- Heilongjiang University of Traditional Chinese Medicine, Harbin, Heilongjiang, China
Diabetic nephropathy (DN) is a microvascular complication of diabetes mellitus. The progressive damage to glomeruli, tubules, and interstitium in the kidneys can lead to the development of chronic kidney disease (CKD) and end-stage renal disease (ESRD). Most of the energy we need comes from mitochondria. Mitochondria are best known as the sites for production of respiratory ATP and are essential for eukaryotic life. The pathogenesis of DN involves a variety of factors, such as altered haemodynamics, oxidative stress, and inflammation, and studies from animal models suggest that mitochondrial dysfunction plays an important role in the development of DN. Traditional Chinese medicine (TCM) has a history of more than 2,500 years and has rich experience and remarkable efficacy in the treatment of DN. Recent studies have found that TCM may have great potential in regulating mitochondrial dysfunction in the treatment of DN. This review will elucidate the main causes of mitochondrial dysfunction and the relationship with DN, and explore in depth the potential mechanisms of TCM to protect the kidney by improving mitochondrial dysfunction. Current pharmacological treatments for patients with DN do not prevent the inevitable progression to ESRD. With the rich variety of Chinese herbs, TCM is expected to be the most promising candidate for the treatment of DN as we continue to learn more about the mechanisms of DN and incorporate the current advances in extraction techniques.
1 Introduction
Diabetic nephropathy (DN) is a chronic disease stemming from diabetes mellitus, characterized by microangiopathy and structural and functional renal alterations, standing as the foremost cause of chronic kidney disease (CKD) and end-stage renal disease (ESRD) (1). Epidemiological data reveals a global prevalence of diabetes mellitus among individuals aged 20-79 years, reaching 537 million in 2021, with projections indicating a rise to 784 million individuals (2). It is estimated that 30-40% of diabetic patients will eventually develop DN (3). Initial stages of DN manifest with glomerular hyperfiltration and hypertrophy, later progressing to impaired glomerular filtration barrier, heightened urinary albumin excretion, and accumulation of excessive extracellular matrix (ECM), leading to thylakoid stroma accumulation, hypertrophy, and consequent nodular glomerulosclerosis and interstitial fibrosis, culminating in ESRD and renal failure (4, 5). Currently, the traditional treatment of DN is mainly based on weight reduction, blood pressure and glucose control, and the use of renin-angiotensin system inhibitors (RASIs), including angiotensin-converting enzyme inhibitors (ACEIs) or angiotensin receptor blockers (ARBs) as the first-line medication for DN (6). However, there are some side effects associated with long-term use of these medications, such as ACEIs may cause angioedema and ARBs have the risk of causing hyperkalemia (7, 8). This not only substantially diminishes patients’ quality of life but also imposes significant burdens on families and society. Hence, the quest for novel therapeutic strategies or drugs to address diabetic nephropathy or mitigate disease advancement is imperative.
Mitochondria, the double-layered membrane organelles renowned as the “powerhouses” of the cell, provide the main energy for cellular activities through oxidative phosphorylation(OXPHOS) (9). The kidney, as a metabolic organ in the human body, requires a large amount of ATP to maintain its normal function, and the oxygen consumption and mitochondrial content of the kidneys are second only to the heart (10). Mitochondrial dysfunction is closely related to the development of DN, and the loss of mitochondrial control affects renal health (11). Activation of mitochondrial autophagy by maintaining mitochondria-associated endoplasmic reticulum membrane(MAM) integrity can attenuate renal tubular injury in DN during the treatment of DN (12). Under diabetic conditions, MAMs in podocytes are increased, leading to excessive mitochondrial fission and resulting in podocyte injury (13). However, excessive mitochondrial fusion may also be involved in the pathogenesis of DN (14). As revealed by the detection of mitochondrial DNA(mtDNA)in blood samples from DN patients, systemic mitochondrial dysfunction initiated by glucose induced mtDNA damage may be involved in the development of DN (15). Diabetes mellitus is known as “xiaoke” in traditional Chinese medicine and is characterised by polydipsia, polyuria, and emaciation. DN falls under the category of “xiaoke kidney disease” in TCM (16). With a history spanning thousands of years, TCM is an integral component of China’s healthcare system, focusing on the harmonization of yin and yang, and the kidneys are considered the foundation of “Yin and Yang of the five zang viscera”. According to TCM principles, the kidney governing water metabolism, emphasizing that urine production and excretion rely on the kidney qi transpiration and gasification (17). In TCM, the screening and prediction of active ingredients, disease genes, and related proteins have been conducted. Through some digital mining and analysis techniques, such as network pharmacology and molecular docking technology, the active ingredients of drugs, disease genes and related proteins are screened and predicted (18, 19). This approach aligns with the TCM concept of treating DN through “multi-components, multi-targets, and multi-pathways.” Consequently, research efforts have shifted from evaluating the clinical efficacy of single empirical prescriptions to exploring the active ingredients of TCM and the mechanisms underlying the combinations of Chinese herbal medicines, offering new therapeutic prospects for a diverse patient population.
This review discusses and analyses the relationship between mitochondrial dysfunction (e.g., mtDNA damage, mitochondrial respiratory chain damaged, mitochondrial dynamics disorders, impaired mitophagy, elevated ROS and the development of oxidative stress) and DN. It summarizes the most recent discoveries in Chinese medical therapies for DN, emphasizing their impact on regulating mitochondrial dysfunction, aiming to serve as a guide for the prevention and treatment of DN. Reviewed literature from PubMed, Web of science and China National Knowledge Infrastructure using key terminologies related to DN and Mitochondrial Dysfunction.
2 Mitochondrial dysfunction and DN
The development of DN is closely associated with damage to mtDNA, mitochondrial respiratory chain disorders, mitochondrial dynamics disorders, mitophagy damage, and elevated mitochondrial derivative ROS with the development of oxidative stress. The main mechanisms of action of mitochondrial dysfunction and DN are shown in Figure 1.
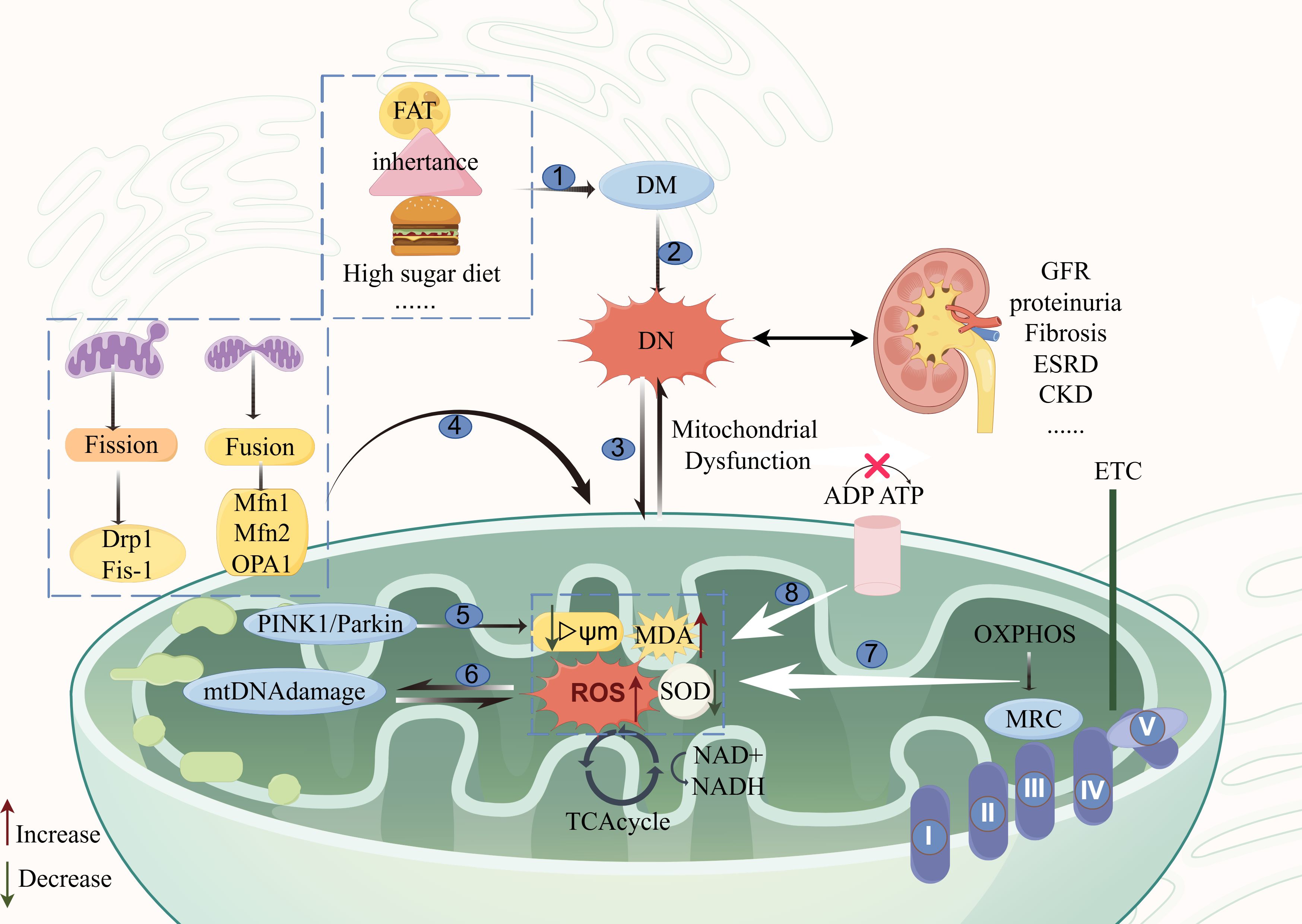
Figure 1 Diagram of the mechanism of action of mitochondrial dysfunction and DN. The main causes of mitochondrial dysfunction and the main pathways affecting the development of DN are as follows: ①: etiologies of diabetes mellitus; ②: the onset of diabetic nephropathy is rooted in diabetes mellitus; ③: mitochondrial dysfunction and diabetic nephropathy are mutually interactive; ④: mitochondrial kinetic aberrations; ⑤: mitophagy signaling pathway; ⑥: mitochondrial DNA damage pathway; ⑦: disorders of the mitochondrial respiratory chain; ⑧: impairment in ATP conversion. The figure was drawn using Figdraw (www.figdraw.com). Mfn1, Mfn2, mitochondrial fusion proteins 1 and 2; OPA1, optic atrophy protein 1; Fis1: receptor proteins mitochondrial fission1; Drp1, mitochondrial dynamin-related protein 1; MRC, The mitochondrial respiratory chain; OXPHOS, oxidative phosphorylation; DN, Diabetic nephropathy; DM, Diabetes mellitus; ROS, Reactive Oxygen Species; MDA, malondialdehyde; TCAcycle, The tricarboxylic acid cycle; GFR, Evaluation of glomerular filtration rate; ESRD, end-stage renal disease; CKD, chronic kidney disease.
2.1 Mitochondrial DNA damage and DN
Mitochondria contain their own genetic material, known as mitochondrial DNA (mtDNA), which encodes key proteins in the mitochondrial respiratory complex (20). This circular double-stranded DNA molecule is comprised of heavy and light strands, encoding a crucial array of proteins essential for the mitochondrial respiratory complex (21). The coordination between the mitochondrial genome (mtDNA) and the nuclear genome (nDNA) is essential for regulating mitochondrial function. Unlike nDNA, mtDNA is closer to sites of phosphorylated oxidation and lacks protection from the abundant histones in mitochondria, making it susceptible to damage from exogenous and endogenous stresses. mtDNA damage consists of five types: alkylation damage, hydrolysis damage, adduct formation, base mismatches, and DNA strand breaks (22). mtDNA, which is usually located in the mitochondrial matrix in the vicinity of major sites of reactive oxygen species (ROS) generation, not only induces oxidative damage, but also its mtDNA fragments escape from the matrix to the extracellular compartment and activate downstream inflammatory responses (23). Recent studies highlight the mitochondrial matrix as a principal site of mtDNA damage.
In renal cells, disruption of mitochondrial integrity may lead to the release of the mtDNA gene into the urine, potentially serving as a surrogate marker for renal mitochondrial dysfunction. Studies investigating the relationship between mtDNA copy number and the risk of kidney disease progression have revealed a negative correlation between serum mtDNA copy number levels and the progression of CKD and renal failure in individuals of both black and white ethnicities with mild to moderate CKD, as well as in patients with diabetes mellitus, and patients with albuminuria (24). In a case-control study, it was observed that 50 healthy participants, 50 patients with type 2 diabetes mellitus, and 50 patients with diabetic nephropathy had lower mtDNA copy numbers than those with diabetes mellitus and healthy controls, which further suggests that the mtDNA copy number in peripheral blood could serve as a novel potential biomarker for diabetic nephropathy patients (25). These findings collectively underscore the significance of mtDNA damage on diabetes and kidney disease, emphasizing the potential utility by detecting mtDNA in assessing and predicting diabetic nephropathy.
2.2 Mitochondrial respiratory chain damaged and DN
The mitochondrial respiratory chain (MRC) is the basic structure of OXPHOS and occupies a central position in cellular energy metabolism. Comprising two mobile electron carriers, ubiquinone and cytochrome C, as well as four multiprotein enzyme complexes (complexes I-IV) (26). These enzyme complexes facilitate the phosphorylation of adenosine diphosphate (ADP) to ATP by harnessing an electrochemical gradient across the inner membrane via an electron transport chain (27).
The kidney, being a highly metabolic organ rich in mitochondria, demands substantial ATP to sustain its physiological functions. Damage to the mitochondrial respiratory chain impedes electron transmission and reduces ATP synthesis. Consequently, impaired ATP synthesis disrupts the cation gradient, allowing Ca2+ influx, which in turn triggers the breakdown of mitochondrial membrane phospholipids. This process increases mitochondrial membrane permeability, leading to the release of the cytochrome C into the cytosol, thereby accelerating the initiation of apoptosis initiation and ultimately decreasing the mitochondrial membrane potential (MMP) (28).
Moreover, the MRC is a major site for ROS production, and in the presence of hyperglycaemia, damaged mitochondria produce excessive electron leakage in MRC complex I and at the interface between MRC complex III and coenzyme Q (29). The escaped electrons are then individually reduced, giving rise to oxygen free radicals (OFRs) that subsequently generate deleterious hydroxyl radicals, culminating in ROS accumulation; The excessive ROS production from impaired MRCs exacerbates mtDNA and respiratory chain complex enzymes, further intensifying mitochondrial damage (30). Previous studies have revealed a reduction in 31 mitochondrial proteins podocytes cultured under high glucose conditions, with key components of complexes III, IV, and V experiencing diminished levels at the respiratory chain (31). Studies have found a significant increase in the expression of respiratory chain complex subunits CO1, CO2, CO3, ATP6 and ATP8 in repair experiments of renal tubular epithelial cells by investigating lipocalin (32). Currently, some researchers also consider the MRC as a potential therapeutic target for chronic renal failure, underscoring its pivotal role in renal injury progression (33). The aforementioned studies indicate that renal mitochondria are particularly vulnerable to impairment in a high-glucose milieu, where the concurrent overproduction of ROS and diminished ATP synthesis exacerbate the progression of DN upon MRC injury.
2.3 Mitochondrial dynamics disorders and DN
The process of changing mitochondrial morphology, location and quantity within eukaryotic cells is tremed as mitochondrial dynamics, which maintains a dynamic equilibrium through constant fusion and fission (34). When there is an imbalance between these processes, it is referred to as a mitochondrial kinetic disorder. Mixing and exchange of contents between mitochondria is facilitated by mitochondrial fusion, allowing rapid metabolite exchange to replenish damaged mitochondria and maintain mitochondrial function (35). The three large kinetic protein family GTPases responsible for mitochondrial fusion are mitochondrial fusion proteins 1 and 2 (Mfn1, Mfn2), and optic atrophy protein 1 (OPA1). Mfn1 and Mfn2 are responsible for the fusion of the outer mitochondrial membrane, and the fusion of the inner mitochondrial membrane is mediated by OPA1; mitochondrial fission splits to form two new organelles, thereby providing a sufficient number of mitochondria for cell growth by providing a sufficient number of mitochondria; damaged or dysfunctional mitochondria can also be eliminated by fission to control mitochondrial mass and promote apoptosis. Mitochondrial fission is regulated by the receptor proteins mitochondrial fission1(Fis1), mitochondrial fission factor (Mff), and mitochondrial dynamin-related protein 1 (Drp1), and fission occurs at the contact site between the mitochondria and the endoplasmic reticulum at the contact site (36–38). In diabetes mellitus, insulin secretion disorders and elevated blood glucose cause an imbalance between cellular energy and demand. To maintain normal cellular energy balance, mitochondria down-regulate fusion proteins and up-regulate fission proteins, leading to an increase in the number of mitochondria, often resulting in small, fragmented, and non-functional mitochondria. As the disease progresses, this compensatory mechanism becomes insufficient, leading to secondary dysfunctions such as diabetic nephropathy (39).
It was discovered that highly fragmented and discrete mitochondria were present in high glucose-induced human podocytes, which cover the outer side of glomerular capillaries and are essential for the glomerular filtration barrier. Decreased expression of Opa1, Mfn1, and Mfn2, along with increased expression of Drp1 in podocytes cultured under high glucose conditions, mitigated excessive mitochondrial fission and cellular damage in podocytes when thromboxane/prostaglandin receptor (TP receptor) was silenced (40). Calcium/calmodulin-dependent protein kinase type 1 (CAMK1) was found to alleviate the progression of DN by regulating mitochondrial dynamics in a mouse model of DN. Conditional deletion of CAMK1 promoted mitochondrial fission and exacerbated renal injury in diabetic mice, while in vitro studies found that overexpression of CAMK1 inhibited mitochondrial fission and mitigated high-glucose-induced apoptosis in HK-2 cells (41). In another experiment (13), it was revealed that the mitochondria-associated endoplasmic reticulum membrane (MAM) promotes mitochondrial fission in podocytes under high-glucose conditions via the AKAP1-Drp1 pathway, and that excessive mitochondrial fission in podocytes is a key feature of DN. A-kinase anchoring protein 1 (AKAP1) is located in MAM, and knockdown of AKAP1 significantly reduces mitochondrial fission and attenuates high glucose-induced podocyte injury by regulating the phosphorylation of Drp1 and subsequent mitochondrial translocation. Ubiquitinated degradation of Mnf2 was found to promote ischemia-reperfusion-induced apoptosis of renal tubular epithelial cells in an animal model, suggesting that reduced mitochondrial fusion is closely associated with acute kidney injury (42). There is increasing evidence that mitochondrial fusion and fission can impact podocytes, renal tubular epithelial cells, and others in a high-glucose environment, and that an abnormal kinetic balance of mitochondria severely influences the progression of DN.
2.4 Mitophagy and DN
The process of mitophagy involves the selective encapsulation and degradation of damaged or dysfunctional mitochondria by cells through the autophagy mechanism, thereby maintaining mitochondrial and intracellular homeostasis (43). Mitophagy begins with the depolarization and lose of membrane potential in damaged mitochondria, which are then engulfed by autophagosomes to form mitochondrial autophagosomes. These structures are subsequently delivered to lysosomes for degradation, ultimately leading to the recycling of mitochondrial contents (44). Ubiquitin-mediated mitophagy, which includes PTEN-induced putative kinase 1 (PINK1)-dependent and Parkin dependent mitophagy pathways, is the major mitophagy pathway in mammals (45). PINK1 undergoes transfer to the inner mitochondrial membrane (TIM23) complex with the help of the outer mitochondrial membrane (TOM22, TOM70, TOM20). Once there, PINK1 is cleaved by the mitochondrial processing peptidase (MPP) and the presenilin-associated rhomboid-like (PARL), and then degraded via the N-end rule pathway. When mitochondria are damaged, the C-terminus in PINK1 binds to TOM7, leading to the stable accumulation of PINK1 in the mitochondrial outer membrane and trans-autophosphorylation. Parkin is an E3 ubiquitin ligase, Parkin acts as a signal amplifier which is readily phosphorylated and activated by PINK1 at Ser65. Activated Parkin ubiquitinates many mitochondrial outer membrane proteins, and these ubiquitinated proteins are further phosphorylated by PINK1, which recruits more Parkin into the mitochondria, resulting in the production of more ubiquitin chains (46, 47). Polyubiquitin chains lead to phagocytosis of target mitochondria within autophagosomes by binding to the receptor and to the microtubule-associated protein LC3 on the autophagosome membrane (48). In addition, ubiquitin-independent pathways of autophagy can initiate mitophagy without ubiquitination by binding directly to L3. For example, Nip3-like protein X (NIX)/BCL2-interacting protein 3-like (BNIP3L) receptor, BCL2-interacting protein 3 (BNIP3) receptor, FUN14 domain containing 1 (FUNDC1) receptor, and others (49). Thus, if mitophagy is impaired, there is an increased accumulation of dysfunctional mitochondria, which may lead to abnormal cellular function and contribute to the development of DN.
In a study of DN, the aldose reduction inhibitor WJ-39 demonstrated efficacy in improving renal tubular morphology and inhibiting renal fibrosis in DN rats by inhibiting the activation of the polyol pathway. Its mechanism of action appears to be linked to the activation of the PINK1/Parkin pathway, promoting mitophagy and attenuating apoptotic processes. This was corroborated by experiments on HK-2 cells exposed to high glucose conditions, confirming that WJ-39’s tubular protection is closely tied to enhanced mitophagy via PINK1/Parkin signalling pathway (50). Similarly, by detecting the effect of hypoxia-inducible factor-1 alpha (HIF-1α) on mitophagy -associated proteins in HK-2 cells cultured under high glucose conditions, revealed that HIF-1α activated the parkin/PINK1 signalling pathway. HIF-1α-Parkin/PINK1-mediated mitophagy prevented apoptosis and ROS production in HG-exposed HK-2 cells, which could protect renal tubular cells from apoptosis and ROS (51). Furthermore, in a canine model of type 1 DN treated with N-acetylcysteine (NAC) and insulin, a reduction in the gene expression levels of renal autophagy receptors BNIP3, NIX, and FUNDC1 was observed, alongside facilitation of mitochondrial fusion and inhibition of fission (52). Maintaining autophagic homeostasis is vital for normal renal function, and it has been noted that mitophagy occurs in DN in various renal cells, including podocytes, glomerular mesangial cells, and proximal tubular epithelial cells. Herbs and active compounds have shown promise in reducing renal injury by modulating mitophagy in DN (53). The above suggests that the buildup of apoptotic material is susceptible to oxidative stress and inflammatory reactions, emphasizing the crucial role of promptly eliminating damaged mitochondria and apoptotic cells to cellular homeostasis.
2.5 Increase in mitochondria-derived ROS and oxidative stress in DN
Oxidative stress (OS) is a metabolic dysfunction mediated by an imbalance between the overexpression of reactive oxygen species (ROS) and the body’s antioxidant defence system (54). The mitochondrial respiratory chain primarily generates ROS, with a minor amount produced by OXPHOS during ATP generation. ROS, as a by-product of OXPHOS, can play an important role in maintaining redox homeostasis, signal transduction, and defence against infections. The most common ROS include superoxide anion (O2-), hydrogen peroxide (H2O2), and hydroxyl radicals (OH •). OS results when ROS production exceeds the scavenging capacity of the antioxidant defence system (55). Abnormalities in oxidative stress metabolic pathways are involved in the production of diabetic free radicals and complications, including glucose oxidation and activation of glyceraldehyde-3-phosphate dehydrogenase, increased expression of advanced glycosylation end-products (AGEs) and their activating ligand receptors, diacylglycerol formation and activation of protein kinase C isoforms, overactivation of the hexosamine pathway, and increased flux of the polyol pathway (56). These aberrant pathways induce mitochondrial dysfunction and OS. In a high-glucose environment, mitochondrial OS impairs energy metabolism, activates inflammatory pathways, enhances inflammatory responses, and induces apoptosis by disrupting normal cellular metabolism (57). Experiments in DN rat model have shown significant mitochondrial OS damage in rats with DN, the mechanism of which is associated with the downregulation of PGC-1α-SIRT3-SOD2 and increased ROS activity (58). It was found that Salvianolic acid salt (SAL) reduced 8-hydroxy-2 deoxyguanosine (8-OHdG) with MDA levels by inhibiting NOX4-based NADPH oxidase in db/db mice. Its main active ingredient, SalB, blocked high glucose-induced ROS production and apoptosis in NOX4-based human podocytes thereby ameliorating oxidative stress and podocyte damage (59). All of the above studies illustrate that mitochondrial mitochondria-derived ROS constitute a primary source of oxidative stress in the kidney, emphasizing the significance of reducing ROS generation as a pivotal therapeutic strategy for DN.
3 TCM targeted mitochondrial therapy DN
TCM employs a nuanced approach to healing, harnessing the intricate pharmacological properties of natural flora. Through meticulous concoction and processing, these plants are transformed into formulas or their active constituents are extracted and isolated. In our discussion, we explore how Chinese herbal formulas, Chinese patent medicine, and compounds and extracts from Chinese herbs are utilized to regulate mitochondrial function in the treatment of DN.
3.1 Chinese herbal formulas
Chinese herbal formulas consist of two or more herbs that act synergistically and reduce toxicity. Herbal formulas have a long history of treating diabetic nephropathy, and this chapter summarises the potential link between the improvement of DN by certain herbal formulas and the modulation of mitochondrial dysfunction (Table 1).
Huangqi Danshen decoction (HDD) comprises Astragali Radix (Huang-qi) and Salviae Miltiorrhizae Radix et Rhizoma (Dan-shen). The extracts of both components can suppress Drp-1 expression and regulate the PINK1/Parkin pathway. This modulation leads to an improvement in mitophagy, which in turn alleviate renal injury in DN mice. Phenotyping experiments revealed that glomerular hypertrophy as well as the increase of thylakoid cells and thylakoid matrix were significantly improved in mice, and extensive fusion of pedunculated synapses was ameliorated by HDD treatment in mice (60).
JinChanYiShen TongLuoFormula (JCYSTL), composed of eight Chinese herbs, can activate PINK1/Parkin-mediated mitophagy by stabilising HIF-1α. Hypoxia-inducible factor-1alpha (HIF-1α) is a key molecule that plays an important role in the hypoxic environment and can be regulated by the O2 tension of the alkaline helix-loop-helix PAS heterodimer.HIF1α- is able to attenuate renal tubular cell injury by promoting mitophagy in the HG milieu (51, 66). JCYSTL can reduce the concentrations of serum creatinine (Scr),blood urea nitrogen (BUN), uric acid (UA), and 24-h urinary albumin(UAlb)in DN rats, and increase the mitochondrial respiration. concentrations and increased the activity of mitochondrial respiratory chain complexes I, III, and IV, thereby protecting renal tubules from mitochondrial dysfunction and apoptosis under DN conditions (61).
HuangQi decoction (HQD) is a traditional Chinese herbal formula consisting of Astragalus, Poria, Psoralea, Psidium guajava, Ophiopogonis, Schisandra chinensis, Glycyrrhiza glabra, and Radix Rehmanniae. Clinical studies have shown that HQD can significantly improve the TCM symptoms of qi and yin deficiency in stage III DN patients, reducing the urinary albumin-creatinine ratio (UACR), blood glucose, and blood lipid levels, while improving glycolipid metabolism disorders. Moreover, HQD effectively enhances serum inflammatory factor levels, indicating promising clinical efficacy (67, 68). In vivo experiments revealed that HQD significantly inhibited Nox4/p53/Bax signalling and effectively alleviated progressive proteinuria, glomerulosclerosis and podocyte loss in DN mice (62). NADPH oxidase is a major source of ROS, and Nox4 overexpression enhances NADPH oxidase activity, which significantly triggers oxidative stress and activates downstream signalling pathways in the podocyte, including p53, to induce podocyte injury (69). Whether HQD can have an effect on mitochondria needs to be further verified, but it also provides a new therapeutic idea for diabetic nephropathy caused by mitochondrial dysfunction.
Danggui Buxue decoction is composed of Astragali Radix and Angelicae sinensis radox in a 5:1 dosage ratio, which has the effect of tonifying Qi and generating blood, and resolving blood stasis.It has been reported that peroxisome proliferator-activated receptor-γ coactivator-1-α (PGC-1α) is a major regulator of mitochondrial biogenesis, and it participates in mitochondrial biogenesis in renal diseases by orchestrating transcriptional mechanisms. Activation of PGC-1α also prevents renal dysfunction in various renal diseases and has emerged as a potential therapeutic target against renal aging (70, 71). Studies have shown that Danggui Buxue decoction can elevate the levels of PGC-1α, MnSOD mRNA and protein expression, reduce the levels of NLRP3, IL-1β mRNA and protein expression improve the mitochondrial dysfunction of podocytes in DN rats, alleviate oxidative stress, mitigate the inflammatory response, and slow down the progression of DN (63). Clinical trials have shown that Danggui Buxue decoction can effectively reduce blood glucose, blood lipids and urinary protein levels in patients with DN, and has the effect of protecting kidney function (72).
XiaoYuXieZhuo decoction can reduce urinary protein, improve renal function, attenuate renal structural pathological changes, and inhibit podocyte apoptosis in mice with DN by modulating HIF-1α signalling pathway (64). In a controlled clinical trial, it was found that DN patients could reduce serum leptin levels and improve clinical symptoms and renal function after taking the XiaoYuXieZhuo decoction (73). HIF-1α was found to ameliorate mitochondrial dysfunction and limit mitochondria-dependent apoptosis in DN tubular cells via Heme Oxygenase 1(HO-1). It also further ameliorated renal tubular injury in DN through HO-1 mediated control of mitochondrial dynamics. Moreover, HIF-1α attenuates high-glucose-induced renal tubular cell injury by promoting PINK1/Parkin-mediated mitophagy (51, 74).
TongLuoYiShen formula consists of ten Chinese herbs, which can enhance the mRNA expression of Peroxisome proliferator-activated receptor-γ coactivator-1-α (PGC-1α), mitochondrial transcription factor A, restore the mitochondrial membrane potential, and promote mitochondrial biosynthesis in DN rats (65). PGC-1α has been validated to play a moderate role in promoting mitochondrial generation, restoring mitochondrial membrane potential, and influencing mitochondrial quality control (75). TongLuoYiShen formula combined with candesartan can delay the renal function damage of DN patients and reduce the Chinese medicine symptoms in clinical practice, and the efficacy of the combination of Chinese and Western medicine in treating DN is remarkable (76).
All aforementioned experimental findings indicate the efficacy of Chinese herbal formulas in lowering blood glucose, reducing proteinuria, and enhancing renal function. Such formulas hold promise in the treatment of DN through the modulation of mitophagy, mitochondrial respiratory chain dysfunction, oxidative stress, and other pathways.
3.2 Chinese patent medicine
Chinese patent medicine(CPM) are a component of traditional Chinese medicine (TCM), and they have the advantages of ease of use, good efficacy, low dosage, and fewer side effects compared to Western pharmaceuticals (77). Certain CPM used in the treatment of DN also have an effect on mitochondrial function (Table 2).
Gushen Jiedu capsule(GSJD)originated from the classic Chinese kidney tonic formula “Shuilu Erxiandan” in the Song Dynasty (1170 AD), which down-regulates pro-apoptotic protein Bcl-2-associated X protein (Bax) expression and up-regulates B cell lymphoma 2 (BCL-2) expression in the kidney of DN rats to regulate the mitochondrial apoptosis pathway. Bax and Bcl-2 are two key markers of apoptosis and play a critical role in high glucose-induced apoptosis in podocytes (83, 84). At the same time, the DN rat podocyte peduncle markers podocin and nephrin were also upregulated by GSJD, suggesting that GSJD may play a role in protecting the kidneys by modulating mitochondrial dysfunction (78).
QiDiTangShen granules (QDTS) significantly reduces urinary albumin excretion in db/db mice, improves thylakoid matrix deposition, attenuates protein and mRNA expression of Transforming growth factor β(TGF-β), α-smooth muscle actin (α-SMA) and collagen type I (ColI). It improves mitochondrial mass and the number of autophagic vesicles in renal tubular cells, while its active ingredients can have an effect on nutrient-sensitive signalling pathways, and can play a role in protecting the kidneys by activating mitophagy nutrient-sensing mitochondrial pathways (79).
Yishen capsule consists of five herbs, which modulate silent information regulator sirtuin 1 (SIRT1) and acetylated nuclear factor kappa-light-chain-enhancer of activated B cells(NF-kB) p65, increase the expression of SIRT1, LC3-II, and Beclin-1 in vitro and in vivo, thereby enhancing the autophagy process in podocytes through the SIRT1/NF-κB pathway, ultimately ameliorating DN (80). In addition, Yishen capsules have demonstrated efficacy in alleviating the symptoms of diabetic nephropathy by modulating the NOD-like receptor signalling pathway (85).
San-Huang-Yi-Shen capsule (SHYS) has demonstrated clinical efficacy in reducing urinary albumin levels in patients with DN, markedly enhancing renal function and mitigating renal pathological damage, with early intervention proving to be significantly more effective (86). PINK1/Parkin-mediated mitophagy is closely related to diabetic nephropathy. It has been shown that activation of PINK1/Parkin-mediated mitophagy significantly improves mitochondrial function in renal tissues, thereby attenuating renal injury in DN (87). Superoxide dismutase (SOD), catalase (CAT), and glutathione peroxidase (GPX) are antioxidant enzymes necessary for scavenging ROS from various cellular compartments and responding to stressful situations (88). SHYS capsule effectively improved renal function and reduced insulin resistance, and also up-regulated the levels of SOD, GSH-Px, reduced MDA production, and improved antioxidant capacity. It also promoted PINK1/Parkin-mediated mitophagy and inhibited the activation of NLRP3 inflammatory vesicles, thus improving mitochondrial damage and inflammatory response (81).
Danzhi Jiangtang Capsule(DJC)is a traditional Chinese medicine formula, which consists of five Chinese medicines: Radix Pseudostellariae,Radix Rehmanniae, Cortex Moutan, Rhizoma Alismatis,Semen Cuscutae Chinensis and Leech. Malondialdehyde (MDA) is one of the end products of membrane lipid peroxidation (LPO), which is considered a biomarker of oxidative stress, induced by excess ROS. The kidneys are rich in mitochondria, which are more susceptible to damage from oxidative stress (89, 90). DJC reduces LPO and MDA, increases SOD, and improves the antioxidant capacity of the kidneys. DJC treatment significantly reversed JAK2-STAT1/STAT 3 activation and ameliorated STZ-induced renal inflammatory response by inhibiting the JAK-STAT signalling pathway (82).
The results of the above studies suggest that CPM contributes to diminishing podocyte apoptosis, ameliorating thylakoid matrix deposition, and mitigating renal tubular injury. The mechanism is associated with the enhancement of mitophagy and the attenuation of oxidative stress, thereby reducing the inflammatory response.
3.3 Compounds and extracts in herbal medicine
This section encapsulates a variety of Chinese herbal compounds and extracts that exhibit promising therapeutic effects on DN, potentially through their action on mitochondria (Table 3).
3.3.1 Terpenes
Andrographis paniculata (AP) (Burm. f.) Wall. ex Nees, a renowned herb medicine in China. Andrographolide is the major active component of AP, exhibits diverse pharmacological activities, including anti-inflammatory, antioxidant, and antidiabetic activities (102, 103). Studies have demonstrated that Andrographolide not only inhibits extracellular matrix (ECM) over-accumulation, epithelial-mesenchymal transition (EMT) and attenuates diabetic tubular damage and fibrosis, but also prevents mesangial damage and fibrosis by regulating mitochondrial homeostasis and inhibiting mtROS overproduction and NLRP3 inflammasome activation in high-glycemic conditions (91). Moreover, pertinent research has demonstrated that Andrographolide possesses notable reproductive and nephrotoxic characteristics, markedly inhibiting the proliferation of HK-2 cells and inducing apoptosis (103). Ginsenoside Rg1 is the principal active ingredient in ginseng, the main component of ginseng root system, and is regarded as a high-quality component of ginseng root system (104). Rg1 can play a role in restoring mitophagy (105), reducing mitochondrial dysfunction (21), and ameliorating renal lipid deposition and renal fibrosis in type 2 DN mice (106). In vitro and in vivo studies demonstrated that Rg1 down-regulated MDA and ROS, up-regulated SOD, increased the expression of Bcl-2 protein, decreased the activity of proteins such as Bax, cleaved cystathionase-3, and cleaved cystathionase-9, and effectively inhibited the oxidative stress response and reduced apoptosis in rats with DN (92). Astragaloside II (AS II) is a novel saponin purified from the membranes of Astragalus membranaceus. ASII increased mitochondrial dynamics-related proteins such as Mfn 2, Fis1, P62 and LC3.ASII also increased the expression of PINK1 and Parkin, which are associated with mitophagy, in diabetic rats. By modulating the Nrf2 and PINK1 pathways, ASII improved podocyte damage and mitochondrial dysfunction in diabetic rats (93). The above results suggest that terpenoids can reduce the excessive buildup of the extracellular matrix in the kidney, alleviate renal fibrosis, and diminish apoptosis. Nevertheless, certain compounds may exhibit adverse effects, necessitating heightened vigilance during therapeutic application.
3.3.2 Steroidal saponins
Diosgenin (DIO) is a plant sterol saponin, since time immemorial, DIO and its derivatives, which are obtained from the rhizomes of Dioscorea (popularly known as “Shanyao” in Chinese) are utilized in traditional Chinese medications (107). Diosgenin has been shown to not only alleviate the decreased HK-2 cell viability and renal pathological damage in DN rats, but also to inhibit the expression of NOX4 and restore the expression of mitochondrial respiratory chain (MRC) complex I-V, thereby reducing the production of ROS (94). The NOX family of NADPH oxidases is an important source of reactive oxygen species, of which Nox4 is most abundantly expressed in the kidney, and is therefore not only known as the renal NADPH oxidase (Renox), but also as a new therapeutic target for vascular complications of diabetes (108, 109).
3.3.3 Flavonoid
Formononetin (FMN) is one of the major isoflavonoids isolated from Astragalus, which has been found to have antioxidant activity in some studies (110). It was verified by experiments that FMN attenuates DN renal tubular injury and mitochondrial damage, attenuates renal tubular apoptosis, mitochondrial fragmentation, and restores the expression of proteins related to mitochondrial dynamics and apoptosis-related proteins by regulating the Sirt1/PGC-1α pathway (95). Orientin is a flavonoid compound found mainly in Phyllostachys nigra, Trolus chinensis bunge and Passiflora. Orientin was found to inhibit oxidative stress and mitochondrial dysfunction (111). Orientin was found to restore mitochondrial autophagy in podocytes by in vitro experiments and has mitochondrial protective and anti-apoptotic effects (96). Bavachin is a naturally occurring flavonoid compound extracted from the seeds of the Chinese herb psoralen (112). It has been shown to be effective in anti-inflammation, inhibition of calcification and apoptosis (113, 114). Previous studies have found that Bavachin can target the electron transport chain complex to play a role in regulating mitochondrial dysfunction (115). Oral administration of Bavachin to DN mice resulted in decreased urinary microalbumin, blood urea nitrogen and creatinine clearance, and down-regulated protein levels of renal fibrosis markers α-SMA, fibronectin (FN) and collagen1α1. Meanwhile, Bavachin was further found to reduce mitochondrial ROS production and increase protein levels of antioxidant enzymes in mouse kidney tissues. Through SIRT1/PGC-1α signalling, damaged mitochondria were restored and the number of abnormal mitochondria was reduced through increased mitochondrial biosynthesis. Bavachin inhibited oxidative stress and attenuated mitochondrial dysfunction thus effectively improving DN (97). Notably, prolonged administration of low doses of Bavachin has been observed to induce renal fibrosis in zebrafish, leading to a progressive loss of the renal tubular epithelium’s normal structural specificity (116). These results suggest that exhibit significant diversity and efficacy in treating DN, potentially ameliorating mitochondrial dysfunction through various pathways, while also highlighting the necessity of considering the side effects of certain compounds during their application.
3.3.4 Lignans
Schisandrin A is one of the lignans isolated from the dried fruits of Schisandra chinensis, which has a wide range of pharmacological effects in antioxidant, inhibition of apoptosis, and modulation of immunity (117, 118). It was found that Schisandrin A suppressed the levels of urea nitrogen and urinary albumin, reduced the release of inflammatory factors, inhibited the level of MDA activity, and increased the levels of SOD, CAT, and GHS in STZ-induced DN, which effectively ameliorated oxidative stress and inflammatory responses in the DN model. Meanwhile, Schisandra chinensis A attenuated iron death and NLRP3 inflammatory vesicle-mediated pyroptosis in DN via AdipoR1/AMPK-ROS/mitochondrial damage, making it a potential drug for the treatment of DN (98). Eucommia ulmoides Oliv, also called Du-zhong, is an old tonic herb in the traditional Chinese medicine. Lignans are derived from Eucommia ulmoides Oliver (Eucommia lignans) (119). Eucommia lignans were able to attenuate the renal inflammatory response as well as improve the antioxidant capacity in DN rats by down-regulating IL-1β, TNF-α, and MDA, and up-regulating the activity of SOD; It is known that aldose reductase(AR)is a key mediator in DN as its up-regulation promotes the progression of DN and is considered a marker of DN (120). Eucommia lignans increased the viability of glomerular thylakoid cells and down-regulated AR reversal of HG-induced mitochondrial damage in glomerular thylakoid cells (99). The aforementioned studies indicate that lignans possess considerable potential in ameliorating mitochondrial dysfunction and attenuating DN oxidative stress and inflammatory responses.
3.3.5 Polyphenols
Resveratrol is a polyphenol complex that is derived from many fruits and vegetables such as peanut buds, grapes, and peanuts (121). It has great potential in the prevention and treatment of diabetes and diabetic complications (122). Phosphodiesterase-4 (PDE 4) is involved in the regulation of intracellular cyclic AMP (cAMP) levels and protein kinase A (PKA) (123). Resveratrol can ameliorate cognitive deficits in db/db mice by modulating mitochondrial function through PDE4D/PKA/Drp1 signalling (124). In recent years, it has been found that resveratrol inhibits renal mitochondrial breaks in db/db mice, restores the expression of PDE4D, PKA, phosphorylated Drp1-Ser 637, and Drp1, and attenuates the progression of DN (23).
3.3.6 Herbal extracts
The rhizome of Ligusticum chuanxiong Hort. (Abbreviated as LC) has been shown to activate blood circulation and move qi in traditional Chinese medicine. Ethanol extract of Ligusticum chuanxiong rhizome (EEL) reduces oxidative stress and inflammatory response in mice; Nrf2 is considered a novel therapeutic target for DN because of its ability to eliminate ROS and regulate redox balance (125). Validated to exert antioxidant and anti-inflammatory effects in DN by in vitro experiments, EEL was able to inhibit oxidative stress and inflammation through the Nrf2 and NF-κB signalling pathways, exerting a renoprotective effect (100). P38MAPK is an important member of the mitogen-activated protein kinase (MAPK), which plays a pathological role in diabetes and leads to apoptosis in podocytes (126). Cassia alata ethanolic leaf extract (CALE) reduced autophagic necrotic apoptosis in diabetic nephropathy by inhibiting the expression of proteins such as LC 3-IL,IL-11,RIP-IL 1 ,p38 MAPK through in vitro and in vivo experiments, which opens up new avenues of research to study the efficacy of CALE in diabetes-related complications (101). The above studies have shown that herbal extracts can reduce renal inflammation and oxidative stress, thereby contributing to nephroprotection.
In summary, Chinese medicine (Chinese herbal formulas, Chinese patent medicine, compounds and extracts from Chinese herbs) may play a role in the treatment of DN by inhibiting the production of ROS, alleviating oxidative stress and inflammatory responses, restoring mitochondrial function, and decreasing apoptosis, thereby reducing proteinuria, improving renal function, and decreasing renal pathological damage. The main mechanism of action may be related to the pathways of PINK1/Parkin, AKAP1/Drp1, Nox4/p53/Bax, Nrf2/HO-1, SIRT1/NF-κB, JAK-STAT, Sirt1/PGC-1α, and AdipoR1/AMPK. The mechanism of action of TCM in regulating mitochondrial function to improve DN is shown in Figure 2.
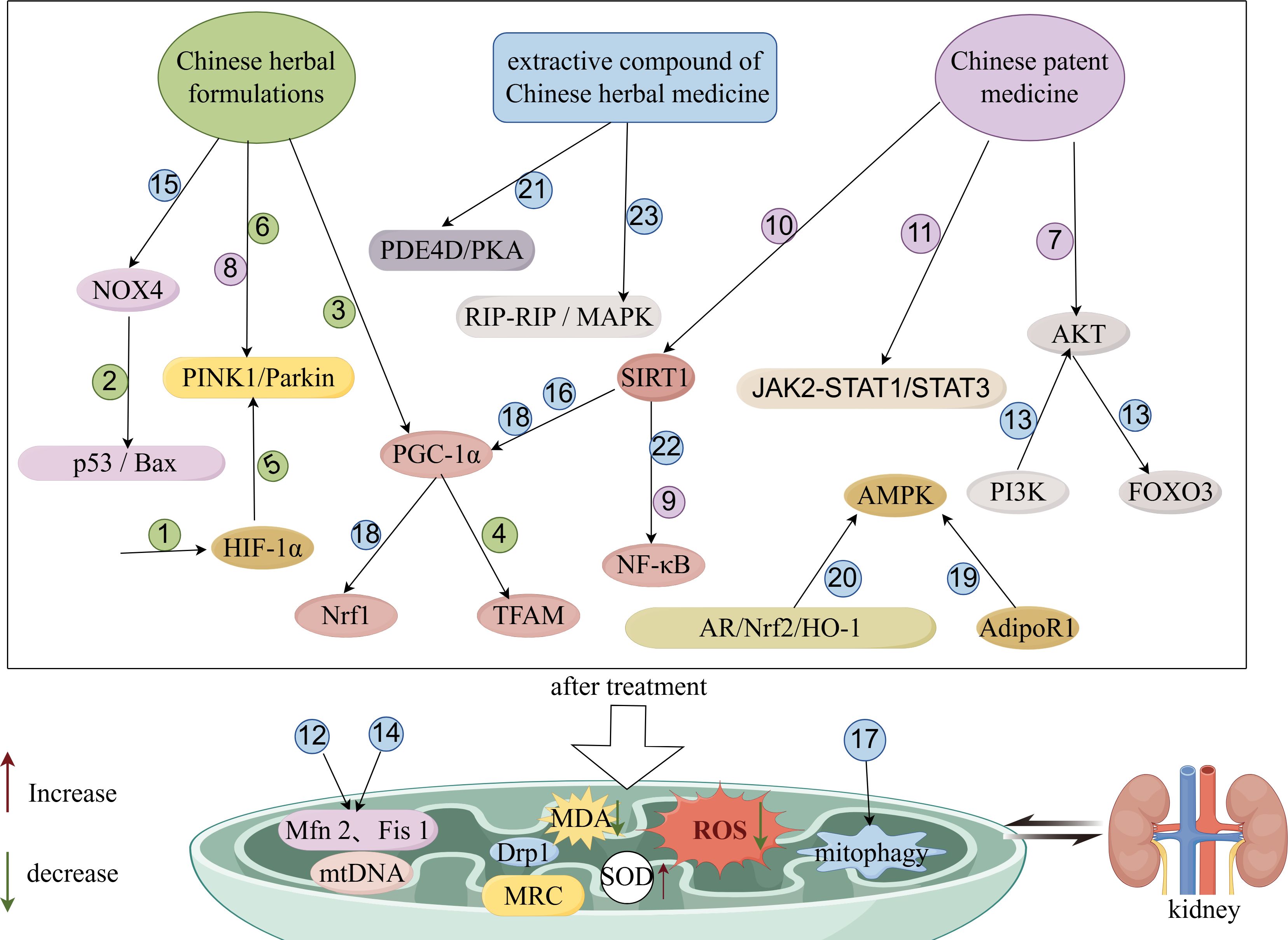
Figure 2 Schematic diagram of improvement of DN by TCM through modulation of mitochondrial function. The figure was drawn using Figdraw (www.figdraw.com). 1: XiaoYuXieZhuo decoction; 2: HuangQi decoction; 3: Danggui Buxue decoction; 4: TongLuoYiShen formula; 5: JinChanYiShen TongLuo Formula; 6: Huangqi Danshen decoction; 7: Gushen Jiedu capsule; 8: San-Huang-Yi-Shen capsule; 9: Yishen capsule; 10: QiDiTangShen granules; 11: DanzhiJiangtangCapsule; 12: Andrographolide; 13: GinsenosideRg1; 14: Astragaloside II; 15: Diosgenin; 16: Formononetin; 17: Orientin; 18: Bavachin; 19: SchisandrinA; 20: Eucommia lignans; 21: Resveratrol; 22: Ethanol extract of Ligusticum chuanxiong rhizome; 23: Cassia alata ethanolic leaf extract.
4 Conclusion
With the development of society, the prevalence of diabetic nephropathy is increasing globally, which not only greatly aggravates the economic burden on society and families, but also becomes a very serious public health problem. Therefore, it is important to find new and more effective treatments with fewer side effects. Chinese medicine is a medical theory system with rich clinical practice established based on the human body and the laws of nature in the development of thousands of years (127). Chinese medicine often emphasizes disease prevention, encouraging the establishment of healthy lifestyle habits encompassing diet, mood and exercise to improve the body’s immunity and prevent diabetic diseases. Similarly, when managing diseases, such as diabetes, there is an emphasis on preventing their progression. For individuals already living with diabetes, early diagnosis and treatment are crucial to prevent the development of DN, safeguard renal function, and decelerate the progression of diabetes. This approach bears resemblance to modern medical strategies for managing diabetic nephropathy (128).
Mitochondria contain genetic material that produces energy and is involved in various metabolic activities within the cell. Oxidative stress, apoptosis, and inflammatory responses caused by mitochondrial dysfunction (e.g., mtDNA damage, damage to the mitochondrial respiratory chain, altered mitochondrial dynamics, impaired mitochondrial autophagy, and elevated ROS with the onset of oxidative stress) are broadly involved in the development of DN. TCM can play a therapeutic role in DN by regulating the PINK1/Parkin-guided mitophagy pathway, and it can also influence mitochondrial fusion and fission through the regulation of kinetic proteins, such as Mfn1, Mfn2, and Drp1. At the same time, many studies have demonstrated that TCM also reduces the production of ROS, improves the antioxidant capacity of the kidneys, and inhibits oxidative stress in the kidneys.
5 Limitations and future prospects
TCM possesses both advantages and limitations in addressing mitochondrial dysfunction in the management of diabetic nephropathy. In terms of safety, compared to certain pharmacological treatments, TCM typically offers enhanced safety and tolerability, rendering it suitable for long-term disease management. Regarding therapeutic stability, TCM often strives to regulate overall balance, thereby modulating the immune system and enhancing the body’s general condition, which contributes to the stability and durability of therapeutic outcomes. As for individualized treatment, TCM emphasizes syndrome differentiation and personalized approaches, enabling the formulation of tailored treatment plans based on the unique physical and medical characteristics of each patient, thereby enhancing the specificity and efficacy of the treatment.
The pathogenesis of DN is multifaceted, often implicating a confluence of factors. Autophagy and pyroptosis are notable contributors to DN, while oxidative stress and inflammatory responses in the kidney have persistently been focal points of research. Emerging studies underscore the roles of ferroptosis (129), gut microbiota (130), myokines (131), immune response (132), and other pathways in DN pathogenesis. These mechanisms may synergistically interact within the renal environment; For instance, DN induced by mitochondrial dysfunction may coincide with the onset of ferroptosis. Regrettably, current research predominantly targets isolated mechanisms, leaving the interplay of multiple pathways in DN insufficiently explored. Additionally, TCM boasts the advantage of a multi-target, multi-pathway, and multi-approach strategy for treating diseases, yet limitations such as low oral bioavailability and unclear target specificity impede drug development. Future advancements must focus on improving technical methodologies, including the extraction and identification technologies of TCM. Compounds and extracts from herbal medicine have demonstrated efficacy in animal models of DN, but the paucity of clinical trials and issues with oral bioavailability constrain their development. Moreover, the complexity of herbal compound identification and the potential side effects of certain TCM components necessitate further experimental validation. In terms of therapeutic strategies, guidance on the combined use of Chinese medicine and modern pharmacotherapy is lacking, necessitating the establishment of standardized protocols for DN treatment. Integrating TCM with nanomedicines to precisely target the kidney and address the issue of low oral bioavailability presents a promising future treatment modality (133). The amalgamation of traditional Chinese and Western medicines may represent the optimal therapeutic approach for DN.
This review encapsulates some of the most promising Chinese medicinal agents for regulating mitochondrial dysfunction in DN treatment. It is anticipated that future advancements in artificial intelligence, coupled with refined identification and extraction technologies for TCM components, will herald high-quality clinical trials focused on mitochondrial dysfunction, thereby elevating the clinical management of DN.
Author contributions
D-MZ: Writing – original draft, Data curation, Investigation. RZ: Data curation, Investigation, Writing – review & editing. X-TW: Funding acquisition, Writing – review & editing. Z-HY: Writing – review & editing, Funding acquisition.
Funding
The author(s) declare financial support was received for the research, authorship, and/or publication of this article. This work was financially supported by Medical and Health Research of Heilongjiang Province of China (No. 20230202080141).
Conflict of interest
The authors declare that the research was conducted in the absence of any commercial or financial relationships that could be construed as a potential conflict of interest.
Publisher’s note
All claims expressed in this article are solely those of the authors and do not necessarily represent those of their affiliated organizations, or those of the publisher, the editors and the reviewers. Any product that may be evaluated in this article, or claim that may be made by its manufacturer, is not guaranteed or endorsed by the publisher.
References
1. Lin YC, Chang YH, Yang SY, Wu KD, Chu TS. Update of pathophysiology and management of diabetic kidney disease. J Formos Med Assoc. (2018) 117:662–75. doi: 10.1016/j.jfma.2018.02.007
2. Sun H, Saeedi P, Karuranga S, Pinkepank M, Ogurtsova K, Duncan BB, et al. IDF Diabetes Atlas: Global, regional and country-level diabetes prevalence estimates for 2021 and projections for 2045. Diabetes Res Clin Pract. (2022) 183:109119. doi: 10.1016/j.diabres.2021.109119
3. Bonner R, Albajrami O, Hudspeth J, Upadhyay A. Diabetic kidney disease. Prim Care. (2020) 47:645–59. doi: 10.1016/j.pop.2020.08.004
4. Zhao L, Zou Y, Liu F. Transforming growth factor-beta1 in diabetic kidney disease. Front Cell Dev Biol. (2020) 8:187. doi: 10.3389/fcell.2020.00187
5. Tonneijck L, Muskiet MH, Smits MM, van Bommel EJ, Heerspink HJ, van Raalte DH, et al. Glomerular hyperfiltration in diabetes: mechanisms, clinical significance, and treatment. J Am Soc Nephrol. (2017) 28:1023–39. doi: 10.1681/ASN.2016060666
6. Doshi SM, Friedman AN. Diagnosis and management of type 2 diabetic kidney disease. Clin J Am Soc Nephrol. (2017) 12:1366–73. doi: 10.2215/CJN.11111016
7. Scott SA, Nicoletti P. Novel pharmacogenomic locus implicated in angiotensin-converting enzyme inhibitor-induced angioedema. J Am Coll Cardiol. (2021) 78:710–2. doi: 10.1016/j.jacc.2021.05.050
8. Huang Y, Zhang Y, Ma L, Zhou H, Fang C, Chen C. Adverse events of sacubitril/valsartan: A meta-analysis of randomized controlled trials. J Cardiovasc Pharmacol. (2021) 78:202–10. doi: 10.1097/FJC.0000000000001049
9. Kummer E, Ban N. Mechanisms and regulation of protein synthesis in mitochondria. Nat Rev Mol Cell Biol. (2021) 22:307–25. doi: 10.1038/s41580-021-00332-2
10. Pagliarini DJ, Calvo SE, Chang B, Sheth SA, Vafai SB, Ong SE, et al. A mitochondrial protein compendium elucidates complex I disease biology. Cell. (2008) 134:112–23. doi: 10.1016/j.cell.2008.06.016
11. Srivastava SP, Kanasaki K, Goodwin JE. Loss of mitochondrial control impacts renal health. Front Pharmacol. (2020) 11:543973. doi: 10.3389/fphar.2020.543973
12. Yang M, Zhang Q, Luo S, Han Y, Zhao H, Jiang N, et al. DsbA-L alleviates tubular injury in diabetic nephropathy by activating mitophagy through maintenance of MAM integrity. Clin Sci (Lond). (2023) 137:931–45. doi: 10.1042/CS20220787
13. Li X, Yang Q, Liu S, Song S, Wang C. Mitochondria-associated endoplasmic reticulum membranes promote mitochondrial fission through AKAP1-Drp1 pathway in podocytes under high glucose conditions. Exp Cell Res. (2023) 424:113512. doi: 10.1016/j.yexcr.2023.113512
14. Kim K, Cha SJ, Choi HJ, Kang JS, Lee EY. Dysfunction of mitochondrial dynamics in drosophila model of diabetic nephropathy. Life (Basel). (2021) 11. doi: 10.3390/life11010067
15. Czajka A, Ajaz S, Gnudi L, Parsade CK, Jones P, Reid F, et al. Altered mitochondrial function, mitochondrial DNA and reduced metabolic flexibility in patients with diabetic nephropathy. EBioMedicine. (2015) 2:499–512. doi: 10.1016/j.ebiom.2015.04.002
16. Zenghui MF. Research progress on etiology and pathogenesis of diabetic nephropathy in chinese medicine. J Basic Chin Med. (2022) 28(08):1373–7. doi: 10.19945/j.cnki.issn.1006-3250.2022.08.036
17. Yinrui W. Probing into the treatment of proteinuria in diabetes nephropathy based on the principle of “scattering and receiving. J Traditional Chin Med. (2023) 40(10):1288–91. doi: 10.11656/j.issn.1672-1519.2023.10.12
18. Fu S, Zhou Y, Hu C, Xu Z, Hou J. Network pharmacology and molecular docking technology-based predictive study of the active ingredients and potential targets of rhubarb for the treatment of diabetic nephropathy. BMC Complement Med Ther. (2022) 22:210. doi: 10.1186/s12906-022-03662-6
19. Dong Y, Zhao Q, Wang Y. Network pharmacology-based investigation of potential targets of astragalus membranaceous-angelica sinensis compound acting on diabetic nephropathy. Sci Rep. (2021) 11:19496. doi: 10.1038/s41598-021-98925-6
20. Luo S, Yang M, Zhao H, Han Y, Liu Y, Xiong X, et al. Mitochondrial DNA-dependent inflammation in kidney diseases. Int Immunopharmacol. (2022) 107:108637. doi: 10.1016/j.intimp.2022.108637
21. Li J, Gao W, Zhao Z, Li Y, Yang L, Wei W, et al. Ginsenoside rg1 reduced microglial activation and mitochondrial dysfunction to alleviate depression-like behaviour via the GAS5/EZH2/SOCS3/NRF2 axis. Mol Neurobiol. (2022) 59:2855–73. doi: 10.1007/s12035-022-02740-7
22. Carvalho G, Repolês BM, Mendes I, Wanrooij PH. Mitochondrial DNA instability in mammalian cells. Antioxid Redox Signal. (2022) 36:885–905. doi: 10.1089/ars.2021.0091
23. Zhu X, Deng Z, Cao Y, Zhou Z, Sun W, Liu C, et al. Resveratrol prevents Drp1-mediated mitochondrial fission in the diabetic kidney through the PDE4D/PKA pathway. Phytother Res. (2023) 37:5916–31. doi: 10.1002/ptr.8004
24. He WJ, Li C, Huang Z, Geng S, Rao VS, Kelly TN, et al. Association of mitochondrial DNA copy number with risk of progression of kidney disease. Clin J Am Soc Nephrol. (2022) 17:966–75. doi: 10.2215/CJN.15551121
25. Al-Kafaji G, Aljadaan A, Kamal A, Bakhiet M. Peripheral blood mitochondrial DNA copy number as a novel potential biomarker for diabetic nephropathy in type 2 diabetes patients. Exp Ther Med. (2018) 16:1483–92. doi: 10.3892/etm.2018.6319
26. Vercellino I, Sazanov LA. The assembly, regulation and function of the mitochondrial respiratory chain. Nat Rev Mol Cell Biol. (2022) 23:141–61. doi: 10.1038/s41580-021-00415-0
27. Guan S, Zhao L, Peng R. Mitochondrial respiratory chain supercomplexes: from structure to function. Int J Mol Sci. (2022) 23(22):13880. doi: 10.3390/ijms232213880
28. Fernández-Vizarra E, Ugalde C. Cooperative assembly of the mitochondrial respiratory chain. Trends Biochem Sci. (2022) 47:999–1008. doi: 10.1016/j.tibs.2022.07.005
29. Addabbo F, Montagnani M, Goligorsky MS. Mitochondria and reactive oxygen species. Hypertension. (2009) 53:885–92. doi: 10.1161/HYPERTENSIONAHA.109.130054
30. Eftekharpour E, Fernyhough P. Oxidative stress and mitochondrial dysfunction associated with peripheral neuropathy in type 1 diabetes. Antioxid Redox Signal. (2022) 37:578–96. doi: 10.1089/ars.2021.0152
31. Imasawa T, Obre E, Bellance N, Lavie J, Imasawa T, Rigothier C, et al. High glucose repatterns human podocyte energy metabolism during differentiation and diabetic nephropathy. FASEB J. (2017) 31:294–307. doi: 10.1096/fj.201600293r
32. Chen Y, Yang Y, Liu Z, He L. Adiponectin promotes repair of renal tubular epithelial cells by regulating mitochondrial biogenesis and function. Metabolism. (2022) 128:154959. doi: 10.1016/j.metabol.2021.154959
33. Turton N, Cufflin N, Dewsbury M, Fitzpatrick O, Islam R, Watler LL, et al. The biochemical assessment of mitochondrial respiratory chain disorders. Int J Mol Sci. (2022) 23(13):7487. doi: 10.3390/ijms23137487
34. Saxena S, Mathur A, Kakkar P. Critical role of mitochondrial dysfunction and impaired mitophagy in diabetic nephropathy. J Cell Physiol. (2019) 234:19223–36. doi: 10.1002/jcp.28712
35. Rovira-Llopis S, Bañuls C, Diaz-Morales N, Hernandez-Mijares A, Rocha M, Victor VM. Mitochondrial dynamics in type 2 diabetes: Pathophysiological implications. Redox Biol. (2017) 11:637–45. doi: 10.1016/j.redox.2017.01.013
36. Chen W, Zhao H, Li Y. Mitochondrial dynamics in health and disease: mechanisms and potential targets. Signal Transduct Target Ther. (2023) 8:333. doi: 10.1038/s41392-023-01547-9
37. Kabra UD, Jastroch M. Mitochondrial dynamics and insulin secretion. Int J Mol Sci. (2023) 24(18):13782. doi: 10.3390/ijms241813782
38. Zacharioudakis E, Gavathiotis E. Mitochondrial dynamics proteins as emerging drug targets. Trends Pharmacol Sci. (2023) 44:112–27. doi: 10.1016/j.tips.2022.11.004
39. Hua ZHQ. Mitochondrial dynamic in the pathogenesis of diabetic complications. J Army Med Univ. (2022) 44(01):64–8. doi: 10.16016/j.1000-5404.202111211
40. Liu S, Li X, Wen R, Chen L, Yang Q, Song S, et al. Increased thromboxane/prostaglandin receptors contribute to high glucose-induced podocyte injury and mitochondrial fission through ROCK1-Drp1 signaling. Int J Biochem Cell Biol. (2022) 151:106281. doi: 10.1016/j.biocel.2022.106281
41. Yuan D, Li H, Dai W, Zhou X, Zhou W, He L. IGF2BP3-stabilized CAMK1 regulates the mitochondrial dynamics of renal tubule to alleviate diabetic nephropathy. Biochim Biophys Acta Mol Basis Dis. (2024) 1870:167022. doi: 10.1016/j.bbadis.2024.167022
42. Shen L, Zhang Q, Tu S, Qin W. SIRT3 mediates mitofusin 2 ubiquitination and degradation to suppress ischemia reperfusion-induced acute kidney injury. Exp Cell Res. (2021) 408:112861. doi: 10.1016/j.yexcr.2021.112861
43. Lu Y, Li Z, Zhang S, Zhang T, Liu Y, Zhang L. Cellular mitophagy: Mechanism, roles in diseases and small molecule pharmacological regulation. Theranostics. (2023) 13:736–66. doi: 10.7150/thno.79876
44. Picca A, Faitg J, Auwerx J, Ferrucci L, D'Amico D. Mitophagy in human health, ageing and disease. Nat Metab. (2023) 5:2047–61. doi: 10.1038/s42255-023-00930-8
45. Li J, Yang D, Li Z, Zhao M, Wang D, Sun Z, et al. PINK1/Parkin-mediated mitophagy in neurodegenerative diseases. Ageing Res Rev. (2023) 84:101817. doi: 10.1016/j.arr.2022.101817
46. Wang S, Long H, Hou L, Feng B, Ma Z, Wu Y, et al. The mitophagy pathway and its implications in human diseases. Signal Transduct Target Ther. (2023) 8:304. doi: 10.1038/s41392-023-01503-7
47. Zimmermann A, Madeo F, Diwan A, Sadoshima J, Sedej S, Kroemer G, et al. Metabolic control of mitophagy. Eur J Clin Invest. (2024) 54:e14138. doi: 10.1111/eci.14138
48. Strappazzon F, Nazio F, Corrado M, Cianfanelli V, Romagnoli A, Fimia GM, et al. AMBRA1 is able to induce mitophagy via LC3 binding, regardless of PARKIN and p62/SQSTM1. Cell Death Differ. (2015) 22:419–32. doi: 10.1038/cdd.2014.139
49. Bhatia D, Choi ME. Autophagy and mitophagy: physiological implications in kidney inflammation and diseases. Am J Physiol Renal Physiol. (2023) 325:F1–f21. doi: 10.1152/ajprenal.00012.2023
50. Yang L, Xu L, Hao X, Song Z, Zhang X, Liu P, et al. An aldose reductase inhibitor, WJ-39, ameliorates renal tubular injury in diabetic nephropathy by activating PINK1/Parkin signaling. Eur J Pharmacol. (2024) 967:176376. doi: 10.1016/j.ejphar.2024.176376
51. Yu L, Wang Y, Guo YH, Wang L, Yang Z, Zhai ZH, et al. HIF-1α Alleviates high-glucose-induced renal tubular cell injury by promoting parkin/PINK1-mediated mitophagy. Front Med (Lausanne). (2021) 8:803874. doi: 10.3389/fmed.2021.803874
52. Ma F, Li H, Huo H, Han Q, Liao J, Zhang H, et al. N-acetyl-L-cysteine alleviates FUNDC1-mediated mitophagy by regulating mitochondrial dynamics in type 1 diabetic nephropathy canine. Life Sci. (2023) 313:121278. doi: 10.1016/j.lfs.2022.121278
53. Liu P, Zhu W, Wang Y, Ma G, Zhao H, Li P. Chinese herbal medicine and its active compounds in attenuating renal injury via regulating autophagy in diabetic kidney disease. Front Endocrinol (Lausanne). (2023) 14:1142805. doi: 10.3389/fendo.2023.1142805
54. Srivastava A, Tomar B, Sharma D, Rath SK. Mitochondrial dysfunction and oxidative stress: Role in chronic kidney disease. Life Sci. (2023) 319:121432. doi: 10.1016/j.lfs.2023.121432
55. Zhang Z, Huang Q, Zhao D, Lian F, Li X, Qi W. The impact of oxidative stress-induced mitochondrial dysfunction on diabetic microvascular complications. Front Endocrinol (Lausanne). (2023) 14:1112363. doi: 10.3389/fendo.2023.1112363
56. Singh A, Kukreti R, Saso L, Kukreti S. Mechanistic insight into oxidative stress-triggered signaling pathways and type 2 diabetes. Molecules. (2022) 27(3):950. doi: 10.3390/molecules27030950
57. Jin Q, Liu T, Qiao Y, Liu D, Yang L, Mao H, et al. Oxidative stress and inflammation in diabetic nephropathy: role of polyphenols. Front Immunol. (2023) 14:1185317. doi: 10.3389/fimmu.2023.1185317
58. Wongmekiat O, Lailerd N, Kobroob A, Peerapanyasut W. Protective effects of purple rice husk against diabetic nephropathy by modulating PGC-1α/SIRT3/SOD2 signaling and maintaining mitochondrial redox equilibrium in rats. Biomolecules. (2021) 11(18):1124. doi: 10.3390/biom11081224
59. Liang Y, Liu H, Fang Y, Lin P, Lu Z, Zhang P, et al. Salvianolate ameliorates oxidative stress and podocyte injury through modulation of NOX4 activity in db/db mice. J Cell Mol Med. (2021) 25:1012–23. doi: 10.1111/jcmm.16165
60. Liu X, Lu J, Liu S, Huang D, Chen M, Xiong G, et al. Huangqi-Danshen decoction alleviates diabetic nephropathy in db/db mice by inhibiting PINK1/Parkin-mediated mitophagy. Am J Transl Res. (2020) 12:989–98.
61. Qiyan Z, Zhang X, Guo J, Wang Y, Jiang Y, Li S, et al. JinChan YiShen TongLuo Formula ameliorate mitochondrial dysfunction and apoptosis in diabetic nephropathy through the HIF-1α-PINK1-Parkin pathway. J Ethnopharmacol. (2024) 328:117863. doi: 10.1016/j.jep.2024.117863
62. Li Z, Deng W, Cao A, Zang Y, Wang Y, Wang H, et al. Huangqi decoction inhibits hyperglycemia-induced podocyte apoptosis by down-regulated Nox4/p53/Bax signaling in vitro and in vivo. Am J Transl Res. (2019) 11:3195–212.
63. Jin H-c, Qiang J-w, Zhang G-w, Liang S-r, Guo D-z. Danggui buxuetang alleviates oxidative stress and inflammation in diabetic kidney disease rats by improving mitochondrial dysfunction of podocytes. Chin J Exp Traditional Med Formulae. (2022) 28(03):31–40. doi: 10.13422/j.cnki.syfjx.20212442
64. F.L.Y.Q.F.Z.e Effect of xiaoyu xiezhuo drink on podocyte apoptosis and expression of HIF1α in diabetic kidney disease mice. J Zhejiang Chin Med Univ. (2024) 48(03):247–54. doi: 10.16466/j.issn1005-5509.2024.03.001
65. Li XJG, Wang Q, Lei G, Gu H, Chen L. Protective effect of Tongluo Yi shen Formula on mitochondrial dysfunction in rats with diabetic nephropathy. Chin Traditional Patent Med. (2021) 43(02):475–80. doi: 10.3969/j.issn.10011528.2021.02.035
66. Wang GL, Jiang BH, Rue EA, Semenza GL. Hypoxia-inducible factor 1 is a basic-helix-loop-helix-PAS heterodimer regulated by cellular O2 tension. Proc Natl Acad Sci USA. (1995) 92:5510–4. doi: 10.1073/pnas.92.12.5510
67. Zhang YZB, Chi Y, Liu S, Wang H, Wang Y. Effects of HuangQi decoction on stage III diabetic nephropathy patients with deficiency of qi and yin renal function and glucose-lipid metabolism Lishizhen Medicine and Materia Medica Research. (2022) 33(10):2435–37. doi: 10.3969/j.issn
68. C.Y.L.S.W.H.e. Effect of huangqi decoction on proteinuria and Related inflammatory factors expression of patients with diabetic kidney disease III. Chin J Integrated Traditional Western Nephrol. (2020) 21(04):305–8.
69. Eid AA, Ford BM, Block K, Kasinath BS, Gorin Y, Ghosh-Choudhury G, et al. AMP-activated protein kinase (AMPK) negatively regulates Nox4-dependent activation of p53 and epithelial cell apoptosis in diabetes. J Biol Chem. (2010) 285:37503–12. doi: 10.1074/jbc.M110.136796
70. Fontecha-Barriuso M, Martin-Sanchez D, Martinez-Moreno JM, Monsalve M, Ramos AM, Sanchez-Niño MD, et al. The role of PGC-1α and mitochondrial biogenesis in kidney diseases. Biomolecules. (2020) 10(2):347. doi: 10.3390/biom10020347
71. Lee G, Uddin MJ, Kim Y, Ko M, Yu I, Ha H. PGC-1α, a potential therapeutic target against kidney aging. Aging Cell. (2019) 18:e12994. doi: 10.1111/acel.12994
72. Lu Wei LX. Clinical efficacy of Danggui Buxue decoction in the treatment of diabetic nephropathy and its effect on the level of inflammatory factors. J Hebei Traditional Chin Med Pharmacol. (2018) 33(01):22-5. doi: 10.16370/j.cnki.13-1214/r.2018.01.007
73. Ke P-x, L-zH, Zhang P-p. Effect of Xiaoyuxiezhuoyin on serum leptin in diabetic patients with renal failure. China J Modern Med. (2018) 28(24):53–6.
74. Jiang N, Zhao H, Han Y, Li L, Xiong S, Zeng L, et al. HIF-1α ameliorates tubular injury in diabetic nephropathy via HO-1-mediated control of mitochondrial dynamics. Cell Prolif. (2020) 53:e12909. doi: 10.1111/cpr.12909
75. Halling JF, Pilegaard H. PGC-1α-mediated regulation of mitochondrial function and physiological implications. Appl Physiol Nutr Metab. (2020) 45:927–36. doi: 10.1139/apnm-2020-0005
76. Rong L. Clinical study on the treatment of diabetic nephropathy by TongLuoYiShen formula combined with candesartan. New Chin Med. (2015) 47(03):89–92. doi: 10.13457/j.cnki.jncm.2015.03.043
77. Zhuang W, Liu SL, Xi SY, Feng YN, Wang K, Abduwali T, et al. Traditional Chinese medicine decoctions and Chinese patent medicines for the treatment of depression: Efficacies and mechanisms. J Ethnopharmacol. (2023) 307:116272. doi: 10.1016/j.jep.2023.116272
78. Zhang L, Yang Z, Zhao Y, Yang X, Meng X, Liu J, et al. Renoprotective effects of Gushen Jiedu capsule on diabetic nephropathy in rats. Sci Rep. (2020) 10:2040. doi: 10.1038/s41598-020-58781-2
79. Wang X, Zhao L, Ajay AK, Jiao B, Zhang X, Wang C, et al. QiDiTangShen granules activate renal nutrient-sensing associated autophagy in db/db mice. Front Physiol. (2019) 10:1224. doi: 10.3389/fphys.2019.01224
80. Liu Y, Liu W, Zhang Z, Hu Y, Zhang X, Sun Y, et al. Yishen capsule promotes podocyte autophagy through regulating SIRT1/NF-κB signaling pathway to improve diabetic nephropathy. Ren Fail. (2021) 43:128–40. doi: 10.1080/0886022X.2020.1869043
81. Li H, Wang Y, Su X, Wang Q, Zhang S, Sun W, et al. San-huang-yi-shen capsule ameliorates diabetic kidney disease through inducing PINK1/parkin-mediated mitophagy and inhibiting the activation of NLRP3 signaling pathway. J Diabetes Res. (2022) 2022:2640209. doi: 10.1155/2022/2640209
82. Sun M, Bu W, Li Y, Zhu J, Zhao J, Zhang P, et al. Danzhi Jiangtang Capsule ameliorates kidney injury via inhibition of the JAK-STAT signaling pathway and increased antioxidant capacity in STZ-induced diabetic nephropathy rats. Biosci Trends. (2019) 12:595–604. doi: 10.5582/bst.2018.01255
83. Chen Y, Zhang L, Liu S, Yao B, Zhang H, Liang S, et al. Sam68 mediates high glucose−induced podocyte apoptosis through modulation of Bax/Bcl−2. Mol Med Rep. (2019) 20:3728–34. doi: 10.3892/mmr
84. Qian X, Tan J, Liu L, Chen S, You N, Yong H, et al. MicroRNA-134-5p promotes high glucose-induced podocyte apoptosis by targeting bcl-2. Am J Transl Res. (2018) 10:989–97.
85. Zhang Z, Hu Y, Liu W, Zhang X, Wang R, Li H, et al. Yishen capsule alleviated symptoms of diabetic nephropathy via NOD-like receptor signaling pathway. Diabetes Metab Syndr Obes. (2022) 15:2183–95. doi: 10.2147/DMSO.S368867
86. Song H-l, Li S-q, Gan Z-q, Su X-h, Zhang S-f, Wang Y-s. Effects of San-Huang-Yi-Shen capsule on renal function and renal fibrosis indexes in patients with early diabetic nephropathy. Modern J Integrated Traditional Chin Western Med. (2018) 27(23):25512553. doi: 10.3969/j.issn.1005-8982.2018.24.010
87. Han YC, Tang SQ, Liu YT, Li AM, Zhan M, Yang M, et al. AMPK agonist alleviate renal tubulointerstitial fibrosis via activating mitophagy in high fat and streptozotocin induced diabetic mice. Cell Death Dis. (2021) 12:925. doi: 10.1038/s41419-021-04184-8
88. Jena AB, Samal RR, Bhol NK, Duttaroy AK. Cellular Red-Ox system in health and disease: The latest update. BioMed Pharmacother. (2023) 162:114606. doi: 10.1016/j.biopha.2023.114606
89. Toto A, Wild P, Graille M, Turcu V, Crézé C, Hemmendinger M, et al. Urinary malondialdehyde (MDA) concentrations in the general population-A systematic literature review and meta-analysis. Toxics. (2022) 10(4):160. doi: 10.3390/toxics10040160
90. Liu Y, Wang S, Jin G, Gao K, Wang S, Zhang X, et al. Network pharmacology-based study on the mechanism of ShenKang injection in diabetic kidney disease through Keap1/Nrf2/Ho-1 signaling pathway. Phytomedicine. (2023) 118:154915. doi: 10.1016/j.phymed.2023.154915
91. Liu W, Liang L, Zhang Q, Li Y, Yan S, Tang T, et al. Effects of andrographolide on renal tubulointersticial injury and fibrosis. Evidence of its mechanism of action. Phytomedicine. (2021) 91:153650. doi: 10.1016/j.phymed.2021.153650
92. Liu H, Chen W, Lu P, Ma Y, Liang X, Liu Y. Ginsenoside Rg1 attenuates the inflammation and oxidative stress induced by diabetic nephropathy through regulating the PI3K/AKT/FOXO3 pathway. Ann Transl Med. (2021) 9:1789. doi: 10.21037/atm
93. Su J, Gao C, Xie L, Fan Y, Shen Y, Huang Q, et al. Astragaloside II ameliorated podocyte injury and mitochondrial dysfunction in streptozotocin-induced diabetic rats. Front Pharmacol. (2021) 12:638422. doi: 10.3389/fphar.2021.638422
94. Zhong Y, Wang L, Jin R, Liu J, Luo R, Zhang Y, et al. Diosgenin inhibits ROS generation by modulating NOX4 and mitochondrial respiratory chain and suppresses apoptosis in diabetic nephropathy. Nutrients. (2023) 15(9):2164. doi: 10.3390/nu15092164
95. Huang Q, Chen H, Yin K, Shen Y, Lin K, Guo X, et al. Formononetin attenuates renal tubular injury and mitochondrial damage in diabetic nephropathy partly via regulating sirt1/PGC-1α Pathway. Front Pharmacol. (2022) 13:901234. doi: 10.3389/fphar.2022.901234
96. Kong ZL, Che K, Hu JX, Chen Y, Wang YY, Wang X, et al. Orientin protects podocytes from high glucose induced apoptosis through mitophagy. Chem Biodivers. (2020) 17:e1900647. doi: 10.1002/cbdv.201900647
97. Park J, Seo E, Jun HS. Bavachin alleviates diabetic nephropathy in db/db mice by inhibition of oxidative stress and improvement of mitochondria function. BioMed Pharmacother. (2023) 161:114479. doi: 10.1016/j.biopha.2023.114479
98. Wang X, Li Q, Sui B, Xu M, Pu Z, Qiu T. Schisandrin A from Schisandra chinensis Attenuates Ferroptosis and NLRP3 Inflammasome-Mediated Pyroptosis in Diabetic Nephropathy through Mitochondrial Damage by AdipoR1 Ubiquitination. Oxid Med Cell Longev 2022. (2022) p:5411462. doi: 10.1155/2022/5411462
99. Huang Q, Zhang Y, Jiang Y, Huang L, Liu Q, Ouyang D. Eucommia lignans alleviate the progression of diabetic nephropathy through mediating the AR/Nrf2/HO-1/AMPK axis in vivo and in vitro. Chin J Nat Med. (2023) 21:516–26. doi: 10.1016/S1875-5364(23)60427-3
100. Yang WJ, Li YR, Gao H, Wu XY, Wang XL, Wang XN, et al. Protective effect of the ethanol extract from Ligusticum chuanxiong rhizome against streptozotocin-induced diabetic nephropathy in mice. J Ethnopharmacol. (2018) 227:166–75. doi: 10.1016/j.jep.2018.08.037
101. Al Shahrani M, Chandramoorthy HC, Alshahrani M, Abohassan M, Eid RA, Ravichandran K, et al. Cassia auriculata leaf extract ameliorates diabetic nephropathy by attenuating autophagic necroptosis via RIP-1/RIP-3-p-p38MAPK signaling. J Food Biochem. (2021) 45:e13810. doi: 10.1111/jfbc.13810
102. Dai Y, Chen SR, Chai L, Zhao J, Wang Y, Wang Y. Overview of pharmacological activities of Andrographis paniculata and its major compound andrographolide. Crit Rev Food Sci Nutr. (2019) 59:S17–s29. doi: 10.1080/10408398.2018.1501657
103. Zeng B, Wei A, Zhou Q, Yuan M, Lei K, Liu Y, et al. Andrographolide: A review of its pharmacology, pharmacokinetics, toxicity and clinical trials and pharmaceutical researches. Phytother Res. (2022) 36:336–64. doi: 10.1002/ptr.7324
104. Wu JJ, Yang Y, Wan Y, Xia J, Xu JF, Zhang L, et al. New insights into the role and mechanisms of ginsenoside Rg1 in the management of Alzheimer's disease. BioMed Pharmacother. (2022) 152:113207. doi: 10.1016/j.biopha.2022.113207
105. Wang N, Yang J, Chen R, Liu Y, Liu S, Pan Y, et al. Ginsenoside Rg1 ameliorates Alzheimer's disease pathology via restoring mitophagy. J Ginseng Res. (2023) 47:448–57. doi: 10.1016/j.jgr.2022.12.001
106. Ji P, Shi Q, Liu Y, Han M, Su Y, Sun R, et al. Ginsenoside Rg1 treatment alleviates renal fibrosis by inhibiting the NOX4-MAPK pathway in T2DM mice. Ren Fail. (2023) 45:2197075. doi: 10.1080/0886022X.2023.2197075
107. Parama D, Boruah M, Yachna K, Rana V, Banik K, Harsha C, et al. Diosgenin, a steroidal saponin, and its analogs: Effective therapies against different chronic diseases. Life Sci. (2020) 260:118182. doi: 10.1016/j.lfs.2020.118182
108. Wang D, Li J, Luo G, Zhou J, Wang N, Wang S, et al. Nox4 as a novel therapeutic target for diabetic vascular complications. Redox Biol. (2023) 64:102781. doi: 10.1016/j.redox.2023.102781
109. Geiszt M, Kopp JB, Várnai P, Leto TL. Identification of renox, an NAD(P)H oxidase in kidney. Proc Natl Acad Sci USA. (2000) 97:8010–4. doi: 10.1073/pnas.130135897
110. Fang Y, Ye J, Zhao B, Sun J, Gu N, Chen X, et al. Formononetin ameliorates oxaliplatin-induced peripheral neuropathy via the KEAP1-NRF2-GSTP1 axis. Redox Biol. (2020) 36:101677. doi: 10.1016/j.redox.2020.101677
111. Xiao Q, Zhao Y, Ma L, Piao R. Orientin reverses acetaminophen-induced acute liver failure by inhibiting oxidative stress and mitochondrial dysfunction. J Pharmacol Sci. (2022) 149:11–9. doi: 10.1016/j.jphs.2022.01.012
112. Li L, Zhu Y, Huang YG, Hou DZ, Ahmed Zaki MS, Sideeg AM, et al. Therapeutic properties, biological effects, antiliver cancer, and anticolon cancer effects of some natural compounds: A biochemical approach. J Biochem Mol Toxicol. (2024) 38:e23573. doi: 10.1002/jbt.23573
113. Hung YL, Wang SC, Suzuki K, Fang SH, Chen CS, Cheng WC, et al. Bavachin attenuates LPS-induced inflammatory response and inhibits the activation of NLRP3 inflammasome in macrophages. Phytomedicine. (2019) 59:152785. doi: 10.1016/j.phymed.2018.12.008
114. He HQ, Law BYK, Zhang N, Qiu CL, Qu YQ, Wu AG, et al. Bavachin Protects Human Aortic Smooth Muscle Cells Against β-Glycerophosphate-Mediated Vascular Calcification and Apoptosis via Activation of mTOR-Dependent Autophagy and Suppression of β-Catenin Signaling. Front Pharmacol. (2019) 10:1427. doi: 10.3389/fphar.2019.01427
115. Lee JY, Lim W, Song G. Bavachin suppresses human placental choriocarcinoma cells by targeting electron transport chain complexes and mitochondrial dysfunction. Free Radic Biol Med. (2020) 156:26–35. doi: 10.1016/j.freeradbiomed.2020.05.022
116. Ni YH, Deng HF, Zhou L, Huang CS, Wang NN, Yue LX, et al. Ginsenoside rb1 ameliorated bavachin-induced renal fibrosis via suppressing bip/eIF2α/CHOP signaling-mediated EMT. Front Pharmacol. (2022) 13:872474. doi: 10.3389/fphar.2022.872474
117. Pu H, Qian Q, Wang F, Gong M, Ge X. Schizandrin A induces the apoptosis and suppresses the proliferation, invasion and migration of gastric cancer cells by activating endoplasmic reticulum stress. Mol Med Rep. (2021) 24. doi: 10.3892/mmr
118. Zong W, Gouda M, Cai E, Wang R, Xu W, Wu Y, et al. The antioxidant phytochemical schisandrin A promotes neural cell proliferation and differentiation after ischemic brain injury. Molecules. (2021) 26(24):7466. doi: 10.3390/molecules26247466
119. Liu B, Li CP, Wang WQ, Song SG, Liu XM. Lignans extracted from eucommia ulmoides oliv. Protects against AGEs-induced retinal endothelial cell injury. Cell Physiol Biochem. (2016) 39:2044–54. doi: 10.1159/000447900
120. Thakur S, Gupta SK, Ali V, Singh P, Verma M. Aldose Reductase: a cause and a potential target for the treatment of diabetic complications. Arch Pharm Res. (2021) 44:655–67. doi: 10.1007/s12272-021-01343-5
121. Zhang LX, Li CX, Kakar MU, Khan MS, Wu PF, Amir RM, et al. Resveratrol (RV): A pharmacological review and call for further research. BioMed Pharmacother. (2021) 143:112164. doi: 10.1016/j.biopha.2021.112164
122. Huang DD, Shi G, Jiang Y, Yao C, Zhu C. A review on the potential of Resveratrol in prevention and therapy of diabetes and diabetic complications. BioMed Pharmacother. (2020) 125:109767. doi: 10.1016/j.biopha.2019.109767
123. Wang G, Chen L, Pan X, Chen J, Wang L, Wang W, et al. The effect of resveratrol on beta amyloid-induced memory impairment involves inhibition of phosphodiesterase-4 related signaling. Oncotarget. (2016) 7:17380–92. doi: 10.18632/oncotarget.v7i14
124. Cao Y, Sun W, Liu C, Zhou Z, Deng Z, Zhang M, et al. Resveratrol ameliorates diabetic encephalopathy through PDE4D/PKA/Drp1 signaling. Brain Res Bull. (2023) 203:110763. doi: 10.1016/j.brainresbull.2023.110763
125. Hashemi M, Zandieh MA, Ziaolhagh S, Mojtabavi S, Sadi FH, Koohpar ZK, et al. Nrf2 signaling in diabetic nephropathy, cardiomyopathy and neuropathy: Therapeutic targeting, challenges and future prospective. Biochim Biophys Acta Mol Basis Dis. (2023) 1869:166714. doi: 10.1016/j.bbadis.2023.166714
126. Han J, Pang X, Zhang Y, Peng Z, Shi X, Xing Y. Hirudin protects against kidney damage in streptozotocin-induced diabetic nephropathy rats by inhibiting inflammation via P38 MAPK/NF-κB pathway. Drug Des Devel Ther. (2020) 14:3223–34. doi: 10.2147/DDDT.S257613
127. Tang G, Li S, Zhang C, Chen H, Wang N, Feng Y. Clinical efficacies, underlying mechanisms and molecular targets of Chinese medicines for diabetic nephropathy treatment and management. Acta Pharm Sin B. (2021) 11:2749–67. doi: 10.1016/j.apsb.2020.12.020
128. Selby NM, Taal MW. An updated overview of diabetic nephropathy: Diagnosis, prognosis, treatment goals and latest guidelines. Diabetes Obes Metab. (2020) 22 Suppl 1:3–15. doi: 10.1111/dom.14007
129. Li J, Li L, Zhang Z, Chen P, Shu H, Yang C, et al. Ferroptosis: an important player in the inflammatory response in diabetic nephropathy. Front Immunol. (2023) 14:1294317. doi: 10.3389/fimmu.2023.1294317
130. Zhao H, Yang CE, Liu T, Zhang MX, Niu Y, Wang M, et al. The roles of gut microbiota and its metabolites in diabetic nephropathy. Front Microbiol. (2023) 14:1207132. doi: 10.3389/fmicb.2023.1207132
131. Yang M, Luo S, Yang J, Chen W, He L, Liu D, et al. Myokines: Novel therapeutic targets for diabetic nephropathy. Front Endocrinol (Lausanne). (2022) 13:1014581. doi: 10.3389/fendo.2022.1014581
132. Chen J, Liu Q, He J, Li Y. Immune responses in diabetic nephropathy: Pathogenic mechanisms and therapeutic target. Front Immunol. (2022) 13:958790. doi: 10.3389/fimmu.2022.958790
133. Paul P, Chacko L, Dua TK, Chakraborty P, Paul U, Phulchand VV, et al. Nanomedicines for the management of diabetic nephropathy: present progress and prospects. Front Endocrinol (Lausanne). (2023) 14:1236686. doi: 10.3389/fendo.2023.1236686
Glossary
Keywords: diabetic nephropathy, mitochondrial dysfunction, traditional Chinese medicine, oxidative stress, mitophagy
Citation: Zhao D-m, Zhong R, Wang X-t and Yan Z-h (2024) Mitochondrial dysfunction in diabetic nephropathy: insights and therapeutic avenues from traditional Chinese medicine. Front. Endocrinol. 15:1429420. doi: 10.3389/fendo.2024.1429420
Received: 08 May 2024; Accepted: 08 July 2024;
Published: 23 July 2024.
Edited by:
Yongsheng Chen, Jinan University, ChinaReviewed by:
Junren Chen, Chengdu University of Traditional Chinese Medicine, ChinaGo Kanzaki, Jikei University School of Medicine, Japan
Copyright © 2024 Zhao, Zhong, Wang and Yan. This is an open-access article distributed under the terms of the Creative Commons Attribution License (CC BY). The use, distribution or reproduction in other forums is permitted, provided the original author(s) and the copyright owner(s) are credited and that the original publication in this journal is cited, in accordance with accepted academic practice. No use, distribution or reproduction is permitted which does not comply with these terms.
*Correspondence: Zhong-hong Yan, OTg2MDQ5MTgzQHFxLmNvbQ==