biological basis.
3.2 Animal level
There are four main types of animal models used in PTs research: cell line-derived xenografts (CDX), patient-derived xenografts (PDX), environmentally induced models, and genetically engineered mouse (GEM) models (63). Although CDX and in vitro cultured animal-derived cell lines are commonly used in pituitary research, their limitations, including the loss of genetic heterogeneity in CDX and differences from the human tumor microenvironment, limit their suitability for long-term studies. Conversely, the use of PDX models is relatively limited. Currently, there are no commercially available human PTs cell lines, making the cultivation of primary human-derived PTs cells challenging and limited to short-term studies. Additionally, while rodent cell lines are more accessible, their pathophysiological characteristics differ from those of humans, posing challenges for developing models that accurately reflect human biology. Emerging techniques, like pituitary induction using human induced pluripotent stem cells, show promise for creating reliable human PTs models. These methods aim to cultivate cell lines with human PTs characteristics in vitro, potentially overcoming current model limitations. Future exploration of these approaches may significantly advance PTs diagnosis and treatment.
Jalali et al. (111) established xenograft mouse models employing GH4C1 cell lines with different FGFR4 genotypes (wild-type G388, polymorphic R388, and parental controls) to assess tumor growth rates and Ki-67 expression. They then analyzed the mTOR pathway activation (p-S6 and p-4EBP1) and administered EVE to assess its effect on tumor growth. They observed that tumors with G388 and R388 genotypes grew faster than parental controls, and showed increased Ki-67 expression. These findings suggest that the FGFR4 genotype may accelerate tumor growth by enhancing cell proliferation. Further research revealed significantly increased p-S6 and p-4EBP1 activity in tumors with G388 and R388 genotypes. EVE treatment reduced p-S6 levels across all genotypes, suggesting that EVE may effectively inhibit tumor growth by suppressing the mTOR pathway (111). This study uses animal models to explore the impact of FGFR4 genotypes on PTs growth rates and the involvement of the mTOR pathway, while also assessing EVE’s potential in slowing intracranial tumor growth. The findings improve understanding of PTs biology and support the development of new treatment strategies. Given the effect of PTs genotypes on EVE efficacy, exploring personalized EVE treatments for different genotypes could be highly significant.
3.3 Human level
Previous clinical studies on EVE treatment for PTs have mainly focused on patients with APTs, with most literature being case reports. Several studies suggest that EVE partially inhibits APTs growth and improves clinical symptoms. Zhang et al. (53) reported a patient with PRL-PAs who were resistant to standard treatments, such as CAB. The addition of EVE to CAB markedly decreased PRL levels and tumor regression. Additionally, Lin et al. (54) described four patients with aggressive PRL-PAs, three of whom finally achieved sustained stabilization of their disease and their PRL levels decreased after adding EVE to CAB therapy. Of the four patients, two discontinued TMZ and pasireotide before adding EVE (patients 2 and 3). Jouanneau et al. (112) found that neither EVE monotherapy nor its combination with octreotide can effectively reduce tumor growth or ACTH secretion. This may be attributed to the weak activation of the mTOR pathway in ACTH-PAs. However, Donovan et al. (113) found that patients with ACTH-PAs harboring STK11 mutations respond better to EVE. This suggests that when identifying patients who may be suitable for treatment with EVE, it is essential to consider not only the mTOR activity of PTs but also other factors that may affect patients’ sensitivity to EVE, such as mutations of specific genes, levels of biological markers (114), and the degree of tumor differentiation (115).
In summary, although EVE has demonstrated potential therapeutic effects in the preclinical studies of PTs, data on its efficacy as a treatment in the clinical management of PTs remain limited. The exact efficacy of EVE in clinical practice is still unclear and it is not known whether EVE should be added to ineffective first-line treatment options (e.g.CAB, pasireotide, etc.) or whether current first-line treatments should be discontinued before initiating EVE monotherapy or combination therapy. Future prospective, multicenter clinical trials are needed to explore these issues.
4 Molecular mechanisms of EVE in Other NETs
Given the limited data on EVE in PAs, we elucidated its mechanism of action by investigating its effects in other NETs. The PI3K/Akt/mTOR pathway plays a crucial role in the development of NETs. French researchers Boilève et al. (116) conducted a retrospective analysis of real-world data on precision treatment for patients with NETs, revealing that the mTOR pathway is the most frequently altered pathway in these patients (24%). Downregulation of the PTEN and TSC2 genes in the PI3K/Akt/mTOR pathway is significantly associated with shorter disease-free survival and overall survival in patients with NETs (117).
EVE exerts its anti-NET effects through several mechanisms. Firstly, EVE exerts an inhibitory effect on the proliferation of NET cells (118, 119). In vitro and in vivo experiments, EVE, either alone or in combination with cytotoxic agents, can block the growth and proliferation of NETs cells, primarily by inhibiting protein synthesis (120, 121). Zitzmann et al. (118) treated BON cells, a human cell line of pancreatic NETs with constitutive activation of the PI3K/AKT/mTOR signaling pathway, with various concentrations of EVE and found that it inhibited the growth of BON cells in a dose-dependent manner. Grozinsky-Glasberg et al. (119) investigated the effects of octreotide, EVE, and their combination on cell proliferation and kinase activation in NET cell lines (the rat insulinoma cell line, INS1), finding that both octreotide and EVE inhibited cell proliferation and reduced the phosphorylation of Akt downstream targets (TSC2, mTOR, and p70S6K).
Secondly, EVE exerts anti-angiogenic effects on NETs. NETs are among the most vascularized tumors discovered to date. In the phase III RADIANT-3 clinical trial, Yao et al. (122) analyzed the levels of vascular endothelial growth factor (VEGF), platelet-derived growth factor (PGF), basic fibroblast growth factor, soluble vascular endothelial growth factor receptor-1 (sVEGFR-1), and soluble vascular endothelial growth factor receptor-2 (sVEGFR-2) in patients before and after treatment with EVE. They found that EVE significantly reduced the levels of sVEGFR-2 and PGF compared to placebo. Furthermore, EVE, in combination with somatostatin analogs, demonstrated a synergistic anti-angiogenic effect, whereby EVE reduced the production of VEGF by tumor cells by inhibiting the mTOR-HIF-1α pathway, while somatostatin analogs acted directly or indirectly on stromal endothelial cells and monocytes expressing somatostatin receptors (123).
Additionally, EVE induced cell cycle arrest and apoptosis in NETs while promoting autophagy. EVE reduced the phosphorylation levels of downstream targets of the PI3K/AKT pathway, including TSC2, mTOR, and p70S6K, leading to cell cycle arrest in the G0/G1 phase and inducing apoptosis (118). Furthermore, EVE affects autophagy by downregulating the AKT signaling pathway. mTORC1 inhibits autophagosome formation and initiation of autophagy by phosphorylating UNC51-like kinase 1 (ULK1); however, the mTOR inhibitor EVE can suppress mTORC1 and prevent the phosphorylation of ULK1, thereby accelerating autophagy (124). Histopathological studies indicate that the combination of chloroquine and EVE can significantly inhibit mTOR activity and the growth of NETs, while simultaneously suppressing the accumulation of autophagosomes and increasing apoptosis (124).
In summary, EVE exhibits multiple mechanisms of action in NETs, including inhibition of cell proliferation and angiogenesis, induction of cell cycle arrest and apoptosis, and promotion of autophagy. Future studies should investigate the combinatorial effects of EVE with other therapeutic modalities to improve treatment outcomes for patients with NETs.
5 Blood-brain barrier permeability of EVE
Chemotherapeutic agents’ permeability through the BBB directly affects their efficacy. EVE, a lipophilic compound, shows considerable BBB permeability and promising therapeutic effects against central nervous system tumors (125). EVE can modulate efflux mechanisms mediated by breast cancer resistance protein (BCRP) and P-glycoprotein (P-gp), improving its passage through BBB (126–128). Researchers have developed several drug delivery systems, such as osmotic pumps (129), convection-enhanced delivery (130), and interstitial therapies (131), to implant EVE directly into the tumor tissue or surrounding stroma, effectively bypassing the BBB. However, the effectiveness of these methods can be affected by factors in the tumor microenvironment, such as the extracellular matrix and brain’s lymphatic drainage system, which may restrict drug distribution and retention. To address this challenge, Han et al. (132) optimized drug distribution and retention in brain tumors using liposomal formulations. They developed liposomes with varying surface charges, PEGylation, and transition temperatures, and evaluated them for in vitro cellular uptake, distribution, and persistence in brain tissue. The study found that PEGylated liposomes with positive charges and high transition temperatures, especially EVE liposomes, showed significantly improved interstitial therapeutic efficacy for intracranial tumors. Future studies should focus on elucidating the mechanisms underlying EVE passage through BBB to optimize drug delivery strategies and enhance its clinical application in intracranial tumors.
6 Safety of EVE
Stomatitis, hyperlipidemia, and hyperglycemia are the most common adverse events associated with EVE (incidence rate ≥1/10), whereas severe adverse events (≥grade 3) are relatively rare (133). The incidence of adverse events associated with EVE is closely correlated with its blood concentration. In one study, when the peak concentration (Cmin) of EVE was <7.8 ng/mL and ≥7.8 ng/mL, 9%, and 14%-19% of patients experienced adverse reactions (134), respectively. Another study reported that for each two-fold increase in the Cmin of EVE, the risk of severe adverse events increased by 1.5, whereas reducing the dose of EVE allowed patients to recover (135). A meta-analysis indicated that the risk of pulmonary adverse events significantly increases when the Cmin of EVE exceeds 30 ng/mL (136). The blood concentration of EVE is considerably higher in patients experiencing dose-limiting toxicities (DLTs) than in those without toxicities (137). The cumulative incidence of DLTs significantly increased (HR: 4.87, 95% CI: 1.53-15.5) (138). There are significant variations in pharmacokinetic parameters of EVE among different individuals, such as clearance rates (range: 5.1-21.3 L/h/70 kg, coefficient of variation: 38.5%) and central distribution volume (range: 9.9-103.6 L/70 kg, coefficient of variation: 57.8%) (137). Therefore, blood drug concentrations should be monitored in patients receiving EVE treatment to ensure that their Cmin remains within a safe range, enabling the early detection of adverse events and appropriate medical management.
7 Conclusion and prospect
The PI3K/AKT/mTOR pathway is overactivated in PTs. EVE, a selective kinase inhibitor, directly and durably inhibits mTOR, demonstrating vigorous anti-PTs effects. Previous studies have validated the therapeutic efficacy of EVE against PTs across cellular, animal, and human-level.
Although EVE shows potential in treating PTs, several issues remain. First, while low mTOR pathway activity has been observed in ACTH-PAs, the precise molecular mechanisms are unclear. Further research is required to identify specific mutations or regulatory mechanisms within this pathway in ACTH-PAs and their impact on EVE’s efficacy. Additionally, since EVE was recently approved, existing studies mainly address short-term outcomes and lack long-term follow-up data. Future efforts should involve large-scale, multi-center studies to evaluate EVE’s long-term efficacy and safety. Furthermore, no definitive biomarkers for treatment-resistant PTs have been identified, complicating early detection of APTs. Research should focus on discovering early predictive biomarkers and treatment response indicators for resistant PTs, as well as defining optimal treatment duration, sequencing, and combinations for personalized strategies. Given the rarity and heterogeneity of APTs, clinical management experience has accumulated slowly. Establishing national and international registries could improve understanding and management of APTs in the future.
Author contributions
ZY: Software, Writing – original draft. HC: Writing – review & editing.
Funding
The author(s) declare that financial support was received for the research, authorship, and/or publication of this article. This study was supported by the National Natural Science Foundation project of China (C031002), Gansu Provincial Science Foundation of China (21JR7RA416) and Lanzhou University Second Hospital's "Cuiying Technology Innovation" Program (CY2018-ZD02).
Acknowledgments
We thank all the participants for their contribution to this study.
Conflict of interest
The authors declare that the research was conducted in the absence of any commercial or financial relationships that could be construed as a potential conflict of interest.
Publisher’s note
All claims expressed in this article are solely those of the authors and do not necessarily represent those of their affiliated organizations, or those of the publisher, the editors and the reviewers. Any product that may be evaluated in this article, or claim that may be made by its manufacturer, is not guaranteed or endorsed by the publisher.
Glossary
ACTH: Adrenocorticotropic hormone
ACTH-PAs: ACTH-secreting pituitary adenoma
ATG: Autophagy-related
APTs: Aggressive Pituitary Tumors
BBB: Blood-Brain Barrier
BCRP: Breast Cancer Resistance Protein
CAB: Cabergoline
CDX: Cell line-Derived Xenografts
Cmin: Peak concentration
DLTs: Dose-limiting toxicities
eIF: Eukaryotic translation initiation factor
EVE: Everolimus
FDA: Food and Drug Administration
FIP200: 200 kDa FAK family kinase-interacting protein
FOXO: Forkhead box protein O
FPAs: Functional pituitary adenomas
GEM: Genetically Engineered Mouse
GH: Growth Hormone
GH-Pas: Growth Hormone-Secreting Pituitary Adenomas
IGF1: Insulin-like growth factor 1
IRS1: Insulin receptor substrate 1
NETs: Neuroendocrine Tumors
NFPAs: Non-functional pituitary adenomas
PTs: Pituitary Tumors
PDK1: 3-phosphoinositide-dependent protein kinase 1
PDX: Patient-Derived Xenografts
PGF: Platelet-derived Growth Factor
P-gp: P-glycoprotein
PI3Ki: PI3K inhibitor
PIP2: Phosphatidylinositol-4,5-bisphosphate
PIP3: Triphosphoinositide
PPARγ: Peroxisome proliferator-activated receptor-γ
PRL: Prolactin
PRL-Pas: Prolactinomas
PTEN: Phosphatase and tensin homologue
RHEB: Ras homologue enriched in the brain
S6K1/p70S6K: Ribosomal S6 kinase
SKAR: S6K1 Aly/REF-like target
SOM230: Pasireotide
SREBP: Sterol regulatory element-binding protein
sVEGFR-1: soluble Vascular Endothelial Growth Factor Receptor-1
sVEGFR-2: soluble Vascular Endothelial Growth Factor Receptor-2
TMZ: Temozolomide
TSC: Tuberous Sclerosis Complex
4EBP1: Eukaryotic translation initiation factor 4E-binding protein 1
ULK1: UNC51-like kinase 1
VEGF: Vascular Endothelial Growth Factor
References
1. Li Y, Ren X, Gao W, Cai R, Wu J, Liu T, et al. The biological behavior and clinical outcome of pituitary adenoma are affected by the microenvironment. CNS Neurosci Ther. (2024) 30:e14729. doi: 10.1111/cns.14729
2. Tritos NA, Miller KK. Diagnosis and management of pituitary adenomas: A review. JAMA. (2023) 329:1386–98. doi: 10.1001/jama.2023.5444
4. Uygur MM, di Filippo L, Giustina A. New tools for bone health assessment in secreting pituitary adenomas. Trends Endocrinol Metab. (2023) 34:231–42. doi: 10.1016/j.tem.2023.01.006
5. Lin AL, Rudneva VA, Richards AL, Zhang Y, Woo HJ, Cohen M, et al. Genome-wide loss of heterozygosity predicts aggressive, treatment-refractory behavior in pituitary neuroendocrine tumors. Acta Neuropathol. (2024) 147:85. doi: 10.1007/s00401-024-02736-8
6. Kolitz T, Greenman Y. Refractory nonfunctioning pituitary adenomas. Pituitary. (2023) 26:278–80. doi: 10.1007/s11102-023-01298-4
7. Geer EB. Refractory pituitary adenomas: preface. Pituitary. (2023) 26:261–2. doi: 10.1007/s11102-023-01330-7
8. Molitch ME. Diagnosis and treatment of pituitary adenomas: A review. JAMA. (2017) 317:516–24. doi: 10.1001/jama.2016.19699
9. Khan DZ, Hanrahan JG, Baldeweg SE, Dorward NL, Stoyanov D, Marcus HJ. Current and future advances in surgical therapy for pituitary adenoma. Endocr Rev. (2023) 44:947–59. doi: 10.1210/endrev/bnad014
10. Osorio RC, Oh JY, Choudhary N, Lad M, Savastano L, Aghi MK. Pituitary adenomas and cerebrovascular disease: A review on pathophysiology, prevalence, and treatment. Front Endocrinol (Lausanne). (2022) 13:1064216. doi: 10.3389/fendo.2022.1064216
11. De Sousa SMC, Lenders NF, Lamb LS, Inder WJ, McCormack A. Pituitary tumours: molecular and genetic aspects. J Endocrinol. (2023) 257(3):e220291. doi: 10.1530/joe-22-0291
12. MacFarlane J, Huynh KA, Powlson AS, Kolias AG, Mannion RJ, Scoffings DJ, et al. Novel imaging techniques in refractory pituitary adenomas. Pituitary. (2023) 26:288–92. doi: 10.1007/s11102-023-01304-9
13. Liu X, Dai C, Bao X, Deng K, Yao Y, Feng M, et al. The clinical and pathological characteristics of refractory pituitary adenomas: A single center experience. Front Oncol. (2022) 12:846614. doi: 10.3389/fonc.2022.846614
14. Pease M, Cohen MA, Tabar V. Advances in surgical approaches for refractory pituitary adenomas. Pituitary. (2023) 26:293–7. doi: 10.1007/s11102-023-01318-3
15. Wang R, Zhou C, McCormack AI, Mamelak AN. Editorial: refractory pituitary adenoma-current challenges and emerging treatments. Front Endocrinol (Lausanne). (2022) 13:868174. doi: 10.3389/fendo.2022.868174
16. Ilie MD, Vasiljevic A, Bertolino P, Raverot G. Biological and therapeutic implications of the tumor microenvironment in pituitary adenomas. Endocr Rev. (2023) 44:297–311. doi: 10.1210/endrev/bnac024
17. McCormack A, Dekkers OM, Petersenn S, Popovic V, Trouillas J, Raverot G, et al. Treatment of aggressive pituitary tumours and carcinomas: results of a European society of endocrinology (Ese) survey 2016. Eur J Endocrinol. (2018) 178:265–76. doi: 10.1530/eje-17-0933
18. Burman P, Trouillas J, Losa M, McCormack A, Petersenn S, Popovic V, et al. Aggressive pituitary tumours and carcinomas, characteristics and management of 171 patients. Eur J Endocrinol. (2022) 187:593–605. doi: 10.1530/eje-22-0440
19. González-Virla B, Vargas-Ortega G, Romero-Gameros CA. Radiotherapy and mortality in pituitary adenomas. Arch Med Res. (2023) 54:102900. doi: 10.1016/j.arcmed.2023.102900
20. Kowalchuk RO, Trifiletti DM, Brown PD, Sheehan JP. Contemporary radiotherapy and radiosurgery techniques for refractory pituitary adenomas. Pituitary. (2023) 26:298–302. doi: 10.1007/s11102-023-01300-z
21. Combs SE, Baumert BG, Bendszus M, Bozzao A, Brada M, Fariselli L, et al. Estro acrop guideline for target volume delineation of skull base tumors. Radiother Oncol. (2021) 156:80–94. doi: 10.1016/j.radonc.2020.11.014
22. Hamblin R, Vardon A, Akpalu J, Tampourlou M, Spiliotis I, Sbardella E, et al. Risk of second brain tumour after radiotherapy for pituitary adenoma or craniopharyngioma: A retrospective, multicentre, cohort study of 3679 patients with long-term imaging surveillance. Lancet Diabetes Endocrinol. (2022) 10:581–8. doi: 10.1016/s2213-8587(22)00160-7
23. Geer EB. Medical therapy for refractory pituitary adenomas. Pituitary. (2023) 26:303–6. doi: 10.1007/s11102-023-01320-9
24. Dai C, Liang S, Sun B, Li Y, Kang J. Anti-vegf therapy in refractory pituitary adenomas and pituitary carcinomas: A review. Front Oncol. (2021) 11:773905. doi: 10.3389/fonc.2021.773905
25. Ilie MD, Jouanneau E, Raverot G. Aggressive pituitary adenomas and carcinomas. Endocrinol Metab Clin North Am. (2020) 49:505–15. doi: 10.1016/j.ecl.2020.05.008
26. Du Four S, van der Veken J, Duerinck J, Vermeulen E, Andreescu CE, Bruneau M, et al. Pituitary carcinoma - case series and review of the literature. Front Endocrinol (Lausanne). (2022) 13:968692. doi: 10.3389/fendo.2022.968692
27. Das L, Gupta N, Dutta P, Walia R, Vaiphei K, Rai A, et al. Early initiation of temozolomide therapy may improve response in aggressive pituitary adenomas. Front Endocrinol (Lausanne). (2021) 12:774686. doi: 10.3389/fendo.2021.774686
28. Petersenn S. Medical therapy of aggressive pituitary tumors. Exp Clin Endocrinol Diabetes. (2021) 129:186–93. doi: 10.1055/a-1331-6939
29. Liu GY, Sabatini DM. Mtor at the nexus of nutrition, growth, ageing and disease. Nat Rev Mol Cell Biol. (2020) 21:183–203. doi: 10.1038/s41580-019-0199-y
30. Saxton RA, Sabatini DM. Mtor signaling in growth, metabolism, and disease. Cell. (2017) 168:960–76. doi: 10.1016/j.cell.2017.02.004
31. Bielska AA, Harrigan CF, Kyung YJ, Morris Q, Palm W, Thompson CB. Activating mtor mutations are detrimental in nutrient-poor conditions. Cancer Res. (2022) 82:3263–74. doi: 10.1158/0008-5472.Can-22-0121
32. Malvi P, Chava S, Cai G, Hu K, Zhu LJ, Edwards YJK, et al. Hoxc6 drives a therapeutically targetable pancreatic cancer growth and metastasis pathway by regulating Msk1 and ppp2r2b. Cell Rep Med. (2023) 4:101285. doi: 10.1016/j.xcrm.2023.101285
33. Prosseda PP, Dannewitz Prosseda S, Tran M, Liton PB, Sun Y. Crosstalk between the mtor pathway and primary cilia in human diseases. Curr Top Dev Biol. (2023) 155:1–37. doi: 10.1016/bs.ctdb.2023.09.004
34. Deng X, Ning Z, Li L, Cui Z, Du X, Amevor FK, et al. High expression of Mir-22-3p in chicken hierarchical follicles promotes granulosa cell proliferation, steroidogenesis, and lipid metabolism via Pten/Pi3k/Akt/Mtor signaling pathway. Int J Biol Macromol. (2023) 253:127415. doi: 10.1016/j.ijbiomac.2023.127415
35. Shi XF, Yu Q, Wang KB, Fu YD, Zhang S, Liao ZY, et al. Active ingredients isorhamnetin of croci srigma inhibit stomach adenocarcinomas progression by Mapk/Mtor signaling pathway. Sci Rep. (2023) 13:12607. doi: 10.1038/s41598-023-39627-z
36. Xu K, He Y, Moqbel SAA, Zhou X, Wu L, Bao J. Sirt3 ameliorates osteoarthritis via regulating chondrocyte autophagy and apoptosis through the Pi3k/Akt/Mtor pathway. Int J Biol Macromol. (2021) 175:351–60. doi: 10.1016/j.ijbiomac.2021.02.029
37. Zheng X, Li W, Xu H, Liu J, Ren L, Yang Y, et al. Sinomenine ester derivative inhibits glioblastoma by inducing mitochondria-dependent apoptosis and autophagy by Pi3k/Akt/Mtor and Ampk/Mtor pathway. Acta Pharm Sin B. (2021) 11:3465–80. doi: 10.1016/j.apsb.2021.05.027
38. Sun W, Lei Y, Jiang Z, Wang K, Liu H, Xu T. Bpa and low-se exacerbate apoptosis and mitophagy in chicken pancreatic cells by regulating the Pten/Pi3k/Akt/Mtor pathway. J Adv Res. (2024). doi: 10.1016/j.jare.2024.01.029
39. Li Z, Zhou H, Zhai X, Gao L, Yang M, An B, et al. Melk promotes hcc carcinogenesis through modulating cuproptosis-related gene dlat-mediated mitochondrial function. Cell Death Dis. (2023) 14:733. doi: 10.1038/s41419-023-06264-3
40. Sun RX, Zhu HJ, Zhang YR, Wang JN, Wang Y, Cao QC, et al. Alkbh5 Causes retinal Pigment epithelium anomalies and Choroidal Neovascularization in Age-Related Macular Degeneration Via the Akt/Mtor Pathway. Cell Rep. (2023) 42:112779. doi: 10.1016/j.celrep.2023.112779
41. Zhou C, Liu C, Liu W, Chen W, Yin Y, Li CW, et al. Slfn11 inhibits hepatocellular carcinoma tumorigenesis and metastasis by targeting Rps4x via Mtor pathway. Theranostics. (2020) 10:4627–43. doi: 10.7150/thno.42869
42. Wang G, Qin S, Chen L, Geng H, Zheng Y, Xia C, et al. Butyrate dictates ferroptosis sensitivity through ffar2-mtor signaling. Cell Death Dis. (2023) 14:292. doi: 10.1038/s41419-023-05778-0
43. Asghari F, Karimi MH, Pourfathollah AA. Mtorc1 inhibition may improve T lymphocytes affected by aging. Immunopharmacol Immunotoxicol. (2023) 45(6):719–29. doi: 10.1080/08923973.2023.2232101
44. Ryan CW, Tangen CM, Heath EI, Stein MN, Meng MV, Alva AS, et al. Adjuvant everolimus after surgery for renal cell carcinoma (Everest): A double-blind, placebo-controlled, randomised, phase 3 trial. Lancet. (2023) 402:1043–51. doi: 10.1016/s0140-6736(23)00913-3
45. Stähli BE, Klingenberg R, Heg D, Branca M, Manka R, Kapos I, et al. Mammalian target of rapamycin inhibition in patients with st-segment elevation myocardial infarction. J Am Coll Cardiol. (2022) 80:1802–14. doi: 10.1016/j.jacc.2022.08.747
46. Kato S, Cohen EEW. Did everolimus break the rules? Clin Cancer Res. (2021) 27:3807–8. doi: 10.1158/1078-0432.Ccr-21-1508
47. Sheng X, Ye D, Zhou A, Yao X, Luo H, He Z, et al. Efficacy and safety of vorolanib plus everolimus in metastatic renal cell carcinoma: A three-arm, randomised, double-blind, multicentre phase iii study (Concept). Eur J Cancer. (2023) 178:205–15. doi: 10.1016/j.ejca.2022.10.025
48. Xu J, Ye Z, Zhuo Q, Gao H, Qin Y, Lou X, et al. Men1 degradation induced by neddylation and the cul4b-dcaf7 axis promotes pancreatic neuroendocrine tumor progression. Cancer Res. (2023) 83:2226–47. doi: 10.1158/0008-5472.Can-22-3599
49. Del Rivero J, Perez K, Kennedy EB, Mittra ES, Vijayvergia N, Arshad J, et al. Systemic therapy for tumor control in metastatic well-differentiated gastroenteropancreatic neuroendocrine tumors: asco guideline. J Clin Oncol. (2023) 41:5049–67. doi: 10.1200/jco.23.01529
50. Zidan L, Iravani A, Oleinikov K, Ben-Haim S, Gross DJ, Meirovitz A, et al. Efficacy and safety of (177)Lu-dotatate in lung neuroendocrine tumors: A bicenter study. J Nucl Med. (2022) 63:218–25. doi: 10.2967/jnumed.120.260760
51. Mosalem O, Sonbol MB, Halfdanarson TR, Starr JS. Tyrosine kinase inhibitors and immunotherapy updates in neuroendocrine neoplasms. Best Pract Res Clin Endocrinol Metab. (2023) 37:101796. doi: 10.1016/j.beem.2023.101796
52. Shimon I. Prolactinomas resistant to dopamine agonists: pathophysiology and treatment. Arch Med Res. (2023) 54:102883. doi: 10.1016/j.arcmed.2023.102883
53. Zhang D, Way JS, Zhang X, Sergey M, Bergsneider M, Wang MB, et al. Effect of everolimus in treatment of aggressive prolactin-secreting pituitary adenomas. J Clin Endocrinol Metab. (2019) 104:1929–36. doi: 10.1210/jc.2018-02461
54. Lin AL, Geer EB, Lala N, Page-Wilson G, Magge R, Young RJ, et al. The treatment of aggressive prolactinomas with everolimus. Pituitary. (2023) 26:474–81. doi: 10.1007/s11102-023-01340-5
55. Toniato de Rezende Freschi J, Cristelli MP, Viana LA, Ficher KN, Nakamura MR, Proença H, et al. A head-to-head comparison of de novo sirolimus or everolimus plus reduced-dose tacrolimus in kidney transplant recipients: A prospective and randomized trial. Transplantation. (2023) 108(1):261–75. doi: 10.1097/tp.0000000000004749
56. Nelson BE, Roszik J, Janku F, Hong DS, Kato S, Naing A, et al. Braf V600e-mutant cancers treated with vemurafenib alone or in combination with everolimus, sorafenib, or crizotinib or with paclitaxel and carboplatin (Vem-plus) study. NPJ Precis Oncol. (2023) 7:19. doi: 10.1038/s41698-022-00341-0
57. Willems LM, Rosenow F, Schubert-Bast S, Kurlemann G, Zöllner JP, Bast T, et al. Efficacy, retention and tolerability of everolimus in patients with tuberous sclerosis complex: A survey-based study on patients' Perspectives. CNS Drugs. (2021) 35:1107–22. doi: 10.1007/s40263-021-00839-4
58. Slomovitz BM, Filiaci VL, Walker JL, Taub MC, Finkelstein KA, Moroney JW, et al. A randomized phase ii trial of everolimus and letrozole or hormonal therapy in women with advanced, persistent or recurrent endometrial carcinoma: A gog foundation study. Gynecol Oncol. (2022) 164:481–91. doi: 10.1016/j.ygyno.2021.12.031
59. Wu W, Chen J, Deng H, Jin L, He Z, Rao N, et al. Neoadjuvant everolimus plus letrozole versus fluorouracil, epirubicin and cyclophosphamide for Er-positive, Her2-negative breast cancer: A randomized pilot trial. BMC Cancer. (2021) 21:862. doi: 10.1186/s12885-021-08612-y
60. Song S, Zhang G, Chen X, Zheng J, Liu X, Wang Y, et al. Hif-1α Increases the osteogenic capacity of adscs by coupling angiogenesis and osteogenesis via the Hif-1α/Vegf/Akt/Mtor signaling pathway. J Nanobiotechnol. (2023) 21:257. doi: 10.1186/s12951-023-02020-z
61. Tewari D, Patni P, Bishayee A, Sah AN, Bishayee A. Natural products targeting the Pi3k-Akt-Mtor signaling pathway in cancer: A novel therapeutic strategy. Semin Cancer Biol. (2022) 80:1–17. doi: 10.1016/j.semcancer.2019.12.008
62. Li J, Jiang M, Yu Z, Xiong C, Pan J, Cai Z, et al. Artemisinin relieves osteoarthritis by activating mitochondrial autophagy through reducing tnfsf11 expression and inhibiting Pi3k/Akt/Mtor signaling in cartilage. Cell Mol Biol Lett. (2022) 27:62. doi: 10.1186/s11658-022-00365-1
63. Derwich A, Sykutera M, Bromińska B, Rubiś B, Ruchała M, Sawicka-Gutaj N. The role of activation of Pi3k/Akt/Mtor and Raf/Mek/Erk pathways in aggressive pituitary adenomas-new potential therapeutic approach-a systematic review. Int J Mol Sci. (2023) 24(13):10952. doi: 10.3390/ijms241310952
64. Wang JF, Wang YP, Xie J, Zhao ZZ, Gupta S, Guo Y, et al. Upregulated pd-L1 delays human neutrophil apoptosis and promotes lung injury in an experimental mouse model of sepsis. Blood. (2021) 138:806–10. doi: 10.1182/blood.2020009417
65. Shen Q, Han Y, Wu K, He Y, Jiang X, Liu P, et al. Mrgprf acts as a tumor suppressor in cutaneous melanoma by restraining pi3k/akt signaling. Signal Transduct Target Ther. (2022) 7:147. doi: 10.1038/s41392-022-00945-9
66. Yamasaki E, Ali S, Sanchez Solano A, Thakore P, Smith M, Wang X, et al. Faulty trpm4 channels underlie age-dependent cerebral vascular dysfunction in Gould syndrome. Proc Natl Acad Sci U.S.A. (2023) 120:e2217327120. doi: 10.1073/pnas.2217327120
67. Jiang Q, Zhang X, Dai X, Han S, Wu X, Wang L, et al. S6k1-mediated phosphorylation of Pdk1 impairs Akt kinase activity and oncogenic functions. Nat Commun. (2022) 13:1548. doi: 10.1038/s41467-022-28910-8
68. Levina A, Fleming KD, Burke JE, Leonard TA. Activation of the essential kinase Pdk1 by phosphoinositide-driven trans-autophosphorylation. Nat Commun. (2022) 13:1874. doi: 10.1038/s41467-022-29368-4
69. Leroux AE, Biondi RM. The choreography of protein kinase pdk1 and its diverse substrate dance partners. Biochem J. (2023) 480:1503–32. doi: 10.1042/bcj20220396
70. Yu L, Wei J, Liu P. Attacking the Pi3k/Akt/Mtor signaling pathway for targeted therapeutic treatment in human cancer. Semin Cancer Biol. (2022) 85:69–94. doi: 10.1016/j.semcancer.2021.06.019
71. Huang L, Zhang XO, Rozen EJ, Sun X, Sallis B, Verdejo-Torres O, et al. Prmt5 activates Akt via methylation to promote tumor metastasis. Nat Commun. (2022) 13:3955. doi: 10.1038/s41467-022-31645-1
72. Xiong Y, Ju L, Yuan L, Chen L, Wang G, Xu H, et al. Knstrn promotes tumorigenesis and gemcitabine resistance by activating Akt in bladder cancer. Oncogene. (2021) 40:1595–608. doi: 10.1038/s41388-020-01634-z
73. Xia X, Li X, Li F, Wu X, Zhang M, Zhou H, et al. A novel tumor suppressor protein encoded by circular Akt3 Rna inhibits glioblastoma tumorigenicity by competing with active phosphoinositide-dependent kinase-1. Mol Cancer. (2019) 18:131. doi: 10.1186/s12943-019-1056-5
74. Chu N, Viennet T, Bae H, Salguero A, Boeszoermenyi A, Arthanari H, et al. The structural determinants of ph domain-mediated regulation of Akt revealed by segmental labeling. Elife. (2020) 9:e59151. doi: 10.7554/eLife.59151
75. Silva-Aguiar RP, Teixeira DE, Peruchetti DB, Peres RAS, Alves SAS, Calil PT, et al. Toll like receptor 4 mediates the inhibitory effect of Sars-Cov-2 spike protein on proximal tubule albumin endocytosis. Biochim Biophys Acta Mol Basis Dis. (2024) 1870:167155. doi: 10.1016/j.bbadis.2024.167155
76. Goldbraikh D, Neufeld D, Eid-Mutlak Y, Lasry I, Gilda JE, Parnis A, et al. Usp1 deubiquitinates akt to inhibit Pi3k-Akt-Foxo signaling in muscle during prolonged starvation. EMBO Rep. (2020) 21:e48791. doi: 10.15252/embr.201948791
77. García Coronado PL, Franco Molina MA, Zárate Triviño DG, Hernández Martínez SP, Castro Valenzuela BE, Zapata Benavides P, et al. Exosomes isolated from immunepotent crp, a hemoderivative, to accelerate diabetic wound healing. Front Bioeng Biotechnol. (2024) 12:1356028. doi: 10.3389/fbioe.2024.1356028
78. Simcox J, Lamming DW. The central motor of metabolism. Dev Cell. (2022) 57:691–706. doi: 10.1016/j.devcel.2022.02.024
79. Szwed A, Kim E, Jacinto E. Regulation and metabolic functions of mtorc1 and mtorc2. Physiol Rev. (2021) 101:1371–426. doi: 10.1152/physrev.00026.2020
80. Prentzell MT, Rehbein U, Cadena Sandoval M, De Meulemeester AS, Baumeister R, Brohée L, et al. G3bps tether the tsc complex to lysosomes and suppress mtorc1 signaling. Cell. (2021) 184:655–74.e27. doi: 10.1016/j.cell.2020.12.024
81. Fitzian K, Brückner A, Brohée L, Zech R, Antoni C, Kiontke S, et al. Tsc1 binding to lysosomal pips is required for Tsc complex translocation and mtorc1 regulation. Mol Cell. (2021) 81:2705–21.e8. doi: 10.1016/j.molcel.2021.04.019
82. Liao WT, Chiang YJ, Yang-Yen HF, Hsu LC, Chang ZF, Yen JJY. Cbap regulates the function of akt-associated Tsc protein complexes to modulate Mtorc1 signaling. J Biol Chem. (2023) 299:105455. doi: 10.1016/j.jbc.2023.105455
83. Hua H, Kong Q, Zhang H, Wang J, Luo T, Jiang Y. Targeting Mtor for cancer therapy. J Hematol Oncol. (2019) 12:71. doi: 10.1186/s13045-019-0754-1
84. Hosios AM, Wilkinson ME, McNamara MC, Kalafut KC, Torrence ME, Asara JM, et al. Mtorc1 regulates a lysosome-dependent adaptive shift in intracellular lipid species. Nat Metab. (2022) 4:1792–811. doi: 10.1038/s42255-022-00706-6
85. Wang W, Tan J, Liu X, Guo W, Li M, Liu X, et al. Cytoplasmic endonuclease G promotes nonalcoholic fatty liver disease via Mtorc2-Akt-Acly and endoplasmic reticulum stress. Nat Commun. (2023) 14:6201. doi: 10.1038/s41467-023-41757-x
86. Byles V, Cormerais Y, Kalafut K, Barrera V, Hughes Hallett JE, Sui SH, et al. Hepatic mtorc1 signaling activates atf4 as part of its metabolic response to feeding and insulin. Mol Metab. (2021) 53:101309. doi: 10.1016/j.molmet.2021.101309
87. Duan S, Wang C, Xu X, Zhang X, Su G, Li Y, et al. Peripheral serum exosomes isolated from patients with acute myocardial infarction promote endothelial cell angiogenesis via the Mir-126-3p/Tsc1/Mtorc1/Hif-1α Pathway. Int J Nanomed. (2022) 17:1577–92. doi: 10.2147/ijn.S338937
88. Exposito F, Redrado M, Houry M, Hastings K, Molero-Abraham M, Lozano T, et al. Pten loss confers resistance to anti-pd-1 therapy in non-small cell lung cancer by increasing tumor infiltration of regulatory T cells. Cancer Res. (2023) 83:2513–26. doi: 10.1158/0008-5472.Can-22-3023
89. da Silva Rosa SC, Martens MD, Field JT, Nguyen L, Kereliuk SM, Hai Y, et al. Bnip3l/Nix-induced mitochondrial fission, mitophagy, and impaired myocyte glucose uptake are abrogated by Prka/Pka phosphorylation. Autophagy. (2021) 17:2257–72. doi: 10.1080/15548627.2020.1821548
90. Mangili F, Esposito E, Treppiedi D, Catalano R, Marra G, Di Muro G, et al. Drd2 agonist cabergoline abolished the escape mechanism induced by Mtor inhibitor everolimus in tumoral pituitary cells. Front Endocrinol (Lausanne). (2022) 13:867822. doi: 10.3389/fendo.2022.867822
91. Burcea IF, Năstase VN, Poiană C. Pituitary transcription factors in the immunohistochemical and molecular diagnosis of pituitary tumours - a systematic review. Endokrynol Pol. (2021) 72:53–63. doi: 10.5603/EP.a2020.0090
92. Murat CB, Braga PB, Fortes MA, Bronstein MD, Corrêa-Giannella ML, Giorgi RR. Mutation and genomic amplification of the pik3ca proto-oncogene in pituitary adenomas. Braz J Med Biol Res. (2012) 45:851–5. doi: 10.1590/s0100-879x2012007500115
93. Lin Y, Jiang X, Shen Y, Li M, Ma H, Xing M, et al. Frequent mutations and amplifications of the pik3ca gene in pituitary tumors. Endocr Relat Cancer. (2009) 16:301–10. doi: 10.1677/erc-08-0167
94. Cai Z, Qian B, Pang J, Tan ZB, Zhao K, Lei T. Celastrol induces apoptosis and autophagy via the akt/mtor signaling pathway in the pituitary Acth-secreting adenoma cells. Curr Med Sci. (2022) 42:387–96. doi: 10.1007/s11596-022-2568-6
95. Sajjad EA, Zieliński G, Maksymowicz M, Hutnik Ł, Bednarczuk T, Włodarski P. Mtor is frequently active in gh-secreting pituitary adenomas without influencing their morphopathological features. Endocr Pathol. (2013) 24:11–9. doi: 10.1007/s12022-012-9230-y
96. Lu C, Willingham MC, Furuya F, Cheng SY. Activation of phosphatidylinositol 3-kinase signaling promotes aberrant pituitary growth in a mouse model of thyroid-stimulating hormone-secreting pituitary tumors. Endocrinology. (2008) 149:3339–45. doi: 10.1210/en.2007-1696
97. Dworakowska D, Wlodek E, Leontiou CA, Igreja S, Cakir M, Teng M, et al. Activation of raf/mek/erk and pi3k/akt/mtor pathways in pituitary adenomas and their effects on downstream effectors. Endocr Relat Cancer. (2009) 16:1329–38. doi: 10.1677/erc-09-0101
98. Jia W, Sanders AJ, Jia G, Liu X, Lu R, Jiang WG. Expression of the mtor pathway regulators in human pituitary adenomas indicates the clinical course. Anticancer Res. (2013) 33:3123–31.
99. Zhang A, Xu Y, Xu H, Ren J, Meng T, Ni Y, et al. Lactate-induced M2 polarization of tumor-associated macrophages promotes the invasion of pituitary adenoma by secreting Ccl17. Theranostics. (2021) 11:3839–52. doi: 10.7150/thno.53749
100. Houghton PJ. Everolimus. Clin Cancer Res. (2010) 16:1368–72. doi: 10.1158/1078-0432.Ccr-09-1314
101. Huang H, Yan J, Xu X, Feng Y, Liu H, Liu J, et al. Everolimus inhibits hepatoblastoma by inducing autophagy-dependent ferroptosis. Drug Dev Res. (2024) 85:e22140. doi: 10.1002/ddr.22140
102. Matsuki M, Adachi Y, Ozawa Y, Kimura T, Hoshi T, Okamoto K, et al. Targeting of tumor growth and angiogenesis underlies the enhanced antitumor activity of lenvatinib in combination with everolimus. Cancer Sci. (2017) 108:763–71. doi: 10.1111/cas.13169
103. Codina-Martínez H, Lorenzo-Guerra SL, Cabal VN, García-Marín R, Suárez-Fernández L, Vivanco B, et al. Signaling pathways mtor and erk as therapeutic targets in sinonasal intestinal-type adenocarcinoma. Int J Mol Sci. (2023) 24(20):15110. doi: 10.3390/ijms242015110
104. Gorshtein A, Rubinfeld H, Kendler E, Theodoropoulou M, Cerovac V, Stalla GK, et al. Mammalian target of rapamycin inhibitors rapamycin and rad001 (Everolimus) induce anti-proliferative effects in Gh-secreting pituitary tumor cells in vitro. Endocr Relat Cancer. (2009) 16:1017–27. doi: 10.1677/erc-08-0269
105. Zatelli MC, Minoia M, Filieri C, Tagliati F, Buratto M, Ambrosio MR, et al. Effect of everolimus on cell viability in nonfunctioning pituitary adenomas. J Clin Endocrinol Metab. (2010) 95:968–76. doi: 10.1210/jc.2009-1641
106. Regazzo D, Gardiman MP, Theodoropoulou M, Scaroni C, Occhi G, Ceccato F. Silent gonadotroph pituitary neuroendocrine tumor in a patient with tuberous sclerosis complex: evaluation of a possible molecular link. Endocrinol Diabetes Metab Case Rep. (2018) 1:17–0092. doi: 10.1530/edm-18-0086
107. Rubinfeld H, Cohen O, Kammer A, Yang G, Cohen ZR, Hadani M, et al. Combination of mtor inhibitors augments potency while activating pi3k signaling in pituitary tumors. Neuroendocrinology. (2016) 103:592–604. doi: 10.1159/000442205
108. Pivonello C, Patalano R, Solari D, Auriemma RS, Frio F, Vitulli F, et al. Effect of combined treatment with a pan-pi3k inhibitor or an isoform-specific pi3k inhibitor and everolimus on cell proliferation in gh-secreting pituitary tumour in an experimental setting. Endocrine. (2018) 62:663–80. doi: 10.1007/s12020-018-1677-2
109. Sukumari-Ramesh S, Singh N, Dhandapani KM, Vender JR. Mtor inhibition reduces cellular proliferation and sensitizes pituitary adenoma cells to ionizing radiation. Surg Neurol Int. (2011) 2:22. doi: 10.4103/2152-7806.77029
110. Di Pasquale C, Gentilin E, Falletta S, Bellio M, Buratto M, Degli Uberti E, et al. Pi3k/Akt/Mtor pathway involvement in regulating growth hormone secretion in a rat pituitary adenoma cell line. Endocrine. (2018) 60:308–16. doi: 10.1007/s12020-017-1432-0
111. Jalali S, Monsalves E, Tateno T, Zadeh G. Role of mtor inhibitors in growth hormone-producing pituitary adenomas harboring different Fgfr4 genotypes. Endocrinology. (2016) 157:3577–87. doi: 10.1210/en.2016-1028
112. Jouanneau E, Wierinckx A, Ducray F, Favrel V, Borson-Chazot F, Honnorat J, et al. New targeted therapies in pituitary carcinoma resistant to temozolomide. Pituitary. (2012) 15:37–43. doi: 10.1007/s11102-011-0341-0
113. Donovan LE, Arnal AV, Wang SH, Odia Y. Widely metastatic atypical pituitary adenoma with Mtor pathway Stk11(F298l) mutation treated with everolimus therapy. CNS Oncol. (2016) 5:203–9. doi: 10.2217/cns-2016-0011
114. Yao JC, Pavel M, Phan AT, Kulke MH, Hoosen S, St Peter J, et al. Chromogranin a and neuron-specific enolase as prognostic markers in patients with advanced pnet treated with everolimus. J Clin Endocrinol Metab. (2011) 96:3741–9. doi: 10.1210/jc.2011-0666
115. Yao JC, Shah MH, Ito T, Bohas CL, Wolin EM, Van Cutsem E, et al. Everolimus for advanced pancreatic neuroendocrine tumors. N Engl J Med. (2011) 364:514–23. doi: 10.1056/NEJMoa1009290
116. Boilève A, Faron M, Fodil-Cherif S, Bayle A, Lamartina L, Planchard D, et al. Molecular profiling and target actionability for precision medicine in neuroendocrine neoplasms: real-world data. Eur J Cancer. (2023) 186:122–32. doi: 10.1016/j.ejca.2023.03.024
117. Missiaglia E, Dalai I, Barbi S, Beghelli S, Falconi M, della Peruta M, et al. Pancreatic endocrine tumors: expression profiling evidences a role for Akt-Mtor pathway. J Clin Oncol. (2010) 28:245–55. doi: 10.1200/jco.2008.21.5988
118. Zitzmann K, De Toni EN, Brand S, Göke B, Meinecke J, Spöttl G, et al. The novel mtor inhibitor rad001 (Everolimus) induces antiproliferative effects in human pancreatic neuroendocrine tumor cells. Neuroendocrinology. (2007) 85:54–60. doi: 10.1159/000100057
119. Grozinsky-Glasberg S, Franchi G, Teng M, Leontiou CA, Ribeiro de Oliveira A Jr., Dalino P, et al. Octreotide and the mtor inhibitor rad001 (Everolimus) block proliferation and interact with the akt-mtor-P70s6k pathway in a neuro-endocrine tumour cell line. Neuroendocrinology. (2008) 87:168–81. doi: 10.1159/000111501
120. Meric-Bernstam F, Gonzalez-Angulo AM. Targeting the mtor signaling network for cancer therapy. J Clin Oncol. (2009) 27:2278–87. doi: 10.1200/jco.2008.20.0766
121. O'Reilly T, McSheehy PM, Wartmann M, Lassota P, Brandt R, Lane HA. Evaluation of the mtor inhibitor, everolimus, in combination with cytotoxic antitumor agents using human tumor models in vitro and in vivo. Anticancer Drugs. (2011) 22:58–78. doi: 10.1097/CAD.0b013e3283400a20
122. Yao J, Tsuchihashi Z, Panneerselvam A, Winkler R, Bugarini R, Pavel M. Poster effect of everolimus treatment on markers of angiogenesis in patients with advanced pancreatic neuroendocrine tumours (Pnet) – results from the phase iii radiant3 study. Eur J Cancer - Eur J Cancer. (2011) 47:463. doi: 10.1016/S0959-8049(11)71884-6
123. Villaume K, Blanc M, Gouysse G, Walter T, Couderc C, Nejjari M, et al. Vegf secretion by neuroendocrine tumor cells is inhibited by octreotide and by inhibitors of the Pi3k/Akt/Mtor pathway. Neuroendocrinology. (2010) 91:268–78. doi: 10.1159/000289569
124. Avniel-Polak S, Leibowitz G, Doviner V, Gross DJ, Grozinsky-Glasberg S. Combining chloroquine with Rad001 inhibits tumor growth in a nen mouse model. Endocr Relat Cancer. (2018) 25:677–86. doi: 10.1530/erc-18-0121
125. Poore B, Yuan M, Arnold A, Price A, Alt J, Rubens JA, et al. Inhibition of mtorc1 in pediatric low-grade glioma depletes glutathione and therapeutically synergizes with carboplatin. Neuro Oncol. (2019) 21:252–63. doi: 10.1093/neuonc/noy150
126. Minocha M, Khurana V, Qin B, Pal D, Mitra AK. Co-administration strategy to enhance brain accumulation of vandetanib by modulating P-glycoprotein (P-Gp/Abcb1) and breast cancer resistance protein (Bcrp1/Abcg2) mediated efflux with M-tor inhibitors. Int J Pharm. (2012) 434:306–14. doi: 10.1016/j.ijpharm.2012.05.028
127. Subbiah V, Berry J, Roxas M, Guha-Thakurta N, Subbiah IM, Ali SM, et al. Systemic and cns activity of the ret inhibitor vandetanib combined with the mtor inhibitor everolimus in Kif5b-ret re-arranged non-small cell lung cancer with brain metastases. Lung Cancer. (2015) 89:76–9. doi: 10.1016/j.lungcan.2015.04.004
128. Carvalho DM, Richardson PJ, Olaciregui N, Stankunaite R, Lavarino C, Molinari V, et al. Repurposing vandetanib plus everolimus for the treatment of acvr1-mutant diffuse intrinsic pontine glioma. Cancer Discovery. (2022) 12:416–31. doi: 10.1158/2159-8290.Cd-20-1201
129. Cassano T, Magini A, Giovagnoli S, Polchi A, Calcagnini S, Pace L, et al. Early intrathecal infusion of everolimus restores cognitive function and mood in a murine model of Alzheimer's disease. Exp Neurol. (2019) 311:88–105. doi: 10.1016/j.expneurol.2018.09.011
130. Faraji AH, Rajendran S, Jaquins-Gerstl AS, Hayes HJ, Richardson RM. Convection-enhanced delivery and principles of extracellular transport in the brain. World Neurosurg. (2021) 151:163–71. doi: 10.1016/j.wneu.2021.05.050
131. Graham-Gurysh EG, Murthy AB, Moore KM, Hingtgen SD, Bachelder EM, Ainslie KM. Synergistic drug combinations for a precision medicine approach to interstitial glioblastoma therapy. J Control Release. (2020) 323:282–92. doi: 10.1016/j.jconrel.2020.04.028
132. Han Y, Park JH. Convection-enhanced delivery of liposomal drugs for effective treatment of glioblastoma multiforme. Drug Delivery Transl Res. (2020) 10:1876–87. doi: 10.1007/s13346-020-00773-w
133. Gau SY, Chen SL, Chang CS, Tsao TF, Tsai JD. Adverse events of everolimus in patients with tuberous sclerosis complex treated for renal angiomyolipoma/subependymal giant cell astrocytoma. Int J Med Sci. (2023) 20:1358–62. doi: 10.7150/ijms.88022
134. van Gelder T, Fischer L, Shihab F, Shipkova M. Optimizing everolimus exposure when combined with calcineurin inhibitors in solid organ transplantation. Transplant Rev (Orlando). (2017) 31:151–7. doi: 10.1016/j.trre.2017.02.007
135. Takasaki S, Kawasaki Y, Kikuchi M, Ito A, Yamaguchi H, Mano N. Clinical importance of plasma drug concentration of oral molecular targeted drugs for renal cell carcinoma. J Pharm Pharm Sci. (2021) 24:127–36. doi: 10.18433/jpps31816
136. Noguchi S, Shinohara N, Ito T, Ohtsu A, Ravaud A, Jerusalem G, et al. Relationship between pulmonary adverse events and everolimus exposure in Japanese and non-Japanese patients: A meta-analysis of oncology trials. Oncology. (2017) 92:243–54. doi: 10.1159/000457904
137. Hirabatake M, Mizuno T, Kato H, Hashida T. Everolimus pharmacokinetics and exposure-response relationship in Japanese patients with advanced breast cancer. Front Pharmacol. (2022) 13:984002. doi: 10.3389/fphar.2022.984002
138. Fukudo M, Ishibashi K, Kitada M. Real-world pharmacokinetics and pharmacodynamics of everolimus in metastatic breast cancer. Invest New Drugs. (2021) 39:1707–15. doi: 10.1007/s10637-021-01131-4
Keywords: Everolimus, pituitary tumor, PI3K/AKT/mTOR, blood-brain barrier, safety
Citation: Yao Z and Chen H (2024) Everolimus in pituitary tumor: a review of preclinical and clinical evidence. Front. Endocrinol. 15:1456922. doi: 10.3389/fendo.2024.1456922
Received: 29 June 2024; Accepted: 27 November 2024;
Published: 16 December 2024.
Edited by:
Elżbieta Skowrońska-Jóźwiak, Medical University of Łódź, PolandReviewed by:
Daniela Regazzo, University Hospital of Padua, ItalyKrzysztof Cezary Lewandowski, Medical University of Lodz, Poland
Copyright © 2024 Yao and Chen. This is an open-access article distributed under the terms of the Creative Commons Attribution License (CC BY). The use, distribution or reproduction in other forums is permitted, provided the original author(s) and the copyright owner(s) are credited and that the original publication in this journal is cited, in accordance with accepted academic practice. No use, distribution or reproduction is permitted which does not comply with these terms.
*Correspondence: Hui Chen, Y2hlbmh1aUBsenUuZWR1LmNu