- 1State Key Laboratory of Space Medicine, China Astronaut Research and Training Center, Beijing, China
- 2School of Life Science and Technology, Harbin Institute of Technology, Harbin, China
The hypometabolism induced by fasting has great potential in maintaining health and improving survival in extreme environments, among which thyroid hormone (TH) plays an important role in the adaptation and the formation of new energy metabolism homeostasis during long-term fasting. In the present review, we emphasize the potential of long-term fasting to improve physical health and emergency rescue in extreme environments, introduce the concept and pattern of fasting and its impact on the body’s energy metabolism consumption. Prolonged fasting has more application potential in emergency rescue in special environments. The changes of THs caused by fasting, including serum biochemical characteristics, responsiveness of the peripheral and central hypothalamus-pituitary-thyroid (HPT) axis, and differential changes of TH metabolism, are emphasized in particular. It was proposed that the variability between brain and liver tissues in THs uptake, deiodination activation and inactivation is the key regulatory mechanism for the cause of peripheral THs decline and central homeostasis. While hypothalamic tanycytes play a pivotal role in the fine regulation of the HPT negative feedback regulation during long-term fasting. The study progress of tanycytes on thyrotropin-releasing hormone (TRH) release and deiodination is described in detail. In conclusion, the combination of the decrease of TH metabolism in peripheral tissues and stability in the central HPT axis maintains the basal physiological requirement and new energy metabolism homeostasis to adapt to long-term food scarcity. The molecular mechanisms of this localized and differential regulation will be a key research direction for developing measures for hypometabolic applications in extreme environment.
1 Introduction
Fasting, with a history spanning nearly thousands of years, has become an ideal lifestyle choice for purportedly maintaining physical and mental well-being, improving metabolic syndrome (1) and combating cancer (2), protecting from chemotherapy toxicity (3) and promoting longevity. Medical fasting for 2 days or more has been applied for preventive and therapeutic purposes in specialized centers (4). Additionally, long-term fasting (LTF) has long been known to induce hypometabolism and hypothermia in humans and animals (5, 6), and has significant potential in the physiological maintenance during emergency rescue, particularly in extreme environments such as mine disasters, cave explorations, and manned deep space explorations. Food deprivation will be last for a long time in such conditions. Reducing energy expenditure is the best approach to enhance survival and rescue efforts. However, the practical application of fasting interventions has become increasingly challenging due to the distinct and individual consequences of short-term complete fasting for up to two days in currently prevalent forms (7, 8) and the limited evidence-based clinical data of LTF (9). More information is necessary for its broader implementations as a therapeutic prescription for diseases or valuable survival strategies for extreme environments of prolonged fasting (10).
Fasting confers healthy benefits to a greater extent than just being attributed to a reduction of caloric intake and may involve a metabolic switch, enhanced autophagy, and antioxidant capacity, as well as cellular stress resistance (11, 12). Metabolic switching from liver-derived glucose to adipose-derived ketones as a fuel source has been observed and contributes to improvements in energy metabolic regulation. Recent studies show that individuals can well tolerate a 10-day complete fasting (CF), experience an energy metabolic substrate shift, and achieve new metabolic homeostasis between days 3 to 6 of CF, with a declining resting metabolic rate (13). Additionally, fasting significantly impacts the regulation of thyroid hormone (TH) levels, which play a crucial role in the regulation of energy metabolism. However, the influence of fasting on energy metabolism and TH metabolism varies depending on the duration and frequency of fasting (14). The physiological mechanisms involved in these effects are complex and required further exploration. The principles of fasting, modifications in TH metabolism, and possible regulatory mechanisms are outlined in the present review. Here we discuss the mechanisms of potential metabolic regulatory and biological effects, and ultimately provide effective practical methods for food conservation, as well as physiological and behavioral adaptations to endure long-term food limitations.
2 Fasting definition and patterns
In humans, fasting typically refers to the dietary pattern of intentionally abstaining from food and caloric beverages for periods ranging from 12 hours to 3 weeks (15), Generally, the body enters a fasting state 8-12 hours after the last meal. Many religious groups incorporate fasting into their rituals for spiritual development and health promotion, including Daoist Bigu, Buddhist fasting, and Muslim Ramadan fasting (RF) (16). Fasting therapy is an ancient folk health method that has been established as a defined therapeutic approach in modern medicine, particularly in specialized hospitals or clinical departments of integrative medicine. Following expert consensus in Germany (17) and China (18), fasting therapy has become an evidence-based preventive treatment for modern diseases. Essentially, fasting is a physiologically adaptive process that restricts energy intake. Under different fasting modes, the body constantly makes physiological metabolic adjustments, reduces energy requirements, and systematically utilizes its own energy reserves and repairs aging components through processes such as fat breakdown, and autophagy. This leads to an improvement in physiological state and a delay in senescence, helps to prevent and treat conditions like obesity, diabetes, and cardiovascular diseases (19). Different fasting patterns produce different physiological impacts, and the health-improving effects vary accordingly.
In general, fasting can be classified into four main modalities based on fasting duration and interval. 1) Caloric Restriction (CR): This involves typically decreasing daily caloric intake by 15% to 40%. 2) Intermittent Fasting (IF): Refers to a cycle of eating patterns that can range from 12 hours to several days, with recurring periods of little to no caloric intake. Time restricted feeding (TRF) and alternate day fasting (ADF) are forms of IF. TRF limits daily food intake to a 4-8 hours window, while ADF alternates normal food intake with days of restricted food intake. The religious fasting practices of RF fall into this category. 3) Long-term Fasting or prolonged fasting (LTF): This involves completely abstaining from food for more than 2 consecutive days, typically ranging from 3 to 21 days (20, 21). Certain forms of Daoist Bigu belong to this category. 4) Periodic Fasting (PF): Lasts 2 to 7 days periodically on a weekly, monthly, or yearly scale (22). The “5:2” diet, a popular fasting regimen, involves 5 days of ad libitum feeding and two fasting days per week, during which food intake is markedly decreased to approximately 500-600 kcal. These PF/IF protocols reduce energy intake for a period of time, repeatedly mobilizing and storing energy substrates. This results in more frequent but less pronounced alterations compared to continuous fasting (15). During LTF, physiological mechanisms ensure the survival of glucose-dependent tissues and employ alternative energy source to fuel other tissues. LTF is capable of deeply remodeling energy metabolism and causes stronger effects than CR and IF (13, 23). These characteristics highlight the therapeutic potential of LTF. Moreover, extended fasting can effectively reduce resting energy expenditure (EE) and has application potential in emergency rescue under special environment with food shortage, although the inducing efficiency to hypometabolism and the health maintenance mechanism need to be further explored.
3 Influences of fasting on energy metabolic expenditure
LTF fasting reduces BMR and EE, but the effects of short-term fasting and IF have not been concluded. Fasting is a popular controlled nutritional intervention for its potential benefits in weight loss and over health improvement. Understanding its effects on EE can help tailor fasting regimens and guide to practical applications. The effects of fasting on EE vary depending on the type of fasting utilized, its duration, frequency and refeeding method (24, 25). Under normal conditions, there is an equilibrium between energy intake and expenditure. EE occurs primarily through the resting metabolic rate (RMR, accounting for approximately 60-70% of total EE), followed by physical activity (contributing about 20-30%) and the thermic effect of food (responsible for roughly 10%of total EE) (26). First of all, the effects of short-term fasting or IF on EE are still being determined. It has been observed that resting EE briefly increased during the first few days of fasting, likely due to increased sympathetic nervous system activity and catecholamine release. Other contributions to increased EE may include the energy costs of fatty acid recycling and gluconeogenesis (31, 32). The resting EE was not different after 12 hours and 60 hours of fasting, but the proportional contribution of carbohydrate, fat and protein oxidation was significantly shifted (33). Total EE showed a decrease of -1.9 MJ/day, but resting EE remained unchanged in obese individuals after 6 days of fasting for weight loss (34). Heilbronn et al. observed no significant changes in RMR and RQ from baseline to day 21 of ADF, but there was an increase in fat oxidation (35). Although RF is associated with decreased activity and sleeping time, no significant alteration was observed in RMR or total EE (36). The daily total EE and resting EE decreased about 12.4% and 6.5% respectively, after a month of RF, but there was no statistical difference in the EE of physical activity (37). Some reports indicated that the change in RMR varies at different times of RF duration. RMR was higher in the first week of RF and showed a significant downward trend in subsequent weeks, potentially due to metabolic adaptation mediated both centrally and locally (36, 38). Therefore, while IF regimens and short fasting may initially boost EE, extended fasting and continuous CR prompt a proportional metabolic slowdown. However, LTF and severe CR have been demonstrated to lower RMR and result in metabolic adaptation (13, 27). As early as 1915, Benedict reported a 20-30% decrease in energy metabolism induced by prolonged starvation (28). LTF significantly decreases 24-hour EE and the respiratory exchange ratio, as detected by a respiration chamber (29, 30). The respiratory quotient (RQ) approaches 0.7 with the extension of fasting time in a 10-day CF experiment, indicating fat as the predominant fuel source (13). An important phenomenon is that fasting leads to a significant decrease in serum thyroid hormone T3, which may contribute to the decline in RMR (39). From an evolutionary perspective, animals instinctively consume more energy to find food in the initial stage of food deprivation (13). By the way, the increased EE in the early stage of food deficiency suggest that we should strengthen psychological guidance to reduce this consumption. This metabolic adaptation likely contributes to conserve energy and enhance survival during prolonged food scarcity such as under some extreme environments.
4 Impacts of fasting on thyroid hormone metabolism
4.1 Effects of fasting on TH levels
As a crucial endocrine regulatory system for basal metabolic rate and EE, hypothalamus- pituitary-thyroid (HPT) axis is profoundly affected by various fasting protocols. To save energy and conserve protein during food shortages, one of the major adaptations is the down-regulation of TH-dependent metabolism. Firstly, the decrease in TH levels during fasting is usually temporary and reversible. Once the individual resumes normal diet habits, the TH levels return to normal. In some cases, LTF or severe CR can lead to a more significant decrease in TH and may even result in low T3 syndrome. Secondary, it is well known that fasting induces alterations of circulating THs, characterized by a marked decrease in serum triiodothyronine (T3), free T3 (FT3), an increase of reverse T3 (rT3) (40, 41), and inconsistent changes in thyroxine (T4), free T4 (FT4) and thyroid stimulating hormone (TSH), which may show a slight decrease or remain unchanged during fasting as depicted in Figure 1 by a meta-analysis during LTF. A 24-hour short-time fasting decreases FT3 by 6%, but increases rT3 by 16% in healthy humans (42). Animal experiments with 12- to 48-hour fasting also confirmed the decreases of serum T3 and T4 as summarized in a recent review (43). Fasting did not alter plasma thyrotropin-releasing hormone (TRH) levels after a 6-day fasting (44). The concentration of TRH in both the median eminence (ME) and the hypothalamic portal blood decreases during fasting (45). As a typical negative feedback regulation, the HPT axis involves the synthesis and release of TRH in the hypothalamus, which stimulates the synthesis and secretion of TSH in the anterior pituitary. Then TSH promotes the thyroid to release T4, which is later converted to active T3 by deiodinase type I (Dio1) and type II (Dio2). In turn, excessive T3 and T4 inhibit the synthesis and secretion of both TRH and TSH. The decreased serum TH concentration was not accompanied by a rise of TSH or TRH during food deprivation, reflecting a deviation from the classic negative feedback regulation of the HPT axis.
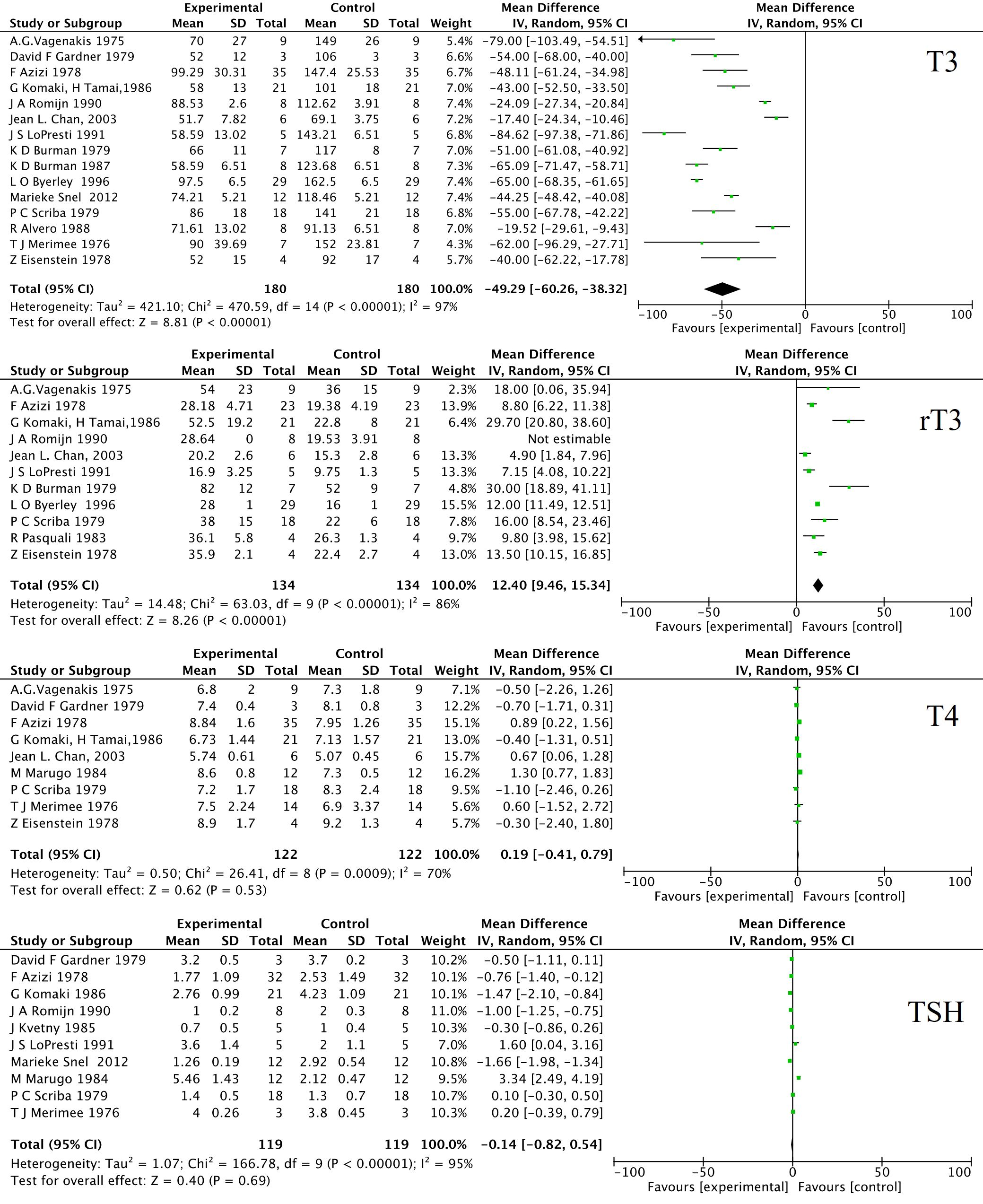
Figure 1. Forest plot of the effects of long-term fasting on T3, rT3, T4 and TSH. 16 human research papers were selected by manually review from PubMed and Web Of Science using the following term: “long-term fasting” or “prolonged fasting” and “thyroid hormone”. The data were analyzed by RevMan 5.3 for MacOS (Cochrane Collaboration, Oxford, UK) as the standard protocol and parameters. The weighted mean differences (WMD) were applied for the comparison of continuous variables. The heterogeneity in studies was assessed through the chi-squared (χ2) test and inconsistency index (I2). χ2 p value < 0.05 or I2 > 50% were considered as significant heterogeneity. A random-effect model was used to estimate the combined WMD. Otherwise, the fixed-effect model was applied. SD, standard deviation; IV, inverse variance; CI, confidence interval; df, degree of freedom.
Local TH metabolism is probably a determinant of net effects and presents a flexible and dynamic regulation, which plays an optimal role in the organism’s adaptation to a range of environmental challenges (46).The expression changes of TH metabolism-related genes differ in the liver, thyroid, pituitary and hypothalamus of mice after 48 hours of fasting (47). Differential regulations of TRH metabolism by fasting depend on the age, and species of the animals (48, 49). Thus, the combination of the decrease of TH metabolism in peripheral tissues and stability in the central HPT axis maintains the basal physiological requirement and new energy metabolism homeostasis to adapt to long-term food scarcity. The tissue variability in regulatory pattern is manifested in the differential responsiveness of the central and peripheral HPT axis to fasting, especially the intracellular T3 availability (50).
4.2 Fasting affects peripheral TH metabolism
During fasting, THs are shifted toward inactivated metabolism including the increase of rT3 conversation and decrease of T4 to T3 conversation. The decreased peripheral conversion of T4 to T3 is likely the primary mechanism responsible for circulating TH change, due to the lack of an appropriate TSH and TRH response in serum. As previously described, a prominent feature of the fasting state is a significant decrease in circulating T3 levels and an increase in rT3. Total caloric deprivation appears to shift the peripheral metabolism of T4 from the activation pathway to the deactivation pathway (51), possibly due to the deceased generation of T3 and the metabolic clearance rate of rT3 (52). The constancy of T4 levels throughout the fasting period by administration of L-thyroxine could not restore the decrease in serum T3, indicating that fasting decreases T3 production (41, 53). The enzyme activity for the conversion of T4 to T3 in fasted rat liver homogenate was reduced by 54%, primarily due to a reduction in enzyme concentration rather than co-factor availability (54). After a 48-hour fasting, only the transport of T3 into the perfused rat intracellular liver compartment decreased, but not the transport to the extracellular liver compartment (55). The clearance of rT3 is also affected by fasting. Both 7 and 13 days of fasting decreased rT3 clearance without changing rT3 production, compared to controls (56). However, Galton et al. found unchanged or even increased liver T3 and T4 concentrations within 16- or 36-hour fasting, with an increase in T3 clearance due to sulfation and UDP-glucuronidation (57). Some reports indicate that T3 glucuronidation was diminished, but T3 sulfation and subsequent deiodination were unaffected in the perfused rat liver after a 48-hour fasting (55). Similarly, Vries et al. also found that a 36-hour fasting did not alter intrahepatic T3 concentrations in rats (58), but a 48-hour fasting decreased both serum and liver T3 and T4 levels in mice (59). A gradual decrease of T3 and T4 concentrations in serum and liver during 12- to 48-hour fasting is confirmed in a recent review (43).
4.3 The potential alteration of central HPT response to fasting
Although fasting induces lower TH level, TSH and TRH secretion remains low, pointing to an altered hypothalamic setpoint of the HPT axis. Fasting, whether intermittent or prolonged, has complex effects on the response of the central HPT axis. The absence of an increase in serum TSH and TRH implies decreased negative feedback from the central part of the HPT axis. Although some reports suggest no change in serum TRH during fasting, LTF influences the secretion and function of TRH through mechanisms such as suppressing the activity of TRH neurons, reducing TRH gene expression, and inhibiting TRH secretion (45, 49). Previous animal studies have shown direct and indirect effects of decreased serum leptin contributing to the decrease of hypophysiotropic TRH neurons (40, 49). Leptin directly acts on hypophysiotropic TRH neurons that project to ME to regulate TSH production in the pituitary and regulates TRH neurons through pro-opiomelanocortin (POMC) and agouti-related peptide/neuropeptide Y (AgRP/NPY). However, recombinant methionyl human leptin only prevents the fasting-induced TSH decrease and pulsatility, but does not significantly affect the changes of the TH level (60).
Moreover, most studies report significant decreases in TSH response to TRH after fasting (60–62), but not all (63). Burman reported in 1980 that 10-day fasting impaired TSH secretion after TRH infusion (64). In the early phase (48 hours) of a 6-day fasting experiment, serum TSH still decreased in the control and TRH infused subjects (44). A 60-hour fasting decreases the mean 24-hour TSH concentration, associated with a decline in mean TSH amplitude, but not its frequency (65). Longer fasting results in a significantly lower TSH response than shorter fasting (66). Recently, Sinkó reported that fasting for 24 hours or 48 hours did not change TH action in the hypothalamic arcuate nucleus-ME region using TH action indicator mice, coupled with decreased expression of TSHβ and FT3 levels (67). Fasting promotes a 94% reduction in TSHβ mRNA expression in the pituitary (47), suggesting a decrease in the response of the pituitary to the low serum levels of T4. Fasting induces an increase of Dio2 expression in the hypothalamus, followed by an upregulation of local T3 content, which is critical to augment the suppression of the central HPT axis and avoid a negative feedback TH elevation (68). These data suggest that there are more complex and fine-tuned regulatory mechanisms of the HPT axis to maintain its stability in the brain during fasting.
5 The fine regulation of tanycytes on HPT axis during fasting
Tanycytes play a crucial role in maintaining the homeostasis of the central HPT axis during fasting by controlling the supply and metabolism of THs. Tanycytes are specialized ependymoglial cells in the third ventricle and are recognized as multifunctional players in energy metabolism through the organization of hormonal and nervous signals (69). Moreover, early studies indicated that Dio2 is absent in the PVN neurons (70). Additionally, it has been observed that a higher dose of T3 than the physiological level is necessary to reduce TRH expression in the PVN (71). This implies that the negative feedback loop involving T3 and TRH in the PVN may be less sensitive, allowing for a more nuanced regulation of the HPT axis.
5.1 Tanycytes regulate the bioavailability of THs to neurons
Acting as gatekeepers of the blood-hypothalamic barrier, tanycytes express the monocarboxylate transporter 8 (MCT8), OATP1c1 (organic anion-transporting polypeptide 1C1), and Dio2 enzyme. T4 is taken up from the cerebrospinal fluid into β2 tanycytes and deiodinated into the active form T3 by the highly expressed Dio2. Then, T3 diffuses to neighboring neurons, supplying almost 80% of the adult brain’s T3 (72–74). Interestingly, the modulator of cellular TH bioavailability µ-crystallin (CRYM), which has high affinity to T3 and T4, is relatively less expressed in β2 tanycytes than in other subtype tanycytes (75). This differential expression adjusts the time-course of T3 interaction with its receptor or the efflux of T3, and the transcription of target genes (72). TSH induces transcriptional regulation of TH-gatekeeper genes through the Tshr/Gαq/PKC pathway in tanycytes (76).
Axons from TRH-containing neurons of the paraventricular nucleus (PVN) project into the ME, where the neuron terminals make contact with the β2 tanycytic end feet (77). Increased circulating TH upregulates the expression of pyroglutamyl peptidase II (PPII), a highly specific TRH inactivating enzyme, in tanycytes and enhances the degradation of extracellular TRH in the ME through glial-axonal association (77). Stimulation of the TRH receptor I increases the intracellular calcium in tanycytes via the Gαq/11 pathway, which in turn increases the size of tanycyte end feet and the expression of PPII, moderating the release of TRH (78). Moreover, the expression of PPII in β2 tanycytes is also upregulated in response to elevated T3 levels, thereby forming a more refined negative feedback regulation. Thirdly, tanycytes are characterized by molecular signatures that sense and integrate nutrient/hormone signaling to modulate and maintain energy homeostasis (79). Indeed, β tanycytes form a “barrier” by expressing tight junction proteins and controlling the access of peripheral metabolites and hormones (80, 81). Many studies have described the metabolic sensor role of tanycytes for glucose, amino acids, leptin etc. Fasting can upregulate the facilitated glucose transporter and enhance blood-hypothalamus barrier plasticity through VEGF-dependent signaling (82, 83). Tanycytes also express receptors involved in the HPT axis and control TRH release (72, 78). Leptin has been identified as a key regulator of the central HPT axis by regulating pro-TRH expression in the PVN and could restore type III iodothyronine deiodinase (Dio3) expression in the liver (84, 85). Tanycytes regulate leptin’s entry into the hypothalamus and play an important role in the supply of THs via the expression of TH transporters and deiodinases (81).
5.2 Tanycytes mediate a central TSH-TSHR feedback loop
Tanycytes have the high number of Tshr transcripts (86). TSH released from pars tuberalis (PT) acts locally on tanycytic TSHRs, resulting in increased Dio2 expression and TH synthesis (87). Both hypothalamic tanycytes and pituitary PT-specific cells can respond to different photoperiods and regulate circulating TH levels (88). Photoperiod-dependent seasonal variations are integrated by tanycytes via the detection of the TSH released from the PT in the anterior pituitary gland (89). The PT-derived TSH is distinctly glycosylated compared with pars distalis-derived TSH. Furthermore, PT-TSH, detectable in the circulation, does not stimulate the thyroid gland (90).
Although our understanding of the functional roles of tanycytes in the regulation of the central HPT during fasting is lacking, current evidences suggest that these local regulatory mechanisms form a more refined negative feedback regulation of TH metabolism, potentially contributing to the homeostasis of the central HPT axis during fasting.
5.3 The effects of fasting on the TH metabolism in tanycytes
Local hyperthyroidism in the hypothalamus suppresses TRH release, which helps avoid excessive TRH response that could affect the body’s adaptability to fasting. Tracer kinetic studies have shown that serum T3 levels can accurately predict tissue T3 content and T3 signaling in most tissues, except the brain and pituitary gland (91). The uptake and conversion of THs are important for local T3 bioavailability and its action in the brain. Although MCT8 plays a pivotal role in the transfer of THs across the blood-brain barrier, fasting for 48 hours induces no significant alteration of MCT8 and monocarboxylate transporter 10 (MCT10) in the hypothalamus and pituitary (47, 92). MCT10 expression was found to increase upon fasting, whereas MCT8 expression remained unchanged (58). Conversely, fasting significantly induced MCT8 expression in the ependymal layer and PVN in mice (93). The impact of fasting on MCT8 expression levels and regional distribution requires further thorough investigation.
Fasting indeed impacts the expression and activity of Dio2 in tanycytes. Researchers observed a 2-fold increase in the expression of Dio2 mRNA in the mouse hypothalamus, but noted no change in the expression of Dio3 and Thrβ (47). In the initial phase of fasting, Dio2 mRNA expression and activity are robustly upregulated in tanycytes, leading to an increase in locally formed T3 and the suppression of TRH (68, 94). Coppola et al. observed that an appropriate induction of Dio2 activity during negative energy balance is dependent upon both leptin and glucocorticoid signaling (95).
Fasting influences the TRH release via tanycytes. Fasting temporarily increases the levels of PPII and Dio2 mRNA in tanycytes after 48 hours, followed by an increase of PPII activity in the ME and a partial reversion of the reduction in PVN pro-TRH mRNA levels and the number of TRH neurons. This delayed increase of ME PPII in fasted rats may facilitate the maintenance of the deep down-regulation of the HPT axis function (45).
6 Differential regulatory mechanisms
6.1 Different expression and activity of deiodinases in tissues
Despite the abundant clinical evidence, there is still a very limited understanding of how tissue-wide TH bioavailability and local TH action are regulated during development and fasting (96). Fasting decreases TH levels while keeping TSH concentrations unaltered, which is linked to local Dios expression and activity alterations in the brain hypothalamus and liver. The individual contributions of the three Dio isoenzymes to systemic and local TH provision are distinct during fasting and exhibit different regulatory mechanisms.
The main source of daily circulating T3 comes from the out ring deiodination of T4 by Dio1 and Dio2 in human. Thyroid gland contributes with about 20% of the daily T3 production, and the residual 80% is contributed outside the thyroid parenchyma, mainly from liver (97–99). The decrease in hepatic deiodination activity may be the primary factor for the decrease in circulating T3 during fasting. A 30-hour fasting decreases the activity of Dio1 in the liver and pituitary, with no changes in the kidney, and reduces the Dio2 activity in brown fat (57). However, fasting induces an increase in Dio2 expression in the hypothalamus (68). The decrease in Dio2 expression and activity was tissue-specific given that cerebral cortex Dio2 mRNA remains stable during fasting period, which leads to reduction in serum T3 levels, whereas serum T4 remains largely unaffected (98).
Dio3 is regarded as the major TH-inactivating enzyme catalyzing both T4 and T3 into rT3 and T2. Fasting results in markedly decreased serum T3 concentration coupled with a marked decrease in liver T3 concentration. Fasting also increases Dio3 mRNA expression mediated by the CAR and mTOR pathway in mice liver and WAT (59). A 36-hour fasting increases Dio3 activity, without changing Dio1 activity (14, 57). Dio3 plays a role in the fasting-induced alteration of TH homeostasis because the decrease of serum T3 is partially blunted in the Dio3-knockout mice after a 30-hour fasting (57). Leptin stimulates hypothalamus TRH expression and plays an important role in the regulation of the HPT axis. Fasting elicits significant reductions of serum leptin concentrations as shown by meta-analysis (100). Leptin administration restores the fasting-induced increase of hepatic Dio3 expression in mice, while serum TH levels and liver TRβ1 expression remain low (85). It is confirmed that fasting decreases the expression of Dio2 in the liver and increases it in the hypothalamus, and increases the expression of Dio3 in the liver. However, further exploration is needed to understand the expression and activity of Dios in the liver and hypothalamus, as well as the molecular mechanisms underlying their regulation.
6.2 Different expression of transporters in tissues
Concentrations of TH in local tissues are mediated by the uptake of circulating THs via their transmembrane transporters. The transport of T3 (55) and T4 (101) to liver cells is decreased, presumably due to the depletion of ATP in a perfused rat liver model. TH transport is mediated by widely expressed MCT8, MCT10 or brain-specific OATP1C1. Both MCT8 and MCT10 mediate T3 transport, but MCT8 also transports rT3 and T4, which are not efficiently transported by MCT10 (102). MCT10 mRNA expression is upregulated in the fasted liver, but MCT8 is not affected (14, 59). In mice, a 48-hour fasting induces a 4-fold increase of MCT8 mRNA expression in WAT, while MCT10 mRNA expression remains unchanged, indicating an enhanced uptake of T3 by adipocytes (59). Decreased MCT8 and increased MCT10 transcription in the fasted liver are also observed in rats. Moreover, the expression of both transporters is increased in the fasted gastrocnemius muscle (103). In the hypothalamus, the expression of MCT8 was reduced, but MCT10 did not change after 48-hour of fasting in mice, and its down-regulation upon fasting may be involved in this feedback mechanism (92). Till now, there is little data and research about the effects of fasting on OATP1C1, which is low expression in primates, so it is not discussed in present review.
7 Conclusion and perspective
Taken together, prolonged fasting has an application potential in emergency rescue under some special environments with food shortage, but the inducing efficiency to hypometabolism and the health maintenance mechanism still need to be understood, especially in the TH metabolism which plays an important role in energy expenditure regulation. Extended fasting significantly influences TH metabolism through localized and differentiated regulatory mechanisms in different tissues, involving the response of the HPT axis, reduced uptake and conversion of THs in the periphery, and nuanced regulation via tanycytes. The molecular mechanism of this local differentiation regulating deiodinated enzymes and transporters will be a top priority for future research.
Author contributions
XS: Conceptualization, Investigation, Writing – original draft, Writing – review & editing. SJ: Investigation, Writing – original draft, Writing – review & editing. HZ: Writing – original draft, Writing – review & editing, Funding acquisition. FW: Writing – original draft, Writing – review & editing. HW: Writing – original draft, Writing – review & editing. CY: Funding acquisition, Writing – original draft, Writing – review & editing. YG: Funding acquisition, Writing – original draft, Writing – review & editing. LW: Conceptualization, Writing – original draft, Writing – review & editing. YL: Conceptualization, Writing – original draft, Writing – review & editing. ZD: Conceptualization, Funding acquisition, Investigation, Writing – original draft, Writing – review & editing.
Funding
The author(s) declare financial support was received for the research, authorship, and/or publication of this article. This research was supported by the Advanced Space Medico-Engineering Research Project of China (2022SY54B0506, BJH22WS1J002); The State Key Laboratory of Space Medicine, China Astronaut Research and Training Center (SMFA22Q03, SMFA22B04); the Space Medical Experiment Project of China Manned Space Program (HYZHXM01002), The Shenzhen Science and Technology Innovation Commission 2020 Basic Research Project (JCYJ20200109110630285) and National Key R&D Program of China (2022YFA1604504).
Conflict of interest
The authors declare that the research was conducted in the absence of any commercial or financial relationships that could be construed as a potential conflict of interest.
Publisher’s note
All claims expressed in this article are solely those of the authors and do not necessarily represent those of their affiliated organizations, or those of the publisher, the editors and the reviewers. Any product that may be evaluated in this article, or claim that may be made by its manufacturer, is not guaranteed or endorsed by the publisher.
References
1. Michalsen A, Li C. Fasting therapy for treating and preventing disease - current state of evidence. Forsch Komplementarmedizin 2006. (2013) 20:444–53. doi: 10.1159/000357765
2. Vernieri C, Fucà G, Ligorio F, Huber V, Vingiani A, Iannelli F, et al. Fasting-mimicking diet is safe and reshapes metabolism and antitumor immunity in patients with cancer. Cancer Discovery. (2022) 12:90–107. doi: 10.1158/2159-8290.Cd-21-0030
3. Barradas M, Plaza A, Colmenarejo G, Lázaro I, Costa-MaChado LF, Martín-Hernández R, et al. Fatty acids homeostasis during fasting predicts protection from chemotherapy toxicity. Nat Commun. (2022) 13:5677. doi: 10.1038/s41467-022-33352-3
4. Wilhelmi de Toledo F, Grundler F, Sirtori CR, Ruscica M. Unravelling the health effects of fasting: A long road from obesity treatment to healthy life span increase and improved cognition. Ann Med. (2020) 52:147–61. doi: 10.1080/07853890.2020.1770849
5. Pétervári E, Balaskó M, Szelényi Z, Hummel Z, Székely M. Fasting hypometabolism and thermoregulation in cold-adapted rats. J Therm Biol. (2002) 27:359–64. doi: 10.1016/S0306-4565(02)00003-7
6. Brooke OG. Influence of malnutrition on the body temperature of children. Br Med J. (1972) 1:331–3. doi: 10.1136/bmj.1.5796.331
7. Templeman I, Smith HA, Chowdhury E, Chen Y-C, Carroll H, Johnson-Bonson D, et al. A randomized controlled trial to isolate the effects of fasting and energy restriction on weight loss and metabolic health in lean adults. Sci Transl Med. (2021) 13:eabd8034. doi: 10.1126/scitranslmed.abd8034
8. Conley M, Le Fevre L, Haywood C, Proietto J. Is two days of intermittent energy restriction per week a feasible weight loss approach in obese males? A randomised pilot study. Nutr Diet J Dietit Assoc Aust. (2018) 75:65–72. doi: 10.1111/1747-0080.12372
9. Laurens C, Grundler F, Damiot A, Chery I, Le Maho AL, Zahariev A, et al. Is muscle and protein loss relevant in long-term fasting in healthy men? a prospective trial on physiological adaptations. J Cachexia Sarcopenia Muscle. (2021) 12:1690–703. doi: 10.1002/jcsm.12766
10. Attinà A, Leggeri C, Paroni R, Pivari F, Dei Cas M, Mingione A, et al. Fasting: how to guide. Nutrients. (2021) 13:1570. doi: 10.3390/nu13051570
11. de Cabo R, Mattson MP. Effects of intermittent fasting on health, aging, and disease. N Engl J Med. (2019) 381:2541–51. doi: 10.1056/NEJMra1905136
12. Mattson MP, Longo VD, Harvie M. Impact of intermittent fasting on health and disease processes. Ageing Res Rev. (2017) 39:46–58. doi: 10.1016/j.arr.2016.10.005
13. Dai Z, Zhang H, Wu F, Chen Y, Yang C, Wang H, et al. Effects of 10-day complete fasting on physiological homeostasis, nutrition and health markers in male adults. Nutrients. (2022) 14:3860. doi: 10.3390/nu14183860
14. de Vries EM, van Beeren HC, Ackermans MT, Kalsbeek A, Fliers E, Boelen A. Differential effects of fasting vs food restriction on liver thyroid hormone metabolism in male rats. J Endocrinol. (2015) 224:25–35. doi: 10.1530/JOE-14-0533
15. Longo VD, Mattson MP. Fasting: molecular mechanisms and clinical applications. Cell Metab. (2014) 19:181–92. doi: 10.1016/j.cmet.2013.12.008
16. Kerndt PR, Naughton JL, Driscoll CE, Loxterkamp DA. Fasting: The history, pathophysiology and complications. West J Med. (1982) 137:379–99.
17. Wilhelmi de Toledo F, Buchinger A, Burggrabe H, Hölz G, Kuhn C, Lischka E, et al. Fasting therapy - an expert panel update of the 2002 consensus guidelines. Forsch Komplementmed. (2013) 20:434–43. doi: 10.1159/000357602
18. Li H, Xiao M, Li H, Ke B, Qin J, Li X, et al. Expert consensus on traditional chinese medicine fasting therapy (Draft for comments). Chin J Intergrated Tradit West Med Liver Dis. (2019) 29:4. doi: 10.3969/j.issn.1005-0264.2019.06.035
19. Golbidi S, Daiber A, Korac B, Li H, Essop MF, Laher I. Health benefits of fasting and caloric restriction. Curr Diabetes Rep. (2017) 17:123. doi: 10.1007/s11892-017-0951-7
20. Karimi R, Cleven A, Elbarbry F, Hoang H. The impact of fasting on major metabolic pathways of macronutrients and pharmacokinetics steps of drugs. Eur J Drug Metab Pharmacokinet. (2021) 46:25–39. doi: 10.1007/s13318-020-00656-y
21. Longo VD, Antebi A, Bartke A, Barzilai N, Brown-Borg HM, Caruso C, et al. Interventions to slow aging in humans: Are we ready? Aging Cell. (2015) 14:497–510. doi: 10.1111/acel.12338
22. Longo VD, Di Tano M, Mattson MP, Guidi N. Intermittent and periodic fasting, longevity and disease. Nat Aging. (2021) 1:47–59. doi: 10.1038/s43587-020-00013-3
23. Wilhelmi de Toledo F, Grundler F, Bergouignan A, Drinda S, Michalsen A. Safety, health improvement and well-being during a 4 to 21-day fasting period in an observational study including 1422 subjects. PloS One. (2019) 14(1):e0209353. doi: 10.1371/journal.pone.0209353
24. Mattson MP, Moehl K, Ghena N, Schmaedick M, Cheng A. Intermittent metabolic switching, neuroplasticity and brain health. Nat Rev Neurosci. (2018) 19:63–80. doi: 10.1038/nrn.2017.156
25. Anton SD, Moehl K, Donahoo WT, Marosi K, Lee SA, Mainous AG, et al. Flipping the metabolic switch: understanding and applying the health benefits of fasting. Obes Silver Spring Md. (2018) 26:254–68. doi: 10.1002/oby.22065
26. McMurray RG, Soares J, Caspersen CJ, McCurdy T. Examining variations of resting metabolic rate of adults: a public health perspective. Med Sci Sports Exerc. (2014) 46:1352–8. doi: 10.1249/MSS.0000000000000232
27. Redman LM, Smith SR, Burton JH, Martin CK, Il’yasova D, Ravussin E. Metabolic slowing and reduced oxidative damage with sustained caloric restriction support the rate of living and oxidative damage theories of aging. Cell Metab. (2018) 27:805–815.e4. doi: 10.1016/j.cmet.2018.02.019
28. Benedict FG, Goodall HW, Ash JE (James E, Langfeld HS, Kendall AI, Higgins HL eds. A study of prolonged fasting. Washington: Carnegie Institution of Washington (1915). p. 1.
29. Andriessen C, Doligkeit D, Moonen-Kornips E, Mensink M, Hesselink MKC, Hoeks J, et al. The impact of prolonged fasting on 24h energy metabolism and its 24h rhythmicity in healthy, lean males: a randomized cross-over trial. Clin Nutr. (2023) 42:2353–62. doi: 10.1016/j.clnu.2023.10.010
30. Weyer C, Vozarova B, Ravussin E, Tataranni PA. Changes in energy metabolism in response to 48 h of overfeeding and fasting in Caucasians and Pima Indians. Int J Obes Relat Metab Disord J Int Assoc Study Obes. (2001) 25:593–600. doi: 10.1038/sj.ijo.0801610
31. Zauner C, Schneeweiss B, Kranz A, Madl C, Ratheiser K, Kramer L, et al. Resting energy expenditure in short-term starvation is increased as a result of an increase in serum norepinephrine. Am J Clin Nutr. (2000) 71(6)1511–5. doi: 10.1093/ajcn/71.6.1511
32. Soeters MR, Soeters PB, Schooneman MG, Houten SM, Romijn JA. Adaptive reciprocity of lipid and glucose metabolism in human short-term starvation. Am J Physiol Endocrinol Metab. (2012) 303:E1397–1407. doi: 10.1152/ajpendo.00397.2012
33. Carlson MG, Snead WL, Campbell PJ. Fuel and energy metabolism in fasting humans. Am J Clin Nutr. (1994) 60:29–36. doi: 10.1093/ajcn/60.1.29
34. Siervo M, Faber P, Lara J, Gibney ER, Milne E, Ritz P, et al. Imposed rate and extent of weight loss in obese men and adaptive changes in resting and total energy expenditure. Metabolism. (2015) 64:896–904. doi: 10.1016/j.metabol.2015.03.011
35. Heilbronn LK, Smith SR, Martin CK, Anton SD, Ravussin E. Alternate-day fasting in nonobese subjects: Effects on body weight, body composition, and energy metabolism. Am J Clin Nutr. (2005) 81:69–73. doi: 10.1093/ajcn/81.1.69
36. Lessan N, Saadane I, Alkaf B, Hambly C, Buckley AJ, Finer N, et al. The effects of ramadan fasting on activity and energy expenditure. Am J Clin Nutr. (2018) 107:54–61. doi: 10.1093/ajcn/nqx016
37. Ağagündüz D, Acar-Tek N, Bozkurt O. Effect of intermittent fasting (18/6) on energy expenditure, nutritional status, and body composition in healthy adults. Evid-Based Complement Altern Med ECAM. (2021) 2021:7809611. doi: 10.1155/2021/7809611
38. Lessan N, Ali T. Energy metabolism and intermittent fasting: The ramadan perspective. Nutrients. (2019) 11:1192. doi: 10.3390/nu11051192
39. Welle SL, Campbell RG. Decrease in resting metabolic rate during rapid weight loss is reversed by low dose thyroid hormone treatment. Metabolism. (1986) 35:289–91. doi: 10.1016/0026-0495(86)90142-3
40. Boelen A, Wiersinga WM, Fliers E. Fasting-induced changes in the hypothalamus-pituitary-thyroid axis. Thyroid. (2008) 18:123–9. doi: 10.1089/thy.2007.0253
41. Merimee TJ, Fineberg ES. Starvation-induced alterations of circulating thyroid hormone concentrations in man. Metabolism. (1976) 25:79–83. doi: 10.1016/0026-0495(76)90162-1
42. Basolo A, Begaye B, Hollstein T, Vinales KL, Walter M, Santini F, et al. Effects of short-term fasting and different overfeeding diets on thyroid hormones in healthy humans. Thyroid. (2019) 29:1209–19. doi: 10.1089/thy.2019.0237
43. Seifert J, Chen Y, Schöning W, Mai K, Tacke F, Spranger J, et al. Hepatic energy metabolism under the local control of the thyroid hormone system. Int J Mol Sci. (2023) 24:4861. doi: 10.3390/ijms24054861
44. Spencer CA, Lum SM, Wilber JF, Kaptein EM, Nicoloff JT. Dynamics of serum thyrotropin and thyroid hormone changes in fasting. J Clin Endocrinol Metab. (1983) 56:883–8. doi: 10.1210/jcem-56-5-883
45. Lazcano I, Cabral A, Uribe RM, Jaimes-Hoy L, Perello M, Joseph-Bravo P, et al. Fasting enhances pyroglutamyl peptidase II activity in tanycytes of the mediobasal hypothalamus of male adult rats. Endocrinology. (2015) 156:2713–23. doi: 10.1210/en.2014-1885
46. Fliers E, Kalsbeek A, Boelen A. Beyond the fixed setpoint of the hypothalamus-pituitary-thyroid axis. Eur J Endocrinol. (2014) 171:R197–208. doi: 10.1530/EJE-14-0285
47. Fontes KN, Cabanelas A, Bloise FF, de Andrade CBV, Souza LL, Wilieman M, et al. Differential regulation of thyroid hormone metabolism target genes during non-thyroidal illness syndrome triggered by fasting or sepsis in adult mice. Front Physiol. (2017) 8:828. doi: 10.3389/fphys.2017.00828
48. De Gortari P, González-Alzati ME, Cisneros M, Joseph-Bravo P. Effect of fasting on the content of thyrotropin-releasing hormone and its mRNA in the central nervous system and pyroglutamyl peptidase II activity in the anterior pituitary of post-weaned and adult rats. Nutr Neurosci. (2000) 3:255–65. doi: 10.1080/1028415X.2000.11747323
49. Campos AMP, Wasinski F, Klein MO, Bittencourt JC, Metzger M, Donato J. Fasting reduces the number of TRH immunoreactive neurons in the hypothalamic paraventricular nucleus of male rats, but not in mice. Neurosci Lett. (2021) 752:135832. doi: 10.1016/j.neulet.2021.135832
50. Everts ME, de Jong M, Lim CF, Docter R, Krenning EP, Visser TJ, et al. Different regulation of thyroid hormone transport in liver and pituitary: Its possible role in the maintenance of low t3 production during nonthyroidal illness and fasting in man. Thyroid Off J Am Thyroid Assoc. (1996) 6:359–68. doi: 10.1089/thy.1996.6.359
51. Vagenakis AG, Burger A, Portnary GI, Rudolph M, O’Brian JR, Azizi F, et al. Diversion of peripheral thyroxine metabolism from activating to inactivating pathways during complete fasting. J Clin Endocrinol Metab. (1975) 41:191–4. doi: 10.1210/jcem-41-1-191
52. Eisenstein Z, Hagg S, Vagenakis AG, Fang SL, Ransil B, Burger A, et al. Effect of starvation on the production and peripheral metabolism of 3,3’,5’-triiodothyronine in euthyroid obese subjects. J Clin Endocrinol Metab. (1978) 47:889–93. doi: 10.1210/jcem-47-4-889
53. Naito K, Inada M, Mashio Y, Tanaka K, Ishii H, Nishikawa M, et al. Modulation of T4 5’-monodeiodination in rat anterior pituitary and liver homogenates by thyroid states and fasting. Endocrinol Jpn. (1981) 28:793–8. doi: 10.1507/endocrj1954.28.793
54. Gavin LA, Bui F, McMahon F, Cavalieri RR. Sequential deiodination of thyroxine to 3,3’-diiodothyronine via 3,5,3’-triiodothyronine and 3,3’,5’-triiodothyronine in rat liver homogenate. The effects of fasting versus glucose feeding. J Biol Chem. (1980) 255:49–54. doi: 10.1016/S0021-9258(19)86261-0
55. De Jong M, Docter R, van der Hoek HJ, Vos RA, Krenning EP, Hennemann G. Transport of 3,5,3’-triiodothyronine into the perfused rat liver and subsequent metabolism are inhibited by fasting. Endocrinology. (1992) 131:463–70. doi: 10.1210/endo.131.1.1612027
56. LoPresti JS, Gray D, Nicoloff JT. Influence of fasting and refeeding on 3,3’,5’-triiodothyronine metabolism in man. J Clin Endocrinol Metab. (1991) 72:130–6. doi: 10.1210/jcem-72-1-130
57. Galton VA, Hernandez A, St Germain DL. The 5’-deiodinases are not essential for the fasting-induced decrease in circulating thyroid hormone levels in male mice: possible roles for the type 3 deiodinase and tissue sequestration of hormone. Endocrinology. (2014) 155:3172–81. doi: 10.1210/en.2013-1884
58. de Vries EM, Eggels L, van Beeren HC, Ackermans MT, Kalsbeek A, Fliers E, et al. Fasting-induced changes in hepatic thyroid hormone metabolism in male rats are independent of autonomic nervous input to the liver. Endocrinology. (2014) 155:5033–41. doi: 10.1210/en.2014-1608
59. de Vries EM, van Beeren HC, van Wijk ACWA, Kalsbeek A, Romijn JA, Fliers E, et al. Regulation of type 3 deiodinase in rodent liver and adipose tissue during fasting. Endocr Connect. (2020) 9:552–62. doi: 10.1530/EC-20-0189
60. Chan JL, Heist K, DePaoli AM, Veldhuis JD, Mantzoros CS. The role of falling leptin levels in the neuroendocrine and metabolic adaptation to short-term starvation in healthy men. J Clin Invest. (2003) 111:1409–21. doi: 10.1172/JCI17490
61. Carlson HE, Drenick EJ, Chopra IJ, Hershman JM. Alterations in basal and TRH-stimulated serum levels of thyrotropin, prolactin, and thyroid hormones in starved obese men. J Clin Endocrinol Metab. (1977) 45:707–13. doi: 10.1210/jcem-45-4-707
62. Vinik AI, Kalk WJ, McLaren H, Hendricks S, Pimstone BL. Fasting blunts the TSH response to synthetic thyrotropin-releasing hormone (TRH). J Clin Endocrinol Metab. (1975) 40:509–11. doi: 10.1210/jcem-40-3-509
63. Gardner DF, Kaplan MM, Stanley CA, Utiger RD. Effect of tri-iodothyronine replacement on the metabolic and pituitary responses to starvation. N Engl J Med. (1979) 300:579–84. doi: 10.1056/NEJM197903153001102
64. Burman KD, Smallridge RC, Osburne R, Dimond RC, Whorton NE, Kesler P, et al. Nature of suppressed TSH secretion during undernutrition: effect of fasting and refeeding on TSH responses to prolonged TRH infusions. Metabolism. (1980) 29:46–52. doi: 10.1016/0026-0495(80)90097-9
65. Romijn JA, Adriaanse R, Brabant G, Prank K, Endert E, Wiersinga WM. Pulsatile secretion of thyrotropin during fasting: a decrease of thyrotropin pulse amplitude*. J Clin Endocrinol Metab. (1990) 70:1631–6. doi: 10.1210/jcem-70-6-1631
66. Röjdmark S. Are fasting-induced effects on thyrotropin and prolactin secretion mediated by dopamine? J Clin Endocrinol Metab. (1983) 56:1266–70. doi: 10.1210/jcem-56-6-1266
67. Sinkó R, Mohácsik P, Kővári D, Penksza V, Wittmann G, Mácsai L, et al. Different hypothalamic mechanisms control decreased circulating thyroid hormone levels in infection and fasting-induced non-thyroidal illness syndrome in male thyroid hormone action indicator mice. Thyroid Off J Am Thyroid Assoc. (2023) 33:109–18. doi: 10.1089/thy.2022.0404
68. Diano S, Naftolin F, Goglia F, Horvath TL. Fasting-induced increase in type II iodothyronine deiodinase activity and messenger ribonucleic acid levels is not reversed by thyroxine in the rat hypothalamus. Endocrinology. (1998) 139:2879–84. doi: 10.1210/endo.139.6.6062
69. Bolborea M, Langlet F. What is the physiological role of hypothalamic tanycytes in metabolism? Am J Physiol Regul Integr Comp Physiol. (2021) 320:R994–R1003. doi: 10.1152/ajpregu.00296.2020
70. Tu HM, Kim SW, Salvatore D, Bartha T, Legradi G, Larsen PR, et al. Regional distribution of type 2 thyroxine deiodinase messenger ribonucleic acid in rat hypothalamus and pituitary and its regulation by thyroid hormone. Endocrinology. (1997) 138:3359–68. doi: 10.1210/endo.138.8.5318
71. Kakucska I, Rand W, Lechan RM. Thyrotropin-releasing hormone gene expression in the hypothalamic paraventricular nucleus is dependent upon feedback regulation by both triiodothyronine and thyroxine. Endocrinology. (1992) 130:2845–50. doi: 10.1210/endo.130.5.1572297
72. Rodríguez-Rodríguez A, Lazcano I, Sánchez-Jaramillo E, Uribe RM, Jaimes-Hoy L, Joseph-Bravo P, et al. Tanycytes and the control of thyrotropin-releasing hormone flux into portal capillaries. Front Endocrinol. (2019) 10:401. doi: 10.3389/fendo.2019.00401
73. Barrett P, Ebling FJP, Schuhler S, Wilson D, Ross AW, Warner A, et al. Hypothalamic thyroid hormone catabolism acts as a gatekeeper for the seasonal control of body weight and reproduction. Endocrinology. (2007) 148:3608–17. doi: 10.1210/en.2007-0316
74. Crantz FR, Silva JE, Larsen PR. An analysis of the sources and quantity of 3,5,3’-triiodothyronine specifically bound to nuclear receptors in rat cerebral cortex and cerebellum. Endocrinology. (1982) 110:367–75. doi: 10.1210/endo-110-2-367
75. Lein ES, Hawrylycz MJ, Ao N, Ayres M, Bensinger A, Bernard A, et al. Genome-wide atlas of gene expression in the adult mouse brain. Nature. (2007) 445:168–76. doi: 10.1038/nature05453
76. Chandrasekar A, Schmidtlein PM, Neve V, Rivagorda M, Spiecker F, Gauthier K, et al. Regulation of thyroid hormone gatekeepers by thyrotropin in tanycytes. Thyroid Off J Am Thyroid Assoc. (2024) 34:261–73. doi: 10.1089/thy.2023.0375
77. Sánchez E, Vargas MA, Singru PS, Pascual I, Romero F, Fekete C, et al. Tanycyte pyroglutamyl peptidase II contributes to regulation of the hypothalamic-pituitary-thyroid axis through glial-axonal associations in the median eminence. Endocrinology. (2009) 150:2283–91. doi: 10.1210/en.2008-1643
78. Müller-Fielitz H, Stahr M, Bernau M, Richter M, Abele S, Krajka V, et al. Tanycytes control the hormonal output of the hypothalamic-pituitary-thyroid axis. Nat Commun. (2017) 8:484. doi: 10.1038/s41467-017-00604-6
79. Langlet F. Tanycyte gene expression dynamics in the regulation of energy homeostasis. Front Endocrinol. (2019) 10:286. doi: 10.3389/fendo.2019.00286
80. Mullier A, Bouret SG, Prevot V, Dehouck B. Differential distribution of tight junction proteins suggests a role for tanycytes in blood-hypothalamus barrier regulation in the adult mouse brain. J Comp Neurol. (2010) 518:943–62. doi: 10.1002/cne.22273
81. Balland E, Dam J, Langlet F, Caron E, Steculorum S, Messina A, et al. Hypothalamic tanycytes are an ERK-gated conduit for leptin into the brain. Cell Metab. (2014) 19:293–301. doi: 10.1016/j.cmet.2013.12.015
82. Langlet F, Levin BE, Luquet S, Mazzone M, Messina A, Dunn-Meynell AA, et al. Tanycytic VEGF-a boosts blood-hypothalamus barrier plasticity and access of metabolic signals to the arcuate nucleus in response to fasting. Cell Metab. (2013) 17:607–17. doi: 10.1016/j.cmet.2013.03.004
83. Barahona MJ, Langlet F, Labouèbe G, Croizier S, Picard A, Thorens B, et al. GLUT2 expression by glial fibrillary acidic protein-positive tanycytes is required for promoting feeding-response to fasting. Sci Rep. (2022) 12:17717. doi: 10.1038/s41598-022-22489-2
84. Légrádi G, Emerson CH, Ahima RS, Flier JS, Lechan RM. Leptin prevents fasting-induced suppression of prothyrotropin-releasing hormone messenger ribonucleic acid in neurons of the hypothalamic paraventricular nucleus. Endocrinology. (1997) 138:2569–76. doi: 10.1210/endo.138.6.5209
85. Boelen A, van Beeren M, Vos X, Surovtseva O, Belegri E, Saaltink D-J, et al. Leptin administration restores the fasting-induced increase of hepatic type 3 deiodinase expression in mice. Thyroid Off J Am Thyroid Assoc. (2012) 22:192–9. doi: 10.1089/thy.2011.0289
86. Ryu V, Gumerova A, Korkmaz F, Kang SS, Katsel P, Miyashita S, et al. Brain atlas for glycoprotein hormone receptors at single-transcript level. eLife. (2022) 11:e79612. doi: 10.7554/eLife.79612
87. Hanon EA, Lincoln GA, Fustin J-M, Dardente H, Masson-Pévet M, Morgan PJ, et al. Ancestral TSH mechanism signals summer in a photoperiodic mammal. Curr Biol CB. (2008) 18:1147–52. doi: 10.1016/j.cub.2008.06.076
88. Kameda Y. Regulation of circulating thyroid hormone levels by hypothalamic tanycytes and hypophysial pars tuberalis-specific cells and their morphological and gene- and protein-expression changes under different photoperiods. J Comp Neurol. (2024) 532:e25555. doi: 10.1002/cne.25555
89. Kannangara H, Cullen L, Miyashita S, Korkmaz F, Macdonald A, Gumerova A, et al. Emerging roles of brain tanycytes in regulating blood-hypothalamus barrier plasticity and energy homeostasis. Ann N Y Acad Sci. (2023) 1525:61–9. doi: 10.1111/nyas.15009
90. Ikegami K, Liao X-H, Hoshino Y, Ono H, Ota W, Ito Y, et al. Tissue-specific posttranslational modification allows functional targeting of thyrotropin. Cell Rep. (2014) 9:801–10. doi: 10.1016/j.celrep.2014.10.006
91. Salas-Lucia F, Bianco AC. T3 levels and thyroid hormone signaling. Front Endocrinol. (2022) 13:1044691. doi: 10.3389/fendo.2022.1044691
92. Schutkowski A, Wege N, Stangl GI, König B. Tissue-specific expression of monocarboxylate transporters during fasting in mice. PloS One. (2014) 9:e112118. doi: 10.1371/journal.pone.0112118
93. Bechtold DA, Sidibe A, Saer BRC, Li J, Hand LE, Ivanova EA, et al. A role for the melatonin-related receptor GPR50 in leptin signaling, adaptive thermogenesis, and torpor. Curr Biol CB. (2012) 22:70–7. doi: 10.1016/j.cub.2011.11.043
94. Coppola A, Liu Z-W, Andrews ZB, Paradis E, Roy M-C, Friedman JM, et al. A central thermogenic-like mechanism in feeding regulation: an interplay between arcuate nucleus T3 and UCP2. Cell Metab. (2007) 5:21–33. doi: 10.1016/j.cmet.2006.12.002
95. Coppola A, Meli R, Diano S. Inverse shift in circulating corticosterone and leptin levels elevates hypothalamic deiodinase type 2 in fasted rats. Endocrinology. (2005) 146:2827–33. doi: 10.1210/en.2004-1361
96. Graffunder AS, Bresser AAJ, Fernandez Vallone V, Megges M, Stachelscheid H, Kühnen P, et al. Spatiotemporal expression of thyroid hormone transporter MCT8 and THRA mRNA in human cerebral organoids recapitulating first trimester cortex development. Sci Rep. (2024) 14:9355. doi: 10.1038/s41598-024-59533-2
97. Larsen PR, Zavacki AM. Role of the iodothyronine deiodinases in the physiology and pathophysiology of thyroid hormone action. Eur Thyroid J. (2013) 1:232–42. doi: 10.1159/000343922
98. Lartey LJ, Werneck-de-Castro JP, O-Sullivan I, Unterman TG, Bianco AC. Coupling between nutrient availability and thyroid hormone activation. J Biol Chem. (2015) 290:30551–61. doi: 10.1074/jbc.M115.665505
99. Cellular and molecular basis of deiodinase-regulated thyroid hormone Signaling1 | endocrine reviews | oxford academic . Available online at: https://academic.oup.com/edrv/article/29/7/898/2355075?login=false (Accessed July 31, 2024).
100. Varkaneh Kord H, Tinsley G M, Santos H O, Zand H, Nazary A, Fatahi S, et al. The influence of fasting and energy-restricted diets on leptin and adiponectin levels in humans: a systematic review and meta-analysis. Clin Nutr Edinb Scotl. (2021) 40:1811–21. doi: 10.1016/j.clnu.2020.10.034
101. Jennings AS, Ferguson DC, Utiger RD. Regulation of the conversion of thyroxine to triiodothyronine in the perfused rat liver. J Clin Invest. (1979) 64:1614–23. doi: 10.1172/JCI109623
102. Johannes J, Braun D, Kinne A, Rathmann D, Köhrle J, Schweizer U. Few amino acid exchanges expand the substrate spectrum of monocarboxylate transporter 10. Mol Endocrinol Baltim Md. (2016) 30:796–808. doi: 10.1210/me.2016-1037
Keywords: long-term fasting, hypometabolism, thyroid hormone, extreme environment, deiodinase, tanycyte
Citation: Sui X, Jiang S, Zhang H, Wu F, Wang H, Yang C, Guo Y, Wang L, Li Y and Dai Z (2024) The influence of extended fasting on thyroid hormone: local and differentiated regulatory mechanisms. Front. Endocrinol. 15:1443051. doi: 10.3389/fendo.2024.1443051
Received: 03 June 2024; Accepted: 07 August 2024;
Published: 26 August 2024.
Edited by:
Joao DTS Anselmo, Hospital do Divino Espírito Santo, PortugalReviewed by:
Arturo Hernandez, MaineHealth Institute for Research, United StatesHelena Marcelino, University of Beira Interior, Portugal
Copyright © 2024 Sui, Jiang, Zhang, Wu, Wang, Yang, Guo, Wang, Li and Dai. This is an open-access article distributed under the terms of the Creative Commons Attribution License (CC BY). The use, distribution or reproduction in other forums is permitted, provided the original author(s) and the copyright owner(s) are credited and that the original publication in this journal is cited, in accordance with accepted academic practice. No use, distribution or reproduction is permitted which does not comply with these terms.
*Correspondence: Zhongquan Dai, ZGFpemhxNzdAMTYzLmNvbQ==; Yinghui Li, eWluZ2h1aWRkQHZpcC5zaW5hLmNvbQ==
†These authors have contributed equally to this work and share first authorship